DOI:
10.1039/D4FO03948C
(Paper)
Food Funct., 2024,
15, 10663-10678
Impact of complementary feeding on infant gut microbiome, metabolites and early development†
Received
16th August 2024
, Accepted 13th September 2024
First published on 20th September 2024
Abstract
Introducing complementary foods is critical for promoting infant health and development. During the weaning period, the dietary patterns provide essential nutrients and facilitate the development of a diverse gut microbiome, which plays significant roles in the regulation of immune, metabolic, and neurological functions. This study enrolled 200 families to assess the impact of complementary feeding on infant growth and health outcomes. Data included detailed records of feeding practices, infant growth measurements, health assessments, and fecal samples and breast milk collected between weeks 12 and 32 postpartum. The gut microbiome was analyzed using 16S rRNA sequencing, while metabolites such as human milk oligosaccharides (HMOs), monosaccharides, and short-chain fatty acids (SCFAs) were measured using chromatography-mass spectrometry. Results revealed a high prevalence of breastfeeding, with complementary food introduced at around 16 weeks. Significant alterations in the infant gut microbiome were observed, particularly in the genera Lactobacillus, Akkermansia, and Staphylococcus. Additionally, the levels of HMOs, monosaccharides, and SCFAs were found to be influenced by the introduction of complementary foods. Significant correlations emerged between complementary feeding practices, gut microbiome diversity, specific bacterial genera (e.g., Streptococcus, Lactobacillus, Bifidobacterium, and Clostridioides), and key metabolites (such as lacto-N-tetraose, lacto-N-neotetraose, mannose, and butyric acid). This study offers valuable insights into the complex interactions between complementary feeding, gut microbiome development, and metabolite profiles during early infant growth. Future research with larger cohorts and targeted dietary interventions is recommended to further elucidate the underlying mechanisms.
1. Introduction
The transition from an exclusive breast milk diet to the introduction of complementary foods, commonly referred to as weaning, is typically recommended at approximately six months postpartum.1 For preterm infants, this transition is often expedited to between 13 and 17 weeks, accounting for their organ immaturity and the need for nutritional support that mirrors in utero growth patterns.2,3 This shift from breast milk to complementary feeding is primarily driven by the infant's increasing nutritional demands, which breast milk or formula alone may not fully address.4,5 Complementary foods provide a diverse array of nutrients, including minerals,6–8 vitamins,9–11 unsaturated fatty acids12–14 and others,15,16 which are crucial not only for meeting the infant's metabolic demands but also for preventing health issues.9,12,13 For instance, infants at risk of vitamin D deficiency may experience bone and musculoskeletal issues, leading to conditions such as rickets in children and osteoporosis, cardiovascular disease and diabetes in adults.17–19 A diet lacking sufficient iron at around six months of age can potentially lead to iron deficiency, but introducing iron-rich foods at this stage helps replenish iron stores.8,20 Emerging research suggests that complementary feeding enriches the diversity of the infant gut microbiome and promotes the early maturation of the immune system.21,22 Evidence has shown that the introduction of a variety of complementary foods alters the composition of gut microbes such as Lachnospiraceae, Bifidobacteriaceae and Akkermansia, thereby significantly impacting infant development.23,24 Specific bacteria such as Bacteroides and Bifidobacterium play a role in teaching the immune system to distinguish between harmful and harmless antigens, reducing the risk of autoimmune diseases and allergies as they grow older.25,26 Research also suggests that complementary feeding not only enriches the diversity of the infant gut microbiome but also accelerates the maturation of T-regulatory cells, which are essential for maintaining immune tolerance.27 Moreover, complementary foods alter the composition of gut microbes such as Lachnospiraceae, Bifidobacteriaceae, and Akkermansia, which further impact infant development in profound ways.28,29 These findings underscore linkages between complementary feeding, dietary nutrients and the infant gut microbiome and how they influence long-term developmental outcomes in infants.30,31
In this study, we recruited 120 families with full-term infants at 12 weeks of age, collecting infant fecal and paired breast milk samples, along with data on complementary feeding, growth parameters and health outcomes. To fully understand how complementary feeding impacts early infant development through the gut microbiome and metabolites, both fecal and milk samples were analyzed and quantified by either liquid/gas chromatography coupled with mass spectrometry or 16s rRNA sequencing. Integrative analysis was conducted to uncover how infant growth and health transformed with the introduction of complementary food, with a specific focus on the interplay between the gut microbiota and metabolites.
2. Materials and methods
2.1 Ethics approval and criteria for participants
The study procedures are in accordance with the ethical standards of the Institutional Review Board of the Peking University Biomedicine. Written informed consent was obtained from all participants. The inclusion criteria for participants were as follows: (1) being over 18 years old, (2) breastfeeding a full-term infant, and (3) residing in the same city for more than a year. The exclusion criteria included: (1) gestational age less than 37 weeks, (2) incomplete follow-up, and (3) weaning before the infant reached 6 months of age. Additional exclusion criteria were pre-existing or ongoing medical conditions (e.g., diabetes mellitus and hypertension), use of medications or antibiotics during pregnancy affecting fetal growth, and habits such as smoking or alcohol consumption during pregnancy.
2.2 Study design
The study design is summarized in Fig. 1. In detail, fecal samples from infants were collected biweekly from weeks 12 to 32 postpartum, paired with mothers’ breast milk samples. Fecal and breast milk metabolites, along with the fecal microbiome, were measured, and the relationship among the metabolites, microbiome and infant growth parameters and health outcomes was analyzed.
 |
| Fig. 1 The flow chart of the experimental design. | |
2.3 Metabolite quantification
HMOs from both infant feces and mothers’ breast milk were extracted based on previous studies.32,33 Briefly, the samples were pretreated with ethanol to remove protein. The HMOs were then identified using high-performance liquid chromatography (HPLC) coupled with mass spectrometry, equipped with electrospray ionization. Quantification of HMOs was performed relatively using lacto-N-tetraose (LNT) as an internal reference due to the lack of commercially available individual HMO standards. Monosaccharides were identified using an HPLC system with an Agilent C18 column, coupled with mass spectrometry. Standard curves for monosaccharides with R2 values exceeding 0.95 were generated for serially diluted pure compounds. SCFAs were measured based on previous studies with modifications34–36 using gas chromatography-mass spectrometry with an internal standard for quantification.
2.4 Gut microbiome identification
To determine the shift of the gut microbiota, bacterial DNA was extracted using the QIAGEN PowerSoil DNA Isolation Kit following the manufacturer's instructions. The DNA concentration of each sample was detected using a NanoDrop spectrophotometer (Thermo Fisher Scientific, Waltham, MA, USA). The V4 region of the 16S rRNA gene was amplified by universal barcoded primers (forward primer 515F 5′-GTGCCAGCMGCCGCGGTAA-3′ and reverse primer 806R 5′-GGACTACNVGGGTWTCTAAT-3′).37 Then, indexed amplicons were purified with AMPure XP beads (Beckman Coulter Genomics, MA, USA), quantified and pooled in equimolar concentrations, and then sequenced on an Illumina MiSeq platform. Microbial operational taxonomic units and their taxonomic assignments were analyzed using the Quantitative Insights Into Microbial Ecology (QIIME2) bioinformatics pipeline with modifications.38–40 To compare fecal samples across time and between the groups, operational taxonomic unit-based and phylogenetic alpha-diversity metrics were calculated using rarefied datasets. UniFrac-based sample clustering was performed using principal component analysis and confirmed using statistical analysis of variance.
2.5 Statistics
The data were presented as means ± standard deviation. Multivariable analysis of variance (ANOVA) using gut diversity, microbiome, metabolites, and time points was performed with Tukey's multiple comparison test for multiple group comparisons. The Student's t-test was performed for simple comparison, with a p-value less than 0.05 considered significant.
3. Results
3.1 Characteristics of the study population
The average maternal age was 31.7 ± 4.4 years. The gender ratio of infants was nearly even, with 52% male and 48% female. It is noteworthy that a significant majority, 68% of infants, were born via vaginal delivery (VD). Data on educational background, family income, and monthly expenses of participating families were collected and analyzed. Interestingly, the average food expense for the infants during the weaning period accounted for approximately 6.3% of the family income. In contrast, these figures were tripled to roughly 18% in the United States, highlighting a significant difference in dietary and cultural habits, along with economic choice, between Chinese and Western dietary middle-class families. This disparity offers a compelling avenue for further study, both from nutritional and sociocultural perspectives. The demographics and relevant participant details are summarized in ESI Table S1.†
3.2 Complementary feeding
In this study, a significant increase was observed in the rate of complementary feeding among participating families, with a rise from 10% at week 16 to 80% by week 28, and reaching a 90% adoption at week 32. Six major types of complementary foods were identified: fruits/rice soup, cereals, leafy vegetables and beans, solid fruits, meat and egg/dairy products, and the feeding time and adding ratio are all summarized in Table 1. As is shown, the most frequently selected complementary foods were fruits and cereals. Leafy vegetables, beans, and meat were generally introduced later, around week 20. The top five complementary foods incorporated were apple, banana, rice cereal, egg yolk, and carrot. No significant correlation was found between food choices and family income, parental education background, or maternal age at birth. In addition, the overall proportion of breastfeeding decreased by only 5% from weeks 12 to 32, with just 5% of families relying solely on breastfeeding. Meanwhile, the rate of introducing complementary feeding rose to 95% and formula feeding increased to 30%. These figures differ significantly from the breastfeeding data in the United States, where the rates were 55% at three months and 42% at six months, suggesting a potential impact of breastfeeding rates on infant growth and health during the weaning period.41
Table 1 Overview of Complementary Feeding Types and Key Food Categories
Food type |
Week 16 |
Week 20 |
Week 24 |
Week 28 |
Week 32 |
Others include coconut milk, soy milk, and goat's milk.
Others include mango, pineapple, plum, apricot, etc.
Others include sausages, duck, lamb, turkey etc.
Others include egg white, cow milk, yogurt, cheese, etc.
|
Complementary food ratio (%) |
Fruits/rice soup |
7.0 |
11.0 |
24.0 |
43.1 |
53.7 |
Cereals |
1.6 |
11.0 |
24.8 |
60.2 |
71.1 |
Leafy vegetables and beans |
0.0 |
0.0 |
8.5 |
37.4 |
52.1 |
Solid fruits |
2.3 |
7.1 |
20.9 |
58.5 |
78.5 |
Meat |
0.0 |
1.6 |
2.3 |
13.8 |
30.6 |
Egg/dairy products |
1.6 |
4.7 |
10.9 |
35.0 |
53.7 |
Fruits/rice soup adding ratio (%) |
Rice soup |
1.6 |
4.7 |
14.0 |
29.3 |
35.5 |
Pure fruit juice |
0.8 |
3.2 |
7.0 |
10.6 |
19.8 |
Boiled fruit juice |
0.8 |
1.6 |
4.7 |
13.8 |
11.6 |
Vegetable juice |
0.8 |
0.1 |
2.3 |
4.1 |
7.4 |
Othersa |
2.3 |
3.2 |
3.1 |
2.4 |
4.1 |
Cereal adding ratio (%) |
Rice product |
0.8 |
7.1 |
20.2 |
43.1 |
54.6 |
Biscuits |
0.0 |
0.0 |
1.6 |
12.2 |
21.5 |
Millet |
0.8 |
1.6 |
3.1 |
10.6 |
19.0 |
Wheat pasta |
0.0 |
1.6 |
0.8 |
8.1 |
16.5 |
Regular bread |
0.0 |
0.8 |
2.3 |
3.3 |
9.1 |
Mixed cereal |
0.0 |
0.0 |
2.3 |
6.5 |
6.6 |
Mixed oatmeal |
0.0 |
0.0 |
0.8 |
1.6 |
4.1 |
Leafy vegetable and bean adding ratio (%) |
Carrot |
0.0 |
0.0 |
7.0 |
26.0 |
36.4 |
Pumpkin |
0.0 |
0.0 |
1.6 |
18.7 |
30.6 |
Mashed/baked Potatoes |
0.0 |
0.0 |
1.6 |
8.9 |
24.8 |
Spinach |
0.0 |
0.0 |
0.0 |
8.1 |
23.1 |
Sweet potatoes |
0.0 |
0.0 |
2.3 |
19.5 |
21.5 |
Broccoli |
0.0 |
0.0 |
0.8 |
7.3 |
20.7 |
Avocado |
0.0 |
0.0 |
0.8 |
4.9 |
14.1 |
Rape |
0.0 |
0.0 |
0.0 |
3.3 |
12.4 |
Corn |
0.0 |
0.0 |
0.0 |
4.9 |
11.6 |
Chinese cabbage |
0.0 |
0.0 |
0.0 |
3.3 |
11.6 |
Squash |
0.0 |
0.0 |
0.0 |
2.4 |
7.4 |
Bok choy |
0.0 |
0.0 |
0.8 |
1.6 |
6.6 |
Cauliflower |
0.0 |
0.0 |
0.0 |
0.8 |
5.8 |
Cucumber |
0.0 |
0.0 |
0.0 |
2.4 |
5.8 |
Pea |
0.0 |
0.0 |
0.8 |
2.4 |
5.8 |
Radish |
0.0 |
0.0 |
0.0 |
1.6 |
5.0 |
Tofu |
0.0 |
0.0 |
0.8 |
2.4 |
4.1 |
Solid fruit adding ratio (%) |
Apple |
1.6 |
3.9 |
15.5 |
47.2 |
72.7 |
Banana |
0.8 |
2.4 |
10.1 |
32.5 |
61.2 |
Orange |
0.0 |
0.0 |
4.7 |
4.9 |
17.4 |
Pear |
0.0 |
0.0 |
1.6 |
9.8 |
12.4 |
Strawberry |
0.0 |
0.0 |
1.6 |
4.9 |
12.4 |
Blueberry |
0.0 |
0.0 |
0.0 |
0.8 |
9.9 |
Melons |
0.8 |
1.6 |
3.1 |
3.3 |
9.9 |
Kiwi |
0.0 |
0.8 |
1.6 |
7.3 |
8.3 |
Peach |
0.0 |
0.0 |
0.0 |
4.1 |
5.8 |
Othersb |
0.0 |
0.0 |
0.8 |
1.0 |
14.9 |
Meat adding ratio (%) |
Beef |
0.0 |
0.0 |
0.0 |
2.4 |
12.4 |
Pork |
0.0 |
0.8 |
1.6 |
6.5 |
12.4 |
Fish |
0.0 |
0.8 |
0.8 |
3.3 |
11.6 |
Chicken |
0.0 |
0.0 |
0.0 |
4.9 |
9.1 |
Animal liver |
0.0 |
0.0 |
0.8 |
1.6 |
7.4 |
Shrimp |
0.0 |
0.0 |
0.0 |
0.8 |
5.8 |
Othersc |
0 |
0 |
1.6 |
2.4 |
6.6 |
Egg/dairy product adding ratio (%) |
Yolk |
0.0 |
4.7 |
10.9 |
33.3 |
47.9 |
Dairy biscuit |
0.0 |
0.0 |
3.1 |
4.9 |
10.7 |
Whole egg |
1.6 |
0.0 |
0.8 |
2.4 |
5.0 |
Othersd |
0.8 |
0.8 |
1.6 |
4.9 |
13.2 |
3.3 Metabolites
Nineteen types of HMOs, six types of monosaccharides and nine types of SCFAs were identified in infant fecal samples, while 28 types of HMOs were identified in breast milk (ESI Table S2†). The average HMO concentrations were 41
000 μg g−1 in feces and 16
000 μg mL−1 in breast milk. The prevalent fecal HMOs 2′-fucosyllactose (2′-FL), 3′-fucosyllactose (3′-FL), LNT, and lacto-N-neotetraose (LNnT) were quantified at 351, 287, 1967 and 946 μg g−1, respectively. However, no direct correlation was observed between the ratios of HMOs in the mother's breast milk and those in infant feces. The corresponding levels in breast milk were 153, 75, 2231 and 831 μg mL−1, respectively. Consistent with previous studies, it is likely that the concentration variances in HMOs result from a combination of factors, including delivery mode and complementary foods, as well as gut microbiome and metabolic activities during weaning.42 In Fig. 2a, a decreasing trend was observed for most identified HMOs, with the concentrations ranging from 1000 to 50 μg g−1. In Fig. 2b, the concentrations of monosaccharides including allose, galactose, glucose, mannose, xylose and arabinose were 0.3, 16.9, 12.7, 1.7, and 0.6 μg mg−1 feces, respectively. Most of the monosaccharides showed a decreasing trend, while mannose and xylose/arabinose remained relatively stable throughout the weaning period. In Fig. 2c, acetic acid was the most abundant SCFA in infant feces, accounting for approximately 58.1%, followed by lactic acid at 13.8% and glycolaldehyde at 11.6%. Overall, increasing levels of acetic acid, butyric acid, and propionic acid were observed, while the levels of lactic acid and hydroxyaldehyde decreased. The concentrations of these SCFAs ranged between 300 and 5000 ng mg−1.
 |
| Fig. 2 The overall changes in the gut microbiota during the weaning period. In (a), four types of HMOs were present. In (b), six types of monosaccharide are present. In (c), nine types of unsaturated fatty acids were present. | |
3.4 Gut microbiome
Major bacterial genera were identified and summarized (Fig. 3a and Table S3†). Overall, the infant gut microbiome was altered by complementary feeding during the weaning period (week 32 versus week 12), with minor differences observed in microbial trajectories, despite most bacteria overlapping between time points (Fig. 3b). Specifically, Bifidobacterium, Escherichia–Shigella, Streptococcus, and Bacteroides maintained similar relative abundance throughout weaning, while remarkable increases were observed in Blautia, Fusicatenibacter, Parasutterella, Akkermansia, etc. (Fig. 4a). In contrast, significant reductions were found in Lactobacillus, Escherichia–Shigella, Coprobacillus, Prevotella, etc. These continuous alterations during weaning suggest a potential mechanism through which complementary feeding affects the gut microbiome, further impacting infant growth and health. As shown in Fig. 4b, Escherichia–Shigella, Clostridium, Ruminococcus, Prevotella and Coprobacillus varied significantly between infants born via VD and those born via Cesarean section (C-section) at the beginning of this study. By the end of the study, variations became more apparent between breast-fed and formula-fed infants, with Parabacteroides and Clostridium being more prevalent in the breast-fed infants, and Leuconostoc and Sutterella were more common in the formula-fed infants (ESI Fig. S1a†). These variations might be associated with the fermentation of food metabolites, such as HMOs, although the underlying mechanisms are not fully understood. Finally, we observed that dietary supplementation such as vitamins, fish oil, probiotics (Bifidobacteria and Lactobacillus) and fruits were linked to changes in the abundance of Sellimonas, Peptostreptococcus, Parasutterella, Parabacteroides and Akkermansia. However, the precise mechanism remains to be elucidated (ESI Fig. S1b and S1c†).
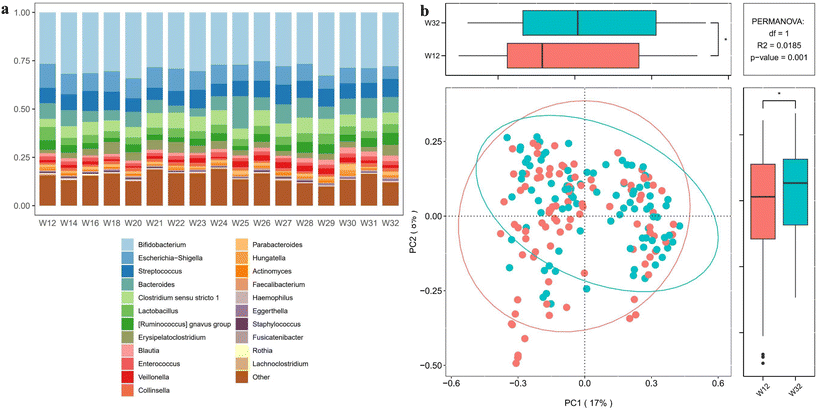 |
| Fig. 3 Top abundance gut microbiota in infant feces across the weaning period and statistical analysis. (a) Top abundance gut microbiome identified from infant feces in each week. (b) The principal component analysis with statistical analysis between week 12 and week 32. The significance of the gut microbiome is represented as *p < 0.05. | |
 |
| Fig. 4 The infant gut microbiome impacted by (a) the weaning period (comparison between week 12 and week 32) and (b) delivery mode (at week 12). In (a), red dots mean bacteria significantly enriched at week 32, yellow dots mean bacteria significantly enriched at week 12, and blue dots mean bacteria with no significant change between week 12 and week 32. In (b), red dots mean bacteria significantly enriched in vaginal delivery infants, and yellow dots mean bacteria significantly enriched in Cesarean section infants. All results are classified at the genus level. The significance was defined by a false discovery rate of <0.05. | |
3.5 Growth and health outcomes
A slight linear increase was observed in infant growth parameters, including body length, weight, and head circumference (Fig. S2 and Table S4†). These growth metrics did not exhibit a significant correlation with variables such as infant gender, birth age, family income or parental education (data not shown). A difference in growth rate was noted between infants who began complementary feeding before and after week 28, although it was not statistically significant. Infants introduced to complementary foods before week 28 demonstrated a higher growth rate, suggesting a potentially advantageous window for initiating complementary feeding between weeks 16 and 28. For health outcomes, spitting was the most frequent issue among infants, followed by jaundice, excessive crying, flatulence, and rash. The occurrence of these issues significantly reduced to 20% or below after week 20. There was also a potential correlation between the selection of complementary foods and the gut microbiome, as well as metabolic activity, although further investigation is required to confirm these association.
3.6 Correlative analysis
3.6.1 Microbiome diversity and metabolites.
Gut microbiome diversity is considered an important indicator of healthy growth, as higher diversity typically suggests better overall health. Using multiple parameters, including the Shannon index, Chao 1 and Simpson index, we explored the correlations between infant microbiota diversity and metabolites during weaning. As shown in Fig. 5a, both the Shannon and Simpson indexes were positively correlated with isovaleric acid, propionic acid, and acetic acid, while showing negative correlations with hydroxyaldehyde and lactic acid. The former acids are primarily generated from whole grains, leafy vegetables and high protein diets, and the latter are reduced, likely due to the introduction of complex carbohydrates and shifts in the gut microbiome. In addition, we found that the Shannon diversity index was negatively correlated with nearly all identified HMOs and showed no correlation with monosaccharides, although the mechanisms remained unclear (Fig. 5b and c).
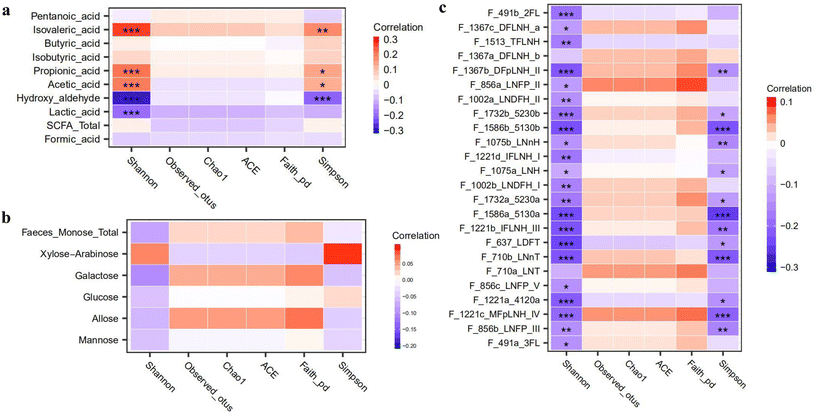 |
| Fig. 5 Correlations between gut microbiome diversity and metabolites: (a) alpha-diversity and short-chain fatty acids and (b) alpha-diversity and (c) fecal HMOs. No significant correlation was observed between alpha-diversity and HMOs in breastmilk. The significance is represented as *p < 0.05, **p < 0.01 and ***p < 0.001. | |
3.6.2 Metabolites, gut microbiome and their potential link to infant health.
Given the differences in the gut microbiome observed between VD and C-section infants at the start of this study, we divided the participants into two subgroups for further analysis. In VD infants, Streptococcus, Rothia, and Bifidobacteria showed positive correlations with the HMO production, particularly with LNT and LNnT. In addition, fewer genera such as Clostridium sensu stricto and Actinomyces were identified as influencing the concentration of 2′-FL and 3′-FL. Other HMOs such as lacto-N-hexaose and lacto-N-neohexaose also exhibited correlations with specific genera such as Prevotella, Bifidobacterium and Blautia. All details regarding individual bacterial genera correlated with HMOs are shown in Fig. 6. In C-section infants, a diverse group of genera including Escherichia–Shigella, Erysipelatoclostridium, and Clostridium sensu stricto were positively correlated with most HMOs. In detail, Parvimonas and Lachnoclostridium were correlated with the concentration of 2′-FL and 3′-FL, while Streptococcus and Clostridium sensu stricto were correlated with LNT and LNnT. Unlike VD infants, a broader spectrum of gut microbiota was associated with various HMOs, suggesting a potentially more immediate and efficient utilization of nutrients within the gut microbiome composition of C-section infants (Fig. 7). These microbiome-related alterations may be linked to reductions in common infant health issues such as flatulence, diarrhea and constipation during weaning. However, the exact mechanisms and individual responses to microbiome/metabolite variations require further exploration.
 |
| Fig. 6 Correlation between the infant gut microbiome at the phylum level and HMOs from vaginal delivery infants. The significance is represented as *p < 0.05, **p < 0.01 and ***p < 0.001. | |
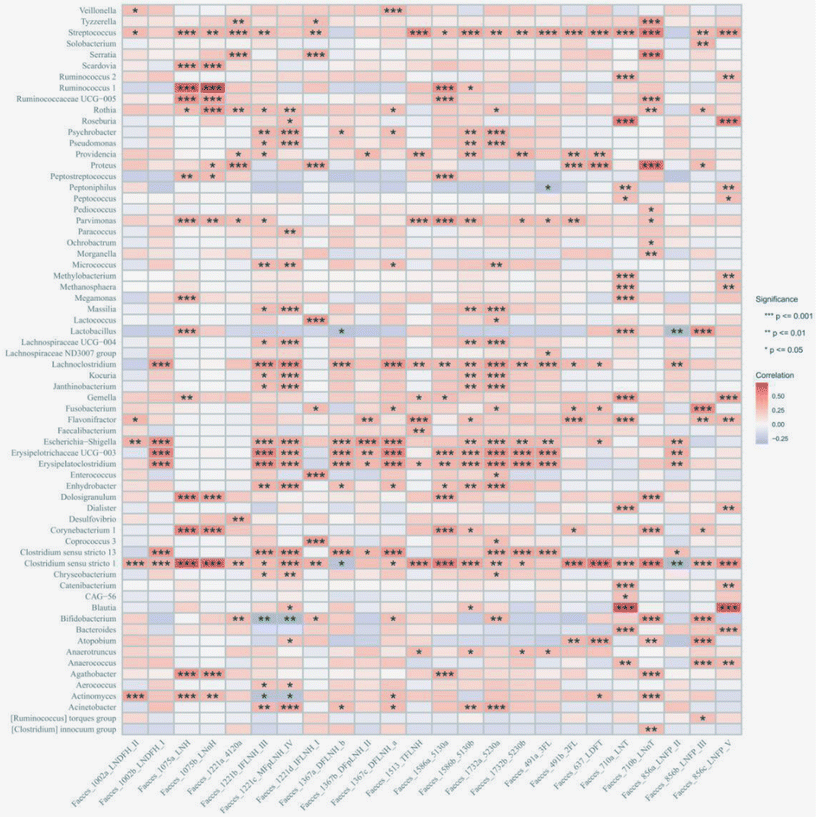 |
| Fig. 7 Correlation between the infant gut microbiome at the phylum level and HMOs from C-section infants. The significance is represented as *p < 0.05, **p < 0.01 and ***p < 0.001. | |
For other metabolites, Veillonella, Streptococcus, and Bacteroides were positively associated with most of the identified monosaccharides, while Clostridium innocuum showed negative associations in the VD infants (Fig. 8a). Specifically, Lactobacillus and Bifidobacteria had selective associations, showing negative correlations with allose and galactose while exhibiting positive correlations with other monosaccharides. In the C-section infants, Lactobacillus and Clostridium sensu stricto were positively associated with most of the monosaccharides, and glucose and xylose/arabinose showed strong positive correlations with most bacterial genera (Fig. 8b). For SCFAs, Lactobacillus showed positive correlations with most SCFAs, except for hydroxyaldehyde and lactic acid in the VD infants (Fig. 9a). However, in the C-section infants, it showed positive correlations only with isovaleric acid, pentanoic acid and propionic acid (Fig. 9b). Other genera, such as Bifidobacteria, Streptococcus and Clostridium sensu stricto, demonstrated similar correlative patterns across both groups, whereas Clostridium innocuum showed the opposite trends. No direct linkage was observed between health outcomes and changes in monosaccharides or SCFAs. Large studies with individual probiotic and metabolite interventions are needed to explore how gut microbiome and metabolite changes affect infant growth and health.
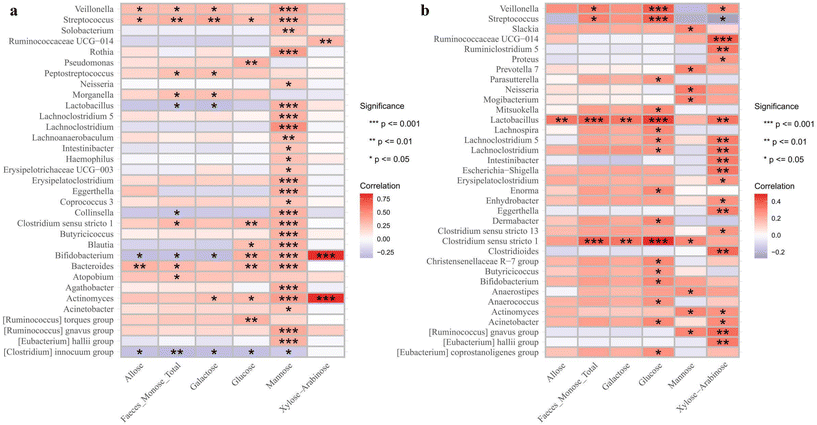 |
| Fig. 8 Correlation between the infant gut microbiome at the genus level and monosaccharides in (a) vaginal delivery and (b) C-section infants. The significance is represented as *p < 0.05, **p < 0.01 and ***p < 0.001. | |
 |
| Fig. 9 Correlation between the infant gut microbiome at the genus level and short-chain fatty acids in (a) vaginal delivery and (b) C-section infants. The significance is represented as *p < 0.05, **p < 0.01 and ***p < 0.001. | |
4. Discussion
The weaning period, which encompasses the first year of life, marks the transition from exclusive breast milk or formula feeding to the introduction of diverse foods, significantly impacting early infant development.43–45 Commercially prepared, homemade, culturally specific practices, and organic/non-organic complementary foods are recommended by health organizations and nutrition experts. These recommendations are tailored to the infant's developmental readiness and nutritional needs.46–48 During this period, the gut microbiome and metabolites undergo significant alterations, influenced by factors such as delivery mode, breastfeeding practices, and complementary feeding.49–51
Lactobacillus, predominant in the guts of breast-fed infants, decreased significantly during weaning, potentially influenced by dietary shifts in fiber content and competition for nutrients.52–54 In this study, we observed a significant 1.5-fold decrease in the Lactobacilli during the weaning period, with its relative abundance remaining consistent in the range from 0.3% to 0.7%, in accordance with findings from prior research.55 This reduction was primarily attributed to the introduction of complementary foods, which led to selective modifications in the infant gut microbiome, influenced by dietary changes in fiber content, fermentability, and oligosaccharide structures.56–61Akkermansia, a mucin-degrading bacterium, was initially present in only 16% of the infant intestinal tract at four weeks of age.62,63 Most notably, we found a more than 4-fold increase following the introduction of complementary feeding. This increase suggests a relationship between mucin utilization and microbial diversity during early life development. Akkermansia, which plays a critical role in maintaining gut barrier integrity and modulating immune responses, has been associated with various beneficial health outcomes in adults, including improved metabolic health and anti-inflammatory effects.64,65 The rise of Akkermansia during the weaning period might point to its role in early immune system maturation, as well as potential long-term health benefits in infants. Moreover, these findings highlighted the importance of the gut microbiome in early infant health and development. The shifts in microbiota composition during weaning, with reductions in Lactobacilli, an increase in Akkermansia spp., and the introduction of complementary foods over the period from week 16 to 28, suggested the dynamic relationship between diet and microbiome maturation. These microbial changes were not just markers of gut health but were closely tied to broader developmental outcomes, such as dietary supplementation.66 This finding warrants further exploration, particularly in how Akkermansia levels may be influenced by the specific types of complementary foods introduced, such as those rich in fiber and prebiotics.67,68 Future clinical practices could be informed by the observation that specific bacterial genera, such as Akkermansia, increase in response to dietary modifications during infancy.69 This suggests that strategically promoting the colonization of beneficial microbes through diet could be an important component of infant nutrition recommendations. For example, tailored dietary advice focusing on the inclusion of foods that promote Akkermansia and other beneficial genera could be considered as part of nutritional guidelines aimed at optimizing gut health and immune system development.70 Foods high in dietary fibers, prebiotics, and certain oligosaccharides could be prioritized, as these have been shown to selectively promote the growth of beneficial bacteria such as Akkermansia and Bifidobacterium.71,72
For metabolites, 2′-FLwas positively associated with height and weight gain in infants aged 3 to 12 months, while LNnT demonstrated a negative association.73 Similarly, 3′-FL positively correlates with various indicators of infant growth, including fat mass, weight-for-length and weight-for-age.74 A higher concentration of HMOs generally suggests enhanced health benefits like diminished incidence of viral diarrhea, bolstered immune system functions, and improved cognitive and brain development.75–77 Remarkably, Bacteroides fragilis, Bacteroides vulgatus, and Bacteroides thetaiotaomicron, frequently colonized in the infant gut through vaginal delivery and vertical transfer from the mother's gut microbiome,78 showed different capabilities of breaking down various HMOs.79–83 In addition, HMOs also selectively promoted the health beneficial bacteria such as Bifidobacterium, Lactobacillus and Streptococcus.80,82,84–87 Based on previous studies, there were significant differences in breastfeeding rates among ethnic groups in the United States, with the highest rate being in Asian Americans, which is similar to the high rate of breastfeeding in this study.88,89 This might provide a unique opportunity to improve infant development by exploring the alterations of HMO composition and metabolism from breast milk and its associated gut microbiome changes during weaning. Other metabolites, such as monosaccharides and SCFAs, were also impacted by the gut microbiome, complementary feeding, and birth weight, maternal age and antibiotic usage, within a concentration trajectory that varied significantly.90–97 To sum up, the introduction of a diverse range of complementary foods, particularly high-fiber foods such as cereals, fruits, and vegetables, significantly impacts the infant gut ecosystem through microbiome and metabolite interactions.98,99 Early infant development is intricate and challenging to study, yet it is of critical importance.
While this study provides valuable insights into the impact of complementary feeding on the metabolites, the gut microbiome and infant development, several limitations must be acknowledged. First, the selection of participants from a specific geographic region may limit the generalizability of the findings to broader populations with differing dietary practices and environmental exposures. In addition, the divergence in early microbial colonization caused by delivery modes has shown a long-term impact on the infant's immune system development and susceptibility to certain diseases later in life, which should be carefully considered and accounted for in future research designs. Moreover, potential confounding factors such as maternal diet, antibiotic use, and environmental influences were not fully controlled for, which could have affected the microbiome composition and development outcomes. The observational nature of the study, with a relatively small sample size, also limits the strength of the correlations between complementary food choices and changes in the gut microbiome and metabolites. Future research should aim to include a more diverse participant pool and incorporate randomized controlled trials to better establish causality and more accurately assess the long-term effects of various complementary feeding practices on infant health.
5. Conclusion
In this study, we observed a substantial change in the gut microbiome composition, with significant alterations in specific genera such as Lactobacillus, Bifidobacteria, and Akkermansia during the weaning period. There were distinct trends between the gut microbiome and various metabolites, including HMOs, monosaccharides and SCFAs. Bifidobacteria, mannose, butyric acid, LNT/LNnT, and 2′-FL, along with complementary foods, showed beneficial effects on infant development during the weaning period. These findings underscore the importance of tailored dietary interventions during the weaning period to promote optimal gut health and overall development in infants. Our work serves as a foundation for future investigations into the intricate relationships between diet, microbiome, and metabolic health in early infancy.
Abbreviations
SCFA | Short chain fatty acid |
HMO | Human milk oligosaccharide |
2′FL | 2′-Fucosyllactose |
3′FL | 3′-Fucosyllactose |
LNT | Lacto-N-tetraose |
LNnT | Lacto-N-neotetraose |
HPLC | High-performance liquid chromatography |
VD | Vaginal delivery |
C-section | Cesarean section |
Author contributions
YS and YW designed the study; YS, RY, JP, YC, ZL and SS conducted the sample collection; YS, RY, JP and YC conducted the experiments on both fecal and breast milk samples; YS and RY conducted the analysis on raw data; YS, RY and JP drafted the manuscript. All authors reviewed and approved the final version of the manuscript.
Data availability
The raw data including 16s rRNA sequencing raw data, analytical codes and other collected data that support the findings of this study are available from the corresponding author upon request.
Conflicts of interest
All authors from this study are employees of Inner Mongolia Mengniu Dairy (group) Co. Ltd, China. The authors declare that the study was conducted in the absence of any commercial or financial relationships that could be construed as a potential conflict of interest.
Acknowledgements
The authors want to acknowledge all the participants and their families for their support in this study. We also thank the Peking University Health Science Center and the Institute of Chemistry, Chinese Academy of Sciences, for their support in this study.
References
- J. Y. Meek, L. Noble and B. Section on, Policy Statement: Breastfeeding and the Use of Human Milk, Pediatrics, 2022, 150, 1–15 Search PubMed.
- R. Barachetti, E. Villa and M. Barbarini, Weaning and complementary feeding in preterm infants: management, timing and health outcome, Pediatr. Med. Chir., 2017, 39, 181 Search PubMed.
- F. J. Norris, M. S. Larkin, C. M. Williams, S. M. Hampton and J. B. Morgan, Factors affecting the introduction of complementary foods in the preterm infant, Eur. J. Clin. Nutr., 2002, 56, 448–454 CrossRef CAS PubMed.
- R. E. Black, L. H. Allen, Z. A. Bhutta, L. E. Caulfield, M. de Onis, M. Ezzati, C. Mathers and J. Rivera, Maternal and G. Child Undernutrition Study, Maternal and child undernutrition: global and regional exposures and health consequences, Lancet, 2008, 371, 243–260 CrossRef PubMed.
- N. F. Krebs and K. M. Hambidge, Complementary feeding: clinically relevant factors affecting timing and composition, Am. J. Clin. Nutr., 2007, 85, 639S–645S CrossRef CAS PubMed.
- K. G. Dewey, The challenge of meeting nutrient needs of infants and young children during the period of complementary feeding: an evolutionary perspective, J. Nutr., 2013, 143, 2050–2054 CrossRef CAS PubMed.
- M. Fewtrell, J. Bronsky, C. Campoy, M. Domellof, N. Embleton, N. Fidler Mis, I. Hojsak, J. M. Hulst, F. Indrio, A. Lapillonne and C. Molgaard, Complementary Feeding: A Position Paper by the European Society for Paediatric Gastroenterology, Hepatology, and Nutrition (ESPGHAN) Committee on Nutrition, J. Pediatr. Gastroenterol. Nutr., 2017, 64, 119–132 CrossRef CAS PubMed.
- M. Domellof, C. Braegger, C. Campoy, V. Colomb, T. Decsi, M. Fewtrell, I. Hojsak, W. Mihatsch, C. Molgaard, R. Shamir, D. Turck, J. van Goudoever and E. C. o. Nutrition, Iron requirements of infants and toddlers, J. Pediatr. Gastroenterol. Nutr., 2014, 58, 119–129 CrossRef PubMed.
- C. L. Wagner, F. R. Greer, B. American Academy of Pediatrics Section on and N. American Academy of Pediatrics Committee on, Prevention of rickets and vitamin D deficiency in infants, children, and adolescents, Pediatrics, 2008, 122, 1142–1152 CrossRef PubMed.
- T. A. Nicklas, C. E. O'Neil and V. L. Fulgoni, 3rd, Nutrient intake, introduction of baby cereals and other complementary foods in the diets of infants and toddlers from birth to 23 months of age, AIMS Public Health, 2020, 7, 123–147 Search PubMed.
- P. Nestel, A. Briend, B. de Benoist, E. Decker, E. Ferguson, O. Fontaine, A. Micardi and R. Nalubola, Complementary food supplements to achieve micronutrient adequacy for infants and young children, J. Pediatr. Gastroenterol. Nutr., 2003, 36, 316–328 CAS.
- E. E. Birch, J. C. Khoury, C. L. Berseth, Y. S. Castaneda, J. M. Couch, J. Bean, R. Tamer, C. L. Harris, S. H. Mitmesser and D. M. Scalabrin, The impact of early nutrition on incidence of allergic manifestations and common respiratory illnesses in children, J. Pediatr., 2010, 156, 902–906 CrossRef PubMed.
- C. Campoy, M. V. Escolano-Margarit, T. Anjos, H. Szajewska and R. Uauy, Omega 3 fatty acids on child growth, visual acuity and neurodevelopment, Br. J. Nutr., 2012, 107(Suppl 2), S85–106 CrossRef CAS PubMed.
- A. Argaw, K. P. Bouckaert, M. Wondafrash, P. Kolsteren, C. Lachat, B. De Meulenaer, G. Hanley-Cook and L. Huybregts, Effect of fish-oil supplementation on breastmilk long-chain polyunsaturated fatty acid concentration: a randomized controlled trial in rural Ethiopia, Eur. J. Clin. Nutr., 2021, 75, 809–816 CrossRef CAS PubMed.
- J. Rios, V. Valero-Jara and S. Thomas-Valdes, Phytochemicals in breast milk and their benefits for infants, Crit. Rev.
Food Sci. Nutr., 2022, 62, 6821–6836 CrossRef CAS PubMed.
- N. C. Mukonowenzou, K. A. Adeshina, J. Donaldson, K. G. Ibrahim, D. Usman and K. H. Erlwanger, Medicinal Plants, Phytochemicals, and Their Impacts on the Maturation of the Gastrointestinal Tract, Front. Physiol., 2021, 12, 684464 CrossRef PubMed.
- A. B. Stoica and C. Marginean, The Impact of Vitamin D Deficiency on Infants’ Health, Nutrients, 2023, 15, 4379 CrossRef CAS PubMed.
- C. Mathieu, C. Gysemans, A. Giulietti and R. Bouillon, Vitamin D and diabetes, Diabetologia, 2005, 48, 1247–1257 CrossRef CAS PubMed.
- J. L. Anderson, H. T. May, B. D. Horne, T. L. Bair, N. L. Hall, J. F. Carlquist, D. L. Lappe, J. B. Muhlestein and G. Intermountain Heart Collaborative Study, Relation of vitamin D deficiency to cardiovascular risk factors, disease status, and incident events in a general healthcare population, Am. J. Cardiol., 2010, 106, 963–968 CrossRef CAS PubMed.
- B. Lozoff and M. K. Georgieff, Iron deficiency and brain development, Semin. Pediatr. Neurol., 2006, 13, 158–165 CrossRef PubMed.
- W. Fan, G. Huo, X. Li, L. Yang and C. Duan, Impact of diet in shaping gut microbiota revealed by a comparative study in infants during the six months of life, J. Microbiol. Biotechnol., 2014, 24, 133–143 CrossRef CAS PubMed.
- A. C. Pantazi, A. L. Balasa, C. M. Mihai, T. Chisnoiu, V. V. Lupu, M. A. K. Kassim, L. Mihai, C. E. Frecus, S. I. Chirila, A. Lupu, A. Andrusca, C. Ionescu, V. Cuzic and S. C. Cambrea, Development of Gut Microbiota in the First 1000 Days after Birth and Potential Interventions, Nutrients, 2023, 15, 3647 CrossRef CAS PubMed.
- M. F. Laursen, L. B. Andersen, K. F. Michaelsen, C. Molgaard, E. Trolle, M. I. Bahl and T. R. Licht, Infant Gut Microbiota Development Is Driven by Transition to Family Foods Independent of Maternal Obesity, mSphere, 2016, 1, e00069–15 CrossRef CAS PubMed.
- M. Yassour, T. Vatanen, H. Siljander, A. M. Hamalainen, T. Harkonen, S. J. Ryhanen, E. A. Franzosa, H. Vlamakis, C. Huttenhower, D. Gevers, E. S. Lander, M. Knip, D. S. Group and R. J. Xavier, Natural history of the infant gut microbiome and impact of antibiotic treatment on bacterial strain diversity and stability, Sci. Transl. Med., 2016, 8, 343ra381 Search PubMed.
- J. L. Round and S. K. Mazmanian, The gut microbiota shapes intestinal immune responses during health and disease, Nat. Rev. Immunol., 2009, 9, 313–323 CrossRef CAS PubMed.
- L. V. Hooper, D. R. Littman and A. J. Macpherson, Interactions between the microbiota and the immune system, Science, 2012, 336, 1268–1273 CrossRef CAS PubMed.
- T. Ruohtula, M. C. de Goffau, J. K. Nieminen, J. Honkanen, H. Siljander, A. M. Hamalainen, A. Peet, V. Tillmann, J. Ilonen, O. Niemela, G. W. Welling, M. Knip, H. J. Harmsen and O. Vaarala, Maturation of Gut Microbiota and Circulating Regulatory T Cells and Development of IgE Sensitization in Early Life, Front. Immunol., 2019, 10, 2494 CrossRef CAS PubMed.
- M. G. Dominguez-Bello, E. K. Costello, M. Contreras, M. Magris, G. Hidalgo, N. Fierer and R. Knight, Delivery mode shapes the acquisition and structure of the initial microbiota across multiple body habitats in newborns, Proc. Natl. Acad. Sci. U. S. A., 2010, 107, 11971–11975 CrossRef PubMed.
- T. Yatsunenko, F. E. Rey, M. J. Manary, I. Trehan, M. G. Dominguez-Bello, M. Contreras, M. Magris, G. Hidalgo, R. N. Baldassano, A. P. Anokhin, A. C. Heath, B. Warner, J. Reeder, J. Kuczynski, J. G. Caporaso, C. A. Lozupone, C. Lauber, J. C. Clemente, D. Knights, R. Knight and J. I. Gordon, Human gut microbiome viewed across age and geography, Nature, 2012, 486, 222–227 CrossRef CAS PubMed.
- T. D. Shen, Diet and Gut Microbiota in Health and Disease, Nestle Nutr. Inst. Workshop Ser, 2017, 88, 117–126 Search PubMed.
- C. Milani, S. Duranti, F. Bottacini, E. Casey, F. Turroni, J. Mahony, C. Belzer, S. Delgado Palacio, S. Arboleya Montes, L. Mancabelli, G. A. Lugli, J. M. Rodriguez, L. Bode, W. de Vos, M. Gueimonde, A. Margolles, D. van Sinderen and M. Ventura, The First Microbial Colonizers of the Human Gut: Composition, Activities, and Health Implications of the Infant Gut Microbiota, Microbiol. Mol. Biol. Rev., 2017, 81, e00036–17 CrossRef PubMed.
- S. M. Totten, A. M. Zivkovic, S. Wu, U. Ngyuen, S. L. Freeman, L. R. Ruhaak, M. K. Darboe, J. B. German, A. M. Prentice and C. B. Lebrilla, Comprehensive profiles of human milk oligosaccharides yield highly sensitive and specific markers for determining secretor status in lactating mothers, J. Proteome Res., 2012, 11, 6124–6133 CrossRef CAS PubMed.
- S. Wu, N. Tao, J. B. German, R. Grimm and C. B. Lebrilla, Development of an annotated library of neutral human milk oligosaccharides, J. Proteome Res., 2010, 9, 4138–4151 CrossRef CAS PubMed.
- T. Sun, R. Yin, A. D. Magnuson, S. A. Tolba, G. Liu and X. G. Lei, Dose-Dependent Enrichments and Improved Redox Status in Tissues of Broiler Chicks under Heat Stress by Dietary Supplemental Microalgal Astaxanthin, J. Agric. Food Chem., 2018, 66, 5521–5530 CrossRef CAS PubMed.
- L. R. Hoving, M. Heijink, V. van Harmelen, K. W. van Dijk and M. Giera, GC-MS Analysis of Short-Chain Fatty Acids in Feces, Cecum Content, and Blood Samples, Methods Mol. Biol., 2018, 1730, 247–256 CrossRef CAS PubMed.
- X. Han, J. Guo, Y. You, M. Yin, C. Ren, J. Zhan and W. Huang, A fast and accurate way to determine short chain fatty acids in mouse feces based on GC-MS, J. Chromatogr. B: Anal. Technol. Biomed. Life Sci., 2018, 1099, 73–82 CrossRef CAS PubMed.
- W. Walters, E. R. Hyde, D. Berg-Lyons, G. Ackermann, G. Humphrey, A. Parada, J. A. Gilbert, J. K. Jansson, J. G. Caporaso, J. A. Fuhrman, A. Apprill and R. Knight, Improved Bacterial 16S rRNA Gene (V4 and V4-5) and Fungal Internal Transcribed Spacer Marker Gene Primers for Microbial Community Surveys, mSystems, 2016, 1, e00009–e00015 CrossRef PubMed.
- M. Estaki, L. Jiang, N. A. Bokulich, D. McDonald, A. Gonzalez, T. Kosciolek, C. Martino, Q. Zhu, A. Birmingham, Y. Vazquez-Baeza, M. R. Dillon, E. Bolyen, J. G. Caporaso and R. Knight, QIIME 2 Enables Comprehensive End-to-End Analysis of Diverse Microbiome Data and Comparative Studies with Publicly Available Data, Curr. Protoc. Bioinf., 2020, 70, e100 CrossRef PubMed.
- E. Bolyen, J. R. Rideout, M. R. Dillon, N. A. Bokulich, C. C. Abnet, G. A. Al-Ghalith, H. Alexander, E. J. Alm, M. Arumugam, F. Asnicar, Y. Bai, J. E. Bisanz, K. Bittinger, A. Brejnrod, C. J. Brislawn, C. T. Brown, B. J. Callahan, A. M. Caraballo-Rodriguez, J. Chase, E. K. Cope, R. Da Silva, C. Diener, P. C. Dorrestein, G. M. Douglas, D. M. Durall, C. Duvallet, C. F. Edwardson, M. Ernst, M. Estaki, J. Fouquier, J. M. Gauglitz, S. M. Gibbons, D. L. Gibson, A. Gonzalez, K. Gorlick, J. Guo, B. Hillmann, S. Holmes, H. Holste, C. Huttenhower, G. A. Huttley, S. Janssen, A. K. Jarmusch, L. Jiang, B. D. Kaehler, K. B. Kang, C. R. Keefe, P. Keim, S. T. Kelley, D. Knights, I. Koester, T. Kosciolek, J. Kreps, M. G. I. Langille, J. Lee, R. Ley, Y. X. Liu, E. Loftfield, C. Lozupone, M. Maher, C. Marotz, B. D. Martin, D. McDonald, L. J. McIver, A. V. Melnik, J. L. Metcalf, S. C. Morgan, J. T. Morton, A. T. Naimey, J. A. Navas-Molina, L. F. Nothias, S. B. Orchanian, T. Pearson, S. L. Peoples, D. Petras, M. L. Preuss, E. Pruesse, L. B. Rasmussen, A. Rivers, M. S. Robeson, 2nd, P. Rosenthal, N. Segata, M. Shaffer, A. Shiffer, R. Sinha, S. J. Song, J. R. Spear, A. D. Swafford, L. R. Thompson, P. J. Torres, P. Trinh, A. Tripathi, P. J. Turnbaugh, S. Ul-Hasan, J. J. J. van der Hooft, F. Vargas, Y. Vazquez-Baeza, E. Vogtmann, M. von Hippel, W. Walters, Y. Wan, M. Wang, J. Warren, K. C. Weber, C. H. D. Williamson, A. D. Willis, Z. Z. Xu, J. R. Zaneveld, Y. Zhang, Q. Zhu, R. Knight and J. G. Caporaso, Reproducible, interactive, scalable and extensible microbiome data science using QIIME 2, Nat. Biotechnol., 2019, 37, 852–857 CrossRef CAS PubMed.
- B. J. Callahan, J. Wong, C. Heiner, S. Oh, C. M. Theriot, A. S. Gulati, S. K. McGill and M. K. Dougherty, High-throughput amplicon sequencing of the full-length 16S rRNA gene with single-nucleotide resolution, Nucleic Acids Res., 2019, 47, e103 CrossRef CAS PubMed.
- R. Li, S. B. Fein, J. Chen and L. M. Grummer-Strawn, Why mothers stop breastfeeding: mothers’ self-reported reasons for stopping during the first year, Pediatrics, 2008, 122(Suppl. 2), S69–S76 CrossRef PubMed.
- K. Borewicz, F. Gu, E. Saccenti, I. C. W. Arts, J. Penders, C. Thijs, S. S. van Leeuwen, C. Lindner, A. Nauta, E. van Leusen, H. A. Schols and H. Smidt, Correlating Infant Fecal Microbiota Composition and Human Milk Oligosaccharide Consumption by Microbiota of 1-Month-Old Breastfed Infants, Mol. Nutr. Food Res., 2019, 63, e1801214 CrossRef PubMed.
- M. S. Fewtrell, A. Lucas and J. B. Morgan, Factors associated with weaning in full term and preterm infants, Arch. Dis. Child Fetal Neonatal. Ed., 2003, 88, F296–301 CrossRef CAS PubMed.
- B. Vail, P. Prentice, D. B. Dunger, I. A. Hughes, C. L. Acerini and K. K. Ong, Age at Weaning and Infant Growth: Primary Analysis and Systematic Review, J. Pediatr., 2015, 167, 317–324 CrossRef PubMed.
- K. D. Foote and L. D. Marriott, Weaning of infants, Arch. Dis. Child., 2003, 88, 488–492 CrossRef CAS PubMed.
- M. A. Abeshu, A. Lelisa and B. Geleta, Complementary Feeding: Review of Recommendations, Feeding Practices, and Adequacy of Homemade Complementary Food Preparations in Developing Countries - Lessons from Ethiopia, Front. Nutr., 2016, 3, 41 Search PubMed.
- D. Payet, M. Adjibade, J. Baudry, M. Ghozal, A. Camier, S. Nicklaus, K. Adel-Patient, A. Divaret-Chauveau, J. Gauvreau-Beziat, K. Vin, S. Lioret, M. A. Charles, E. Kesse-Guyot and B. de Lauzon-Guillain, Organic Food Consumption During the Complementary Feeding Period and Respiratory or Allergic Diseases Up to Age 5.5 Years in the ELFE Cohort, Front. Nutr., 2021, 8, 791430 CrossRef PubMed.
- K. H. Paul, M. Muti, B. Chasekwa, M. N. Mbuya, R. C. Madzima, J. H. Humphrey and R. J. Stoltzfus, Complementary feeding messages that target cultural barriers enhance both the use of lipid-based nutrient supplements and underlying feeding practices to improve infant diets in rural Zimbabwe, Mat. Child Nutr., 2012, 8, 225–238 CrossRef PubMed.
- S. McKeen, W. Young, J. Mullaney, K. Fraser, W. C. McNabb and N. C. Roy, Infant Complementary Feeding of Prebiotics for theMicrobiome and Immunity, Nutrients, 2019, 11, 364 CrossRef CAS PubMed.
- N. Li, F. Yan, N. Wang, Y. Song, Y. Yue, J. Guan, B. Li and G. Huo, Distinct Gut Microbiota and Metabolite Profiles Induced by Different Feeding Methods in Healthy Chinese Infants, Front. Microbiol., 2020, 11, 714 CrossRef PubMed.
- N. Li, S. Liang, Q. Chen, L. Zhao, B. Li and G. Huo, Distinct gut microbiota and metabolite profiles induced by delivery mode in healthy Chinese infants, J. Proteomics, 2021, 232, 104071 CrossRef CAS PubMed.
- R. Wall, R. P. Ross, C. A. Ryan, S. Hussey, B. Murphy, G. F. Fitzgerald and C. Stanton, Role of gut microbiota in early infant development, Clin. Med. Pediatr., 2009, 3, 45–54 CAS.
- J. Durack, N. E. Kimes, D. L. Lin, M. Rauch, M. McKean, K. McCauley, A. R. Panzer, J. S. Mar, M. D. Cabana and S. V. Lynch, Delayed gut microbiota development in high-risk for asthma infants is temporarily modifiable by Lactobacillus supplementation, Nat. Commun., 2018, 9, 707 CrossRef PubMed.
- Y. Vandenplas, Oligosaccharides in infant formula, Br. J. Nutr., 2002, 87(Suppl 2), S293–S296 CrossRef CAS PubMed.
- M. Haarman and J. Knol, Quantitative real-time PCR analysis of fecal Lactobacillus species in infants receiving a prebiotic infant formula, Appl. Environ. Microbiol., 2006, 72, 2359–2365 CrossRef CAS PubMed.
- K. M. Van Laere, R. Hartemink, M. Bosveld, H. A. Schols and A. G. Voragen, Fermentation of plant cell wall derived polysaccharides and their corresponding oligosaccharides by intestinal bacteria, J. Agric. Food Chem., 2000, 48, 1644–1652 CrossRef CAS PubMed.
- S. Verkhnyatskaya, M. Ferrari, P. de Vos and M. T. C. Walvoort, Shaping the Infant Microbiome With Non-digestible Carbohydrates, Front. Microbiol., 2019, 10, 343 CrossRef PubMed.
- S. Macfarlane, G. T. Macfarlane and J. H. Cummings, Review article: prebiotics in the gastrointestinal tract, Aliment. Pharmacol. Ther., 2006, 24, 701–714 CrossRef CAS PubMed.
- C. E. Rycroft, M. R. Jones, G. R. Gibson and R. A. Rastall, A comparative in vitro evaluation of the fermentation properties of prebiotic oligosaccharides, J. Appl. Microbiol., 2001, 91, 878–887 CrossRef CAS PubMed.
- C. L. Vernazza, G. R. Gibson and R. A. Rastall, Carbohydrate preference, acid tolerance and bile tolerance in five strains of Bifidobacterium, J. Appl. Microbiol., 2006, 100, 846–853 CrossRef CAS PubMed.
- S. Fanaro, J. Jelinek, B. Stahl, G. Boehm, R. Kock and V. Vigi, Acidic oligosaccharides from pectin hydrolysate as new component for infant formulae: effect on intestinal flora, stool characteristics, and pH, J. Pediatr. Gastroenterol. Nutr., 2005, 41, 186–190 CrossRef CAS PubMed.
- J. S. Glover, T. D. Ticer and M. A. Engevik, Characterizing the mucin-degrading capacity of the human gut microbiota, Sci. Rep., 2022, 12, 8456 CrossRef CAS PubMed.
- M. C. Collado, M. Derrien, E. Isolauri, W. M. de Vos and S. Salminen, Intestinal integrity and Akkermansia muciniphila, a mucin-degrading member of the intestinal microbiota present in infants, adults, and the elderly, Appl. Environ. Microbiol., 2007, 73, 7767–7770 CrossRef CAS PubMed.
- M. Derrien, E. E. Vaughan, C. M. Plugge and W. M. de Vos, Akkermansia muciniphila gen. nov., sp. nov., a human intestinal mucin-degrading bacterium, Int. J. Syst. Evol. Microbiol., 2004, 54, 1469–1476 CrossRef CAS PubMed.
- C. Belzer and W. M. de Vos, Microbes inside–from diversity to function: the case of Akkermansia, ISME J., 2012, 6, 1449–1458 CrossRef CAS PubMed.
- D. Rios-Covian, P. Ruas-Madiedo, A. Margolles, M. Gueimonde, C. G. de Los Reyes-Gavilan and N. Salazar, Intestinal Short Chain Fatty Acids and their Link with Diet and Human Health, Front. Microbiol., 2016, 7, 185 Search PubMed.
- A. Everard, C. Belzer, L. Geurts, J. P. Ouwerkerk, C. Druart, L. B. Bindels, Y. Guiot, M. Derrien, G. G. Muccioli, N. M. Delzenne, W. M. de Vos and P. D. Cani, Cross-talk between Akkermansia muciniphila and intestinal epithelium controls diet-induced obesity, Proc. Natl. Acad. Sci. U. S. A., 2013, 110, 9066–9071 CrossRef CAS PubMed.
- P. D. Cani, C. Depommier, M. Derrien, A. Everard and W. M. de Vos, Akkermansia muciniphila: paradigm for next-generation beneficial microorganisms, Nat. Rev. Gastroenterol. Hepatol., 2022, 19, 625–637 CrossRef PubMed.
- M. C. Dao, A. Everard, J. Aron-Wisnewsky, N. Sokolovska, E. Prifti, E. O. Verger, B. D. Kayser, F. Levenez, J. Chilloux, L. Hoyles, M. I.-O. Consortium, M. E. Dumas, S. W. Rizkalla, J. Dore, P. D. Cani and K. Clement, Akkermansia muciniphila and improved metabolic health during a dietary intervention in obesity: relationship with gut microbiome richness and ecology, Gut, 2016, 65, 426–436 CrossRef CAS PubMed.
- H. Plovier, A. Everard, C. Druart, C. Depommier, M. Van Hul, L. Geurts, J. Chilloux, N. Ottman, T. Duparc, L. Lichtenstein, A. Myridakis, N. M. Delzenne, J. Klievink, A. Bhattacharjee, K. C. van der Ark, S. Aalvink, L. O. Martinez, M. E. Dumas, D. Maiter, A. Loumaye, M. P. Hermans, J. P. Thissen, C. Belzer, W. M. de Vos and P. D. Cani, A purified membrane protein from Akkermansia muciniphila or the pasteurized bacterium improves metabolism in obese and diabetic mice, Nat. Med., 2017, 23, 107–113 CrossRef CAS PubMed.
- F. Turroni, C. Milani, S. Duranti, J. Mahony, D. van Sinderen and M. Ventura, Glycan Utilization and Cross-Feeding Activities by Bifidobacteria, Trends Microbiol., 2018, 26, 339–350 CrossRef CAS PubMed.
- R. Zhai, X. Xue, L. Zhang, X. Yang, L. Zhao and C. Zhang, Strain-Specific Anti-inflammatory Properties of Two Akkermansia muciniphila Strains on Chronic Colitis in Mice, Front. Cell. Infect. Microbiol., 2019, 9, 239 CrossRef CAS PubMed.
- H. Lagstrom, S. Rautava, H. Ollila, A. Kaljonen, O. Turta, J. Makela, C. Yonemitsu, J. Gupta and L. Bode, Associations between human milk oligosaccharides and growth in infancy and early childhood, Am. J. Clin. Nutr., 2020, 111, 769–778 CrossRef PubMed.
- J. L. Saben, C. R. Sims, A. Abraham, L. Bode and A. Andres, Human Milk Oligosaccharide Concentrations and Infant Intakes Are Associated with Maternal Overweight and Obesity and Predict Infant Growth, Nutrients, 2021, 13, 446 CrossRef CAS PubMed.
- A. L. Morrow, G. M. Ruiz-Palacios, M. Altaye, X. Jiang, M. L. Guerrero, J. K. Meinzen-Derr, T. Farkas, P. Chaturvedi, L. K. Pickering and D. S. Newburg, Human milk oligosaccharides are associated with protection against diarrhea in breast-fed infants, J. Pediatr., 2004, 145, 297–303 CrossRef CAS PubMed.
- P. K. Berger, R. Bansal, S. Sawardekar, C. Yonemitsu, A. Furst, H. E. Hampson, K. A. Schmidt, T. L. Alderete, L. Bode, M. I. Goran and B. S. Peterson, Associations of Human Milk Oligosaccharides with Infant Brain Tissue Organization and Regional Blood Flow at 1 Month of Age, Nutrients, 2022, 14, 3820 CrossRef CAS PubMed.
- P. K. Berger, J. F. Plows, R. B. Jones, T. L. Alderete, C. Yonemitsu, M. Poulsen, J. H. Ryoo, B. S. Peterson, L. Bode and M. I. Goran, Human milk oligosaccharide 2′-fucosyllactose links feedings at 1 month to cognitive development at 24 months in infants of normal and overweight mothers, PLoS One, 2020, 15, e0228323 CrossRef CAS PubMed.
- Y. Shao, S. C. Forster, E. Tsaliki, K. Vervier, A. Strang, N. Simpson, N. Kumar, M. D. Stares, A. Rodger, P. Brocklehurst, N. Field and T. D. Lawley, Stunted microbiota and opportunistic pathogen colonization in caesarean-section birth, Nature, 2019, 574, 117–121 CrossRef CAS PubMed.
- J. M. Grondin, K. Tamura, G. Dejean, D. W. Abbott and H. Brumer, Polysaccharide Utilization Loci: Fueling Microbial Communities, J. Bacteriol., 2017, 199, e00860–16 CrossRef CAS PubMed.
- A. Marcobal, M. Barboza, J. W. Froehlich, D. E. Block, J. B. German, C. B. Lebrilla and D. A. Mills, Consumption of human milk oligosaccharides by gut-related microbes, J. Agric. Food Chem., 2010, 58, 5334–5340 CrossRef CAS PubMed.
- A. Marcobal, M. Barboza, E. D. Sonnenburg, N. Pudlo, E. C. Martens, P. Desai, C. B. Lebrilla, B. C. Weimer, D. A. Mills, J. B. German and J. L. Sonnenburg, Bacteroides in the infant gut consume milk oligosaccharides via mucus-utilization pathways, Cell Host Microbe, 2011, 10, 507–514 CrossRef CAS PubMed.
- Z. T. Yu, C. Chen and D. S. Newburg, Utilization of major fucosylated and sialylated human milk oligosaccharides by isolated human gut microbes, Glycobiology, 2013, 23, 1281–1292 CrossRef CAS PubMed.
- A. C. Masi and C. J. Stewart, Untangling human milk oligosaccharides and infant gut microbiome, iScience, 2022, 25, 103542 CrossRef CAS PubMed.
- B. M. Vester Boler, M. C. Rossoni Serao, T. A. Faber, L. L. Bauer, J. Chow, M. R. Murphy and G. C. Fahey, Jr., In vitro fermentation characteristics of select nondigestible oligosaccharides by infant fecal inocula, J. Agric. Food Chem., 2013, 61, 2109–2119 CrossRef CAS PubMed.
- Z. T. Yu, C. Chen, D. E. Kling, B. Liu, J. M. McCoy, M. Merighi, M. Heidtman and D. S. Newburg, The principal fucosylated oligosaccharides of human milk exhibit prebiotic properties on cultured infant microbiota, Glycobiology, 2013, 23, 169–177 CrossRef CAS PubMed.
- R. G. Locascio, M. R. Ninonuevo, S. R. Kronewitter, S. L. Freeman, J. B. German, C. B. Lebrilla and D. A. Mills, A versatile and scalable strategy for glycoprofiling bifidobacterial consumption of human milk oligosaccharides, Microb. Biotechnol., 2009, 2, 333–342 CrossRef CAS PubMed.
- K. Salli, J. Hirvonen, J. Siitonen, I. Ahonen, H. Anglenius and J. Maukonen, Selective Utilization of the Human Milk Oligosaccharides 2′-Fucosyllactose, 3-Fucosyllactose, and Difucosyllactose by Various Probiotic and Pathogenic Bacteria, J. Agric. Food Chem., 2021, 69, 170–182 CrossRef CAS PubMed.
- A. Ross, C. MacPherson, L. Baker, S. Kim, G. Njau and A. D. Williams, Impact of Breastfeeding Barriers on Racial/Ethnic Disparities in Breastfeeding Outcomes in North Dakota, J. Racial and Ethnic Health Disparities, 2024 DOI:10.1007/s40615-024-01943-z.
- K. M. Jones, M. L. Power, J. T. Queenan and J. Schulkin, Racial and ethnic disparities in breastfeeding, Breastfeed. Med., 2015, 10, 186–196 CrossRef PubMed.
- M. Wu, N. P. McNulty, D. A. Rodionov, M. S. Khoroshkin, N. W. Griffin, J. Cheng, P. Latreille, R. A. Kerstetter, N. Terrapon, B. Henrissat, A. L. Osterman and J. I. Gordon, Genetic determinants of in vivo fitness and diet responsiveness in multiple human gut Bacteroides, Science, 2015, 350, aac5992 CrossRef PubMed.
- C. Milani, G. A. Lugli, S. Duranti, F. Turroni, L. Mancabelli, C. Ferrario, M. Mangifesta, A. Hevia, A. Viappiani, M. Scholz, S. Arioli, B. Sanchez, J. Lane, D. V. Ward, R. Hickey, D. Mora, N. Segata, A. Margolles, D. van Sinderen and M. Ventura, Bifidobacteria exhibit social behavior through carbohydrate resource sharing in the gut, Sci. Rep., 2015, 5, 15782 CrossRef CAS PubMed.
- N. Tsukuda, K. Yahagi, T. Hara, Y. Watanabe, H. Matsumoto, H. Mori, K. Higashi, H. Tsuji, S. Matsumoto, K. Kurokawa and T. Matsuki, Key bacterial taxa and metabolic pathways affecting gut short-chain fatty acid profiles in early life, ISME J., 2021, 15, 2574–2590 CrossRef CAS PubMed.
- M. Nilsen, C. M. Saunders, I. L. Angell, M. O. Arntzen, K. C. L. Carlsen, K. H. Carlsen, G. Haugen, L. H. Hagen, M. H. Carlsen, G. Hedlin, C. M. Jonassen, B. Nordlund, E. M. Rehbinder, H. O. Skjerven, L. Snipen, A. C. Staff, R. Vettukattil and K. Rudi, Butyrate Levels in the Transition from an Infant- to an Adult-Like Gut Microbiota Correlate with Bacterial Networks Associated with Eubacterium Rectale and Ruminococcus Gnavus, Genes, 2020, 11, 1245 CrossRef CAS PubMed.
- S. L. Bridgman, M. B. Azad, C. J. Field, A. M. Haqq, A. B. Becker, P. J. Mandhane, P. Subbarao, S. E. Turvey, M. R. Sears, J. A. Scott, D. S. Wishart, A. L. Kozyrskyj and C. S. Investigators, Fecal Short-Chain Fatty Acid Variations by Breastfeeding Status in Infants at 4 Months: Differences in Relative versus Absolute Concentrations, Front. Nutr., 2017, 4, 11 Search PubMed.
- C. T. Pekmez, M. W. Larsson, M. V. Lind, N. Vazquez Manjarrez, C. Yonemitsu, A. Larnkjaer, L. Bode, C. Molgaard, K. F. Michaelsen and L. O. Dragsted, Breastmilk Lipids and Oligosaccharides Influence Branched Short-Chain Fatty Acid Concentrations in Infants with Excessive Weight Gain, Mol. Nutr. Food Res., 2020, 64, e1900977 CrossRef PubMed.
- F. Moens, P. Van den Abbeele, A. W. Basit, C. Dodoo, R. Chatterjee, B. Smith and S. Gaisford, A four-strain probiotic exerts positive immunomodulatory effects by enhancing colonic butyrate production in vitro, Int. J. Pharm., 2019, 555, 1–10 CrossRef CAS PubMed.
- L. Wang, J. Zhang, Z. Guo, L. Kwok, C. Ma, W. Zhang, Q. Lv, W. Huang and H. Zhang, Effect of oral consumption of probiotic Lactobacillus planatarum P-8 on fecal microbiota, SIgA, SCFAs, and TBAs of adults of different ages, Nutrition, 2014, 30, 776–783 CrossRef CAS PubMed.
- K. Salli, H. Anglenius, J. Hirvonen, A. A. Hibberd, I. Ahonen, M. T. Saarinen, K. Tiihonen, J. Maukonen and A. C. Ouwehand, The effect of 2′-fucosyllactose on simulated infant gut microbiome and metabolites; a pilot study in comparison to GOS and lactose, Sci. Rep., 2019, 9, 13232 CrossRef PubMed.
- G. Casaburi, R. M. Duar, H. Brown, R. D. Mitchell, S. Kazi, S. Chew, O. Cagney, R. L. Flannery, K. G. Sylvester, S. A. Frese, B. M. Henrick and S. L. Freeman, Metagenomic insights of the infant microbiome community structure and function across multiple sites in the United States, Sci. Rep., 2021, 11, 1472 CrossRef CAS PubMed.
|
This journal is © The Royal Society of Chemistry 2024 |
Click here to see how this site uses Cookies. View our privacy policy here.