DOI:
10.1039/D4FO01650E
(Paper)
Food Funct., 2024,
15, 9563-9578
Effect of dark sweet cherry (Prunus avium) supplementation on the fecal microbiota, metabolic endotoxemia, and intestinal permeability in obese subjects: a single-blind randomized trial†
Received
9th April 2024
, Accepted 21st August 2024
First published on 27th August 2024
Abstract
This single blind placebo-controlled study has as its main objectives to investigate the influence of dark sweet cherries (DSC) consumption on obesity-related dysbiosis, metabolic endotoxemia, and intestinal permeability. Participants (>18 years old, BMI: 30–40 kg m−2) consumed 200 mL of DSC juice with 3 g of DSC powder (n = 19) or a placebo drink (n = 21) twice per day for 30 days. The gut microbiota abundance was investigated using 16S ribosomal RNA sequencing on fecal DNA. Metabolic endotoxemia was evaluated by measuring lipopolysaccharide-binding protein (LBP) in fasting plasma samples. Intestinal permeability was assessed using the lactulose/mannitol (L/M) test and by measuring regeneration islet-derived protein 4 (REG4), and interleukin-22 (IL-22) mRNA levels in stool samples. Results showed that DSC supplementation decreased the abundance of Anaerostipes hadrus (p = 0.02) and Blautia (p = 0.04), whose changes were significant in BMI ≥ 35 participants (p = 0.004 and p = 0.006, respectively). Additionally, DSC prevented the increase of Alistipes shahii (p = 0.005) and Bilophila (p = 0.01) compared to placebo. Notably, DSC intervention favored the abundance of bacteria supporting a healthy gut ecosystem such as Roseburia intestinalis (p = 0.01), Turicibacter (p = 0.01), and Bacteroides vulgatus (p = 0.003) throughout the intervention, along with Clostridium leptum (p = 0.03) compared to placebo. The LBP, L/M ratio, REG-4 and IL-22 mRNA levels remained unchanged in placebo and cherry groups, implying that participants did not experience alterations in intestinal permeability. These findings highlight the potential gut-health benefits of DSC and encourage future research among individuals with BMI ≥ 35 and increased intestinal permeability.
1. Introduction
Research over the last years has demonstrated that an imbalance in the gut microbiota, also known as dysbiosis, can contribute to the development and progression of obesity. To contextualize dysbiosis in relation to obesity, several factors should be considered, including the biological role of gut bacteria in regulating metabolism and fat storage, as well as the connection between dysbiosis, inflammation, and hormonal dysregulation. For example, obesity-related dysbiosis is linked to intestinal barrier dysfunction and increased gut permeability to bacterial lipopolysaccharides (LPS), resulting in metabolic endotoxemia, low-grade inflammation and development of metabolic disorders including insulin resistance.1,2 Therefore, understanding the relationship between dysbiosis and obesity underscores the importance of maintaining a healthy gut microbiome for overall metabolic health and presents potential avenues for therapeutic interventions in obesity.
Diet is one of the major factors driving changes in the gut microbiota composition. The Western diet characterized by high fat/high sucrose and low in fiber intake has an impact on promoting dysbiosis. This diet has been shown to induce an abundance of bacterial species belonging to the Proteobacteria phylum, particularly facultative anaerobic bacteria of the Enterobacteriaceae family, which can induce intestinal mucosa dysbiosis, sustain and aggravate chronic inflammation, and metabolic diseases. In contrast, the consumption of plant-based foods rich in bioactive compounds (i.e., fiber and polyphenols) has emerged as a possible dietary intervention against dysbiosis. Accumulating evidence suggests that the beneficial effects exerted by food-bioactive compounds are partially mediated by changes induced in the gut microbiota composition. Sweet and tart cherries are stone fruits considered a promising option for these interventions due to their content of bioactive compounds that can reach the lower intestinal tract, mostly phenolic compounds and fiber. While there are some differences in the specific concentrations and profiles of bioactive compounds, tart and sweet cherries share many similarities in their food chemistry and health-promoting properties.
Consumer awareness of the health benefits of cherries has driven the rise in both production and consumption, particularly for sweet cherries whose global production increased from 1.9 to 2.32 million tons between 2000 and 2016; while sour cherry production remained stable (around 1.1 to 1.3 million tons) during the same period.3 The rise in sweet cherry production and consumption is primarily due to their popularity for fresh consumption, appreciated for their taste, texture, and color, whereas sour cherries are more commonly processed. As reviewed in Faienza et al.,4 sweet cherries contain fiber (2.1 g/100 g), hydrosoluble (C, B) and liposoluble vitamins (A, E and K), carotenoids (beta-carotene, some lutein and zeaxanthin), minerals (calcium, magnesium, phosphorous and potassium) and melatonin, which might protect against oxidative stress. Both tart and sweet cherries contain high levels of polyphenols, which contribute to their health benefits, mainly due to their activity in counteracting oxidative stress and reducing inflammation. The antioxidant activity of sweet and sour cherries varies depending on the cultivar and growing location (Chockchaisawasdee et al., 2016 cited in Blando et al.3). A study by Pissard et al. (2016), cited in Blando et al.,3 found that sour cherries generally exhibited higher antioxidant activity (DPPH and ORAC) than sweet cherries (5.45 vs. 3.69 and 79.64 vs. 48.41 μmol TE g−1 FW, respectively). However, another study by Prior et al. (2016), also cited in Blando et al.,3 reported that the total ORAC of sweet cherries was higher than that of sour cherries (864 vs. 772 μmol TE g−1 DW).
Common polyphenols in cherries include phenolic acids (hydroxycinnamic acids) and flavonoids (anthocyanins, flavan-3-ols and flavonols). Results from HPLC-MS/MS analysis of DSC concentrated juice used for this study,5 showed that flavan-3-ols, (e.g. procyanidin dimers), followed by anthocyanins were the most abundant class of phenolic compounds. Likewise, cyanidin-3-rutinoside showed to be the principal anthocyanin in DSC juice followed by cyanidin 3-glucoside as was also reported for 24 sweet cherry cultivars grown in Sicily, Italy.4 Anthocyanins are known for their beneficial effects on meta-inflammation triggered by obesity, which is associated with chronic conditions such as type 2 diabetes, hypertension, cardiovascular disease, and cancer.6
Tart cherries have been studied more frequently than sweet cherries. Given their similar bioactive compound profiles, it is relevant to reference studies that examine the effects of tart cherry supplementation on gut microbiota composition. Montmorency tart cherry supplementation (60 mL day−1) for 4 weeks did not influence fecal microbiota in middle-aged population.7 Similarly, there were no significant changes in the composition of the fecal microbiota in healthy participants after 30-day intake of Montmorency tart cherry as concentrate or freeze-dried form.8 A short-term human dietary intervention (5 days, 8 oz. tart cherry daily) reported two distinct and inverse responses associated with initial levels of Bacteroides. In this study, the high-Bacteroides individuals responded with a decrease in Bacteroides and Bifidobacterium and an increase of Lachnospiraceae, Ruminococcus and Collinsella. Low-Bacteroides individuals responded with an increase in Bacteroides or Prevotella and Bifidobacterium, and a decrease of Lachnospiraceae, Ruminococcus and Collinsella. This study suggested that different metabotypes need to be considered when analyzing the effects of tart cherries consumption on the gut microbiota.9 Controversial results from human studies are in part the results of the well-known high inter-individual variation in gut microbiota composition, dose and time of supplementation, underlying diets, and health status of participants.10 Although data from animal studies is limited, there is clearer evidence of benefits for gut health and host metabolic parameters. Montmorency tart cherry supplementation for 12 weeks improved markers of glucose homeostasis, increased Actinobacteria, and reduced Proteobacteria and Deferribacteres phyla, as well as fecal short-chain fatty acids (SCFAs) in mice fed a Western diet.11 Tart and sweet cherry concentrate juices were evaluated at increased concentrations to determine their potential effect on the murine gut microbiota composition.12 This study reported microbiota modulation based on concentration regardless of the juice type, with significant increase on relative abundance of Barnesiella and Akkermansia whereas Bacteroides abundance was negatively correlated with the concentration of the juice. Previous work from our research group has shown that DSC powder supplementation (10%) for 12 weeks promoted changes in the fecal microbiota population at different taxonomic levels in obese diabetic (db/db) mice.13 In this study, the relative abundance of Proteobacteria (Enterobacteriaceae family), usually associated with harmful effects for intestinal health, was similar between the healthy lean control and db/db mice fed DSC-supplemented diet, and in significantly lower relative abundance than the db/db control mice. Likewise, the relative abundance of Akkermansia muciniphila (a member of Verrucomicrobia phylum), which has been inversely correlated with inflammatory conditions and intestinal barrier dysfunction, was significantly higher in DSC-supplemented db/db mice than in db/db and lean controls. Based on this evidence, it was hypothesized that DSC intake induces a favorable modulation of gut microbiota in obese adults, and this effect might be accompanied by improved intestinal barrier function. Therefore, this study aimed to assess the effects of a 30-day DSC intervention on the fecal microbiota and biomarkers of intestinal barrier function in obese subjects.
2. Materials and methods
2.1 Study design and participant eligibility
A detailed description of the study design and participant eligibility has been previously reported.14 Briefly, participants were recruited between January 2020 and August 2021 according to the following inclusion criteria: age: ≥18 years old, body mass index (BMI): ≥30 and ≤40 and without history of chronic disease or intestinal disorders, and willingness to stop taking nutritional supplements. Participants were excluded if they had any of the following conditions within the previous 6 months: acute cardiac event, stroke, cancer, alcohol or substance abuse, hepatitis (B or C) or HIV, liver or renal dysfunction, history of dizziness/fainting during and after blood draws, gluten sensitivity or celiac disease. Antibiotic exposure, recurrent admittance to the hospital (twice or more), excessive drinking, allergy or sensitivity to berries, pregnancy/lactation, and smoking were also considered exclusion criteria. Participants who were lactose intolerant were excluded from the urine test aimed to assess intestinal permeability. After completion of a 2-week run-in period in which participants were asked to stop taking nutritional supplements and to refrain from polyphenol-rich foods, participants were assigned to cherry or placebo groups following the adaptive randomization method to minimize the imbalance of prognostic factors (BMI, gender and age).15 The single-blind randomized study design helped maintain participant compliance and reduce potential dropout rates, given the complexity and length of the intervention. Study protocol was approved by the Institutional Review Board (IRB2019-0597F) at Texas A&M University and registered at clinicaltrials.gov as NCT05586386.
2.2 DSC supplementation
DSC concentrated juice, kindly provided by FruitSmart® (Grandview, WA), was supplemented with DSC powder, kindly provided by Anderson Advance Ingredients (Irvine, CA). DSC juice supplemented with DSC powder was individually packaged in 50 mL sterile centrifuge tubes for each dose, kept frozen during transportation to the participants’ homes and stored at −20 °C throughout the study. This storage method prevented enzymatic activity, chemical reactions, microbial growth, and light-induced degradation. The placebo concentrated drink was prepared by study personnel following good manufacturing practices in the Department of Food Science and Technology at Texas A&M University and stored at −20 °C.14 The reconstituted placebo drink was formulated to match Brix, color and sensory characteristics of the DSC drink. The nutritional and physicochemical characteristics of DSC concentrated juice, DSC powder, and formulation of placebo concentrated drink were previously reported in detailed,14 and can be accessed at: https://www.mdpi.com/article/10.3390/nu15030681/s1. Participants were instructed to reconstitute DSC or placebo concentrated drinks with 150 mL of water and consume twice per day for 30 days without modifying their dietary patterns and physical activity. DSC reconstituted drink contained the bioactive compounds provided by the DSC juice and the DSC powder that sum to 0.11 g of fiber, 439.6 mg total phenolics as gallic acid equivalents, and 70.21 mg cyanidin 3-glucoside of anthocyanis.14 Participants were given verbal and written instructions to continue refraining from polyphenol-rich foods and nutritional supplements as in the run-in period. A schematic representation of the study schedule is presented in ESI Fig. 1.†
2.3 Anthropometric, physiological, and dietary assessments
Anthropometric (body weight, height, BMI, waist, and hip circumference) and physiological measurements (temperature, systolic and diastolic blood pressure, heart rate and oxygen saturation) were collected on day 1 (D1) and day 30 (D30), as reported in detail.14 Participants were instructed to use MyFitnessPal (https://www.myfitnesspal.com, accessed between January 2020 and August 2021) to record their daily food and beverage intake. Nutrient intake (calories, carbohydrates, fat, protein, fiber, and cholesterol) was evaluated using 15-day dietary records collected during the 30-day intervention. A detailed description of data collection and analysis was previously reported.14 Briefly, thirteen dietary components based on servings per 1000 kcal, were used to estimate Healthy Eating Index (HEI) scores with values of 0 and 100 indicating the lowest and highest adherence to the Dietary Guidelines for Americans (DGA), respectively.16
2.4 Stool sample collection
Stool samples were collected on D1 and D30 in polypropylene tubes with spoon and screwcaps (Sarstedt; Newton, NC) containing 3 mL DNA/RNA Shield (Zymo Research; Irvine, CA). Each participant was provided with stool collection kits (plastic stool collection hats, collection tubes, disposable gloves, biohazard bags, and written instructions), and instructed to avoid the use of laxatives, stool softeners and antacids within 48 h before stool collection. Participants were asked to aliquot samples into 2 tubes (approximately 2 g per tube), and to bring them to our facility within 2 h of collection or to freeze them if collected a day prior until transported to our facility. Stool weights were measured immediately upon receiving the samples, followed by storage at −80 °C for later use in DNA and RNA extraction. Meals low in fiber and polyphenols were provided to participants for 2 days before each stool collection to minimize variability due to diet.
2.5 Fecal DNA extraction and 16S rRNA sequencing
Each tube containing the stool sample in DNA/RNA shield was sub-aliquoted in our laboratory before DNA and RNA extractions. Stool samples were homogenized by adding three units of 6 mm solid-glass beads (Propper; Long Island City, NY) to collection tubes and vortexed on an analog vortex mixer (Fisher Scientific; Waltham, MA) on setting 9 for 30 seconds to aliquot into at least two Eppendorf tubes to be used for future analyses.17 DNA was extracted from an aliquot of stool slurry using the Quick-DNA™ Fecal/Soil Microbe Miniprep Kit (Zymo Research; Irvine, CA). Briefly, approximately 150 mg of fecal slurry was lysed by bead beating in ultra-high density ZR BashingBead lysis tube™ provided in the kit using a Bead Genie™ fitted with a 2 mL tube holder assembly (Scientific Industries INC; Bohemia, NY) at maximum speed for 2 min, followed by centrifugation at 10
000g for 1 min. Supernatant was then used to extract DNA following the manufacturer's protocol. DNA concentration and purity at 260/280 nm were assessed using a Nanodrop 2000 spectrophotometer (Thermo Scientific; Rockford, IL). DNA extracted from fecal samples was used to amplify a fragment (approximately 500 bp) of the 16S ribosomal RNA (16S rRNA) gene using the primers 341F (5′_CCTACGGGNGGCWGCAG_3′) and 805R (5′_GACTACHVGGGTATCTAATCC_3′) for further high throughput 16S sequencing at MilliporeSigma (Saint Louis, MO) using the MiSeq instrument (Illumina). The workflow used in this study followed Illumina's “16S metagenomic sequencing library preparation” protocol for library preparation and qualification steps prior to sequencing. The protocol involved using primer pairs for the V3–V4 regions to create a single amplicon of approximately 500 bp. The MiSeq Reagent Kit V2, with paired-end sequencing parameters (2 × 250 base reads, 500 cycles), was used for sequencing. The sequencing qualifications adhered to Illumina's MiSeq Sequencing Systems specifications, which included metrics such as output, reads passing filter, supported cluster density, Q30 Quality Scores, and alignment to the spiked PhiX control. Each sequencing run had to meet these guidelines before the data was analyzed in the Microbiome Computational Analysis for Multiomic Profiling (M-CAMP™) Cloud Platform.18 The classification approach on the M-CAMP™ platform consisted of two steps, a pre-processing stage that removed low quality reads and PCR artifacts which can lead to false positive classifications, and a classification stage that used alignments to the optimized M-CAMP 16S V3–V4 database to achieve high precision species levels calls.18 Sequencing data and associated metadata were uploaded into the NCBI (PRJNA859373) BioProject ID: 859373.
2.6 Intestinal barrier function (IBF) biomarkers
Metabolic endotoxemia was evaluated by measuring lipopolysaccharide-binding protein (LBP) levels in fasting plasma samples collected on D1 and D30 using the RayBio ® Human LBP ELISA kit (RayBiotech Inc.; Norcross, GA) according to the manufacturer's protocol. Intestinal permeability was assessed by the lactulose/mannitol (L/M) ratio as reported previously with some modifications.19 Briefly, eligible participants were instructed to avoid taking non-steroidal anti-inflammatory drugs (NSAIDs) for at least a week prior to the test, to refrain from artificial sweeteners 24 h prior the test, and to fast overnight (∼12 h). Participants were asked to empty their bladder (blank urine sample collected) before drinking 200 mL of a sugary solution containing 7.5 g lactulose and 2 g mannitol. The blank urine sample collected before drinking the sugary solution was used to correct for the endogenous presence of lactulose and mannitol. Participants were asked to collect their urine output within 5 h after drinking the sugary solution in a plastic container with added antimicrobial agents (0.1 mL of 1% chlorhexidine aqueous solution, or 10% thymol). Antimicrobial agents were also added to the blank urine sample to match concentrations in 5 h urine output. Participants were encouraged to drink at least 750 mL of non-carbonated water and to avoid eating during the first 2 h of urine collection. Then, participants returned to their regular diet with restrictions on dairy products, fruit juices, soft drinks, and foods with high fructose corn syrup and/or natural or artificial sweeteners. The total 5 h urine output was recorded, aliquoted, and stored at −20 °C until analysis by UPLC-MS/MS as reported.20 Briefly, 2.5 mL of urine sample was mixed with 2.5 mL of deionized water in a 15 mL Falcon tube containing 500 mg amberlite MB150 ion-exchange resin (Sigma Aldrich; Saint Louis, MO) to adsorb sodium ions. Samples were centrifuged at 10
000g for 5 min after stirring for 1 min followed by filtration through a 0.2 μm Acrodisc syringe filter with PTFE membrane (13 mm) (Pall Corporation; Washington, NY). Mannitol and lactulose (Sigma Aldrich; Saint Louis, MO) were used as standards dissolved in ACN/H2O (75
:
25). Sugar analysis was performed using an Ultimate3000 UPLC equipped to a TSQ AltisTM triple quadrupole mass spectrometer (Thermo Fisher Scientific; Waltham, MA). The chromatographic separation was conducted with a XBridgeTM HILIC (4.6 × 150 mm × 3.5 μM) column (Waters Corporation; Milford, MA) with 75% ACN/25% 5 mM of NH4Ac in H2O to 40% ACN/60% 5 mM of NH4Ac in H2O in 10 min. The flow rate was 500 μL min−1.20 Mass spectrometer was run in the negative ionization mode and parameters consisted of a 2300 V spray voltage, a sheath gas of 20, auxiliary gas of 4, and a sweep gas of 1, ion transfer tube temperature set at 350 °C and vaporizer temperature set at 100 °C. Scheduled selective reaction (SSR) monitoring was used to identify and quantify each sugar based on optimized conditions and transitions of their respective standards. SSR conditions are presented in ESI Table S1.† All data were collected and analyzed using Chromeleon 7.2.10 ES software (Thermo Fisher Scientific; Waltham, MA). The percentage of lactulose (L) and mannitol (M) recovery was calculated as previously reported.21
Fecal mRNA levels of regeneration islet-derived protein 4 (REG4), and interleukin-22 (IL-22) have been linked to gut inflammatory conditions.22 Briefly, mRNA was isolated from fecal samples collected on D1 and D30 using the ZymoBIOMICS RNA Miniprep Kit (Zymo Research; Irvine, CA) according to the manufacturer's protocol. The quality and quantity of extracted fecal mRNA were evaluated using the NanoDrop ND-1000 Spectrophotometer (NanoDrop Technologies; Wilmington, DE). Extracted mRNA was used to synthesize cDNA using the iScript reverse transcription supermix (BioRad; Hercules, CA). Primers were purchased from Life Technologies (Carlsbad, CA) with sequences presented in ESI Table S2.† Real-time polymerase chain reaction (RT-PCR) was performed using a CFX384 touch real time PCR detection system (BioRad; Hercules, CA). Each reaction was performed in duplicate and mRNA levels were calculated by the comparative CT method using 18S as a housekeeping gene.23
2.7 Statistical analysis
The M-CAMP™ cloud-based web platform was used to generate data for comparative analysis.18 Clade fragment percentage values representing read counts were used for normalization and calculation of relative abundances.18 Alpha (α) diversity was estimated through the Shannon, Simpson, number of observed_OTUS and Chao1 metrics using a rarefaction depth of 63
659 sequences per sample. The statistical analysis of alpha diversity between cherry and placebo treatments was performed by Kruskal–Wallis test. Beta (β) diversity was estimated by weighted and unweighted Unifrac, as well as the Jaccard and Bray Curtis dissimilarity indexes. The statistical significance of β-diversity within cherry and placebo groups was determined with permutational multivariate analysis of variance (PERMANOVA).
Relative abundances of fecal bacteria were analyzed using non-parametric statistical tests with Graphpad Prism Software (Version 10.2.2, La Jolla, CA). The relative abundance data are presented as mean with 95% confidence interval (95% CI). The Wilcoxon matched pairs signed rank test was performed to detect differences in relative abundance data at different taxonomic levels within each experimental group. The Mann–Whitney test was used to assess differences between cherry and placebo groups at same time points. Only bacteria taxa detected in at least 50% of samples and with relative abundance ≥0.05% were considered for analyses. Bacterial data at genus and species levels that showed statistical significance (p < 0.05) between treatments were stratified by BMI (low: 30–34, high: 35–40) and gender (male and female). Data was also stratified by HEI values based on median values (low: 24–42 and high: 43–60) where a low HEI indicates poor adherence to healthy dietary guidelines, while a high HEI suggests the opposite, reflecting a healthy dietary pattern. When differences between cherry and placebo were significant at D1, the difference between D30 and D1 or delta (Δ) values were computed in each group to determine marginal means while controlling for D1 as a significant covariate using SPSS 26.0 (IBM® SPSS® Statistics, Armonk, NY). Statistical differences between marginal means were determined using unpaired t test with Welch correction. Data statistically significant (p < 0.05) was stratified by BMI, gender, and HEI.
Data from metabolic endotoxemia and intestinal permeability biomarkers did not follow normal distribution, thus, the Wilcoxon matched pairs signed rank test was used to detect differences within cherry and placebo groups. The Mann–Whitney test was used to assess differences between treatments. Spearman correlation analyses were performed in RStudio version 1.4.1717 to examine associations between changes in the gut microbiota and obesity-related biomarkers measured and published previously following DSC supplementation.14
3. Results and discussion
3.1 Participant characteristics, anthropometric and physiological assessments, and nutritional patterns
Participants (n = 60) were enrolled in the study and randomly allocated into placebo (n = 30) and cherry groups (n = 30) as previously reported.14 However, only forty participants (n = 19 cherry, 11 females and 8 males; n = 21 placebo, 14 females and 7 males) were able to complete the study.14 The anthropometric and physiological measurements, as well as nutrient intake and the HEI results were previously reported in detail and can be accessed at https://www.mdpi.com/2072-6643/15/3/681. Briefly, there were no significant differences between cherry and placebo groups for anthropometric and physiological measurements. However, participants in the cherry group had lower systolic and diastolic blood pressure compared to those in placebo at D30.14 Similarly. the analysis of 15-day dietary records showed no significant differences in nutrient intake and HEI scores between study groups.14
3.2 Effect of DSC supplementation on the gut microbiota composition
Fecal samples at D1 and D30 were successfully collected and analyzed for 40 obese subjects. The number of sequences per sample varied from 63
659 (lowest) to 379
614 (highest). Firmicutes, Bacteroidetes, Actinobacteria and Proteobacteria were the most abundant phyla (∼99% of relative abundance). Each phylum, such as Firmicutes or Bacteroidetes, encompasses a wide variety of bacterial species with diverse functions. Some members might confer health benefits, while others may be harmful. Additionally, different bacterial species, even across phyla, can perform similar functions. This functional redundancy means that the overall metabolic and health impact may not directly correlate with changes in the relative abundance of specific phyla. Therefore, drawing conclusions based solely on phylum-level changes can be challenging and sometimes misleading.
Verrucomicrobia phylum was detected in less than 50% of samples with relative abundance of <1% (4 in cherry and 6 in placebo). However, Verrucomicrobia was included in the analyses due to its relevance in intestinal health and its role as an interface between gut microbiome and host tissues. One of the most studied members of this phylum is Akkermansia muciniphila, a mucin-degrading bacterium that resides in the mucus layer of the intestines. It plays a crucial role in maintaining gut barrier integrity, thereby preventing gut dysbiosis and leaky gut, which are associated with obesity and chronic diseases.24 Moreover, a reduced abundance or absence of this commensal bacterium has been linked to conditions such as obesity, diabetes, liver steatosis, inflammation, and responsiveness to cancer.25
The 16S sequencing analyses showed no significant differences between the cherry and placebo groups on D1 regarding the relative abundance of the main phyla Bacteroides, Firmicutes, and Proteobacteria (Table 1 and Fig. 1A). However, the relative abundance of Firmicutes and Bacteroides changed significantly at D30 compared to D1 values in placebo group (p = 0.04 for both phyla). The relative abundances of Actinobacteria were similar at D1 between cherry and placebo but decreased in placebo at D30 compared to D1 (p = 0.01) and reached significance compared to the cherry group at D30 (p = 0.03) (Table 1 and Fig. 1A). Relative abundances and statistical comparisons between cherry and placebo and within each group, organized by phylum at different taxa levels are presented in Table 2. Lachnospiraceae (Firmicutes phylum), Bacteroidaceae (Bacteroidetes phylum), Bifidobacteriaceae (Actinobacteria phylum) and Desulfovibrionaceae (Proteobacteria phylum) represented the most predominant bacterial families in cherry and placebo groups (Table 2 and Fig. 1B).
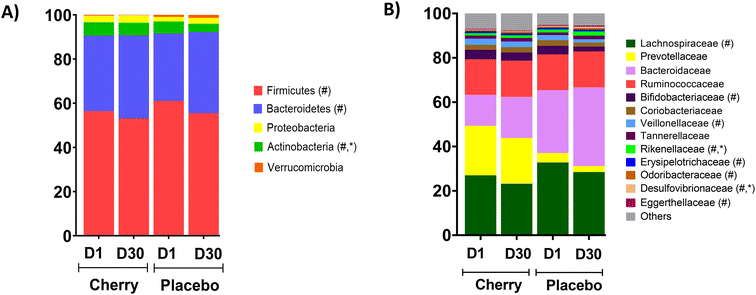 |
| Fig. 1 Relative abundance of bacteria before and after intervention in cherry and placebo groups. (A) Stacked column bar depicts the average relative abundances and distribution of the most highly abundant taxa at the (A) phylum and (B) family level. (*) Indicates significant differences between cherry and placebo at D30, (#) indicates significant differences within placebo group. The Mann–Whitney test was assessed to evaluate differences between cherry and placebo treatments. The Wilcoxon matched-pairs rank test was performed to compare values within treatments. A p value < 0.05 was considered statistically significant. Others: Sutterellaceae, Lactobacillaceae, Streptococcaceae, Eubacteriaceae, Peptostreptococcaceae, Clostridiaceae, Enterobacteriaceae and Oscillospiraceae. | |
Table 1 Mean relative abundance (percentage of 16S sequences) for the 5 more abundant phyla in cherry and placebo groups
Phylum |
Cherry (n = 19) |
Placebo (n = 21) |
Sliced by treatment and/or day |
D1 |
D30 |
D1 |
D30 |
Relative abundances in each group at different time points were analyzed by Wilcoxon matched pairs signed tank test, while the Mann Whitney test was used to compare cherry vs. placebo at same time point. Different letters indicate significant difference between treatments (A, B) and/or day (X, Y) at p value < 0.05. |
Firmicutes |
56.41 (50.47, 62.35) |
53.10 (46.17, 60.03) |
61.06 (55.85, 66.27)A |
55.49 (50.72, 60.26)B |
Placebo (p = 0.04) ↓D30 |
Bacteroidetes |
34.24 (27.30, 41.17) |
37.68 (30.84, 44.52) |
30.48 (25.32, 35.65)A |
36.73 (31.55, 41.91)B |
Placebo (p = 0.04) ↑D30 |
Actinobacteria |
5.99 (4.03, 7.95) |
5.62 (3.22, 8.01)X |
5.44 (3.07, 7.80)A |
3.76 (1.90, 5.63)B, Y |
Placebo (p = 0.01) ↓D30 cherry vs. placebo D30 (p = 0.03) |
Proteobacteria |
2.96 (1.54, 4.38) |
3.42 (1.76, 5.08) |
2.06 (1.27, 2.85) |
2.63 (1.87, 3.38) |
n.s |
Verrucomicrobia |
1.85 (0.26, 3.44) n = 4 |
0.74 (−0.71, 2.21) n = 4 |
2.18 (−0.21, 4.58) n = 9 |
3.17 (0.28, 6.07) n = 9 |
n.s |
Table 2 Mean relative abundance of fecal bacteria modulated at different taxa levels in cherry and placebo groups
Taxa |
Cherry (n = 19) |
Placebo (n = 21) |
Sliced by treatment and/or day |
D1 |
D30 |
D1 |
D30 |
Values are mean (95% CI). Statistically significant results (p < 0.05) are represented by letters A, B (indicating significant difference within treatment), and letters X, Y (indicating significant difference between cherry and placebo groups at same time point). The taxonomic lineage of each taxon is f: family, g: genus, s: species. The Wilcoxon matched-pairs rank test was performed to compare values within treatment. The Mann–Whitney test was used to compare cherry and placebo groups at the same time point. |
Phylum Firmicutes
|
f_Lachnospiraceae
|
26.98 (22.20, 31.76) |
23.21 (19.69, 26.72) |
32.59 (27.63, 37.55) A |
28.01 (24.35, 31.67)B |
Placebo (p = 0.03) ↓D30 |
f_Lachnospiraceae; g_Anaerostipes |
4.11 (2.84, 5.39)A,X |
1.12 (0.68, 1.56)B |
2.21 (1.51, 2.90)A,Y |
1.77 (1.24, 2.29)B |
Cherry (p < 0.0001) ↓D30 Placebo (p = 0.03) ↓D30 cherry vs. placebo D1 (p = 0.02) |
f_Lachnospiraceae; g_Anaerostipes; s_A. hadrus |
6.10 (4.33, 7.87)A,X |
1.81 (1.03, 2.60)B |
2.55 (1.78, 3.32)A,Y |
2.27 (1.55, 3.00)B |
Cherry (p < 0.0001) ↓D30 cherry vs. placebo: D1 (p = 0.0008) |
f_Lachnospiraceae; g_Blautia |
10.71 (8.26, 13.17)X |
9.12 (7.40, 10.84)X |
15.61 (12.67, 18.55)Y |
13.74 (11.29, 16.19)Y |
Cherry vs. placebo D1 (p = 0.01); cherry vs. placebo D30 (p = 0.004) |
f_Lachnospiraceae; g_Blautia; s_B. luti |
3.99 (2.56, 5.42) n = 18 |
3.17 (2.02, 4.31) n = 18 |
4.37 (2.95, 5.79)An = 20 |
3.09 (1.84, 4.35)Bn = 20 |
Placebo (p = 0.04) ↓D30 |
f_Lachnospiraceae; g_Fusicatenibacter; s_F. saccharivorans |
4.46 (3.03, 5.89)A |
3.43 (2.41, 4.45)B |
3.47 (2.23, 4.71)A n = 20 |
2.56 (1.39, 3.74)B n = 20 |
Cherry (p = 0.01) ↓D30 Placebo (p = 0.01) ↓D30 |
f_Lachnospiraceae; g_Roseburia; s_R. intestinalis |
0.99 (0.42, 1.57)A, Xn = 13 |
2.80 (1.25, 4.34)Bn = 13 |
2.94 (1.65, 4.22)Yn = 10 |
1.90 (1.22, 2.59) n = 10 |
Cherry (p = 0.01) ↑D30 cherry vs. placebo D1 (p = 0.002) |
f_Veillonellaceae
|
3.11 (1.62, 4.60) n = 17 |
2.81 (1.36, 4.26) n = 17 |
3.06 (0.78, 5.34)An = 15 |
2.00 (0.61, 3.39)Bn = 15 |
Placebo (p = 0.02) ↓D30 |
f_ Ruminococcaceae; g_ Agathobaculum |
0.39 (0.19, 0.59) n = 16 |
0.44 (0.14, 0.75) n = 16 |
0.41 (0.29, 0.52)An = 19 |
0.30 (0.21, 0.39)Bn = 19 |
Placebo (p = 0.05) ↓D30 |
f_Ruminococcaceae; s_C. leptum |
0.54 (0.19, 0.89) n = 10 |
2.22 (−0.49, 4.95)Xn = 10 |
0.38 (0.06, 0.70) n = 12 |
0.40 (0.12, 0.68)Yn = 12 |
Cherry vs. placebo D30 (p = 0.03) |
f_Erysipelotrichaceae
|
0.60 (0.14, 1.06) |
0.84 (0.38, 1.31) |
0.55 (0.24, 0.85)A |
0.85 (0.31, 1.39)B |
Placebo (p = 0.01) ↑D30 |
f_Erysipelotrichacea; g_Turicibacter |
0.16 (0.04, 0.27)An = 10 |
0.58 (0.10, 1.05)Bn = 10 |
0.35 (0.12, 0.58) n = 12 |
0.54 (0.17, 0.91) n = 12 |
Cherry (p = 0.01) ↑D30 |
Phylum Bacteroidetes
|
f_Bacteroidaceae
|
13.97 (7.21, 20.74)A, X |
18.57 (11.02, 26.11)B, X |
27.87 (23.03, 32.71)A, Y |
35.22 (29.63, 40.80)B, Y |
Cherry (p = 0.04) ↑D30 Placebo (p = 0.007) ↑D30 Cherry vs. placebo D1 (p = 0.001); Cherry vs. placebo D30 (p = 0.0003) |
f_Bacteroidaceae; g_Bacteroides; s_B. vulgatus |
9.43 (4.39, 14.47)An = 17 |
15.53 (7.86, 23.21)Bn = 17 |
14.08 (10.07, 18.09) n = 19 |
16.20 (11.37, 21.04) n = 19 |
Cherry (p = 0.003) ↑D30 |
f_Bacteroidaceae; g_Bacteroides; s_B. thetaiotaomicron |
0.45 (0.06, 0.84)Xn = 14 |
0.70 (0.36, 1.04) n = 14 |
2.40 (−0.08, 4.89)Y |
3.04 (−0.15, 6.24) |
Cherry vs. placebo D1 (p = 0.01) |
f_Bacteroidaceae; g_Bacteroides; s_B. ovatus |
1.92 (0.86, 2.98)X |
2.24 (1.24, 3.24) |
3.79 (2.25, 5.33)Yn = 20 |
4.83 (2.27, 7.39) n = 20 |
Cherry vs. placebo D1 (p = 0.03); cherry vs. placebo D30 (p = 0.02) |
f_Tannerellaceae
|
1.46 (−0.07, 2.99) |
1.32 (0.47, 2.17)X |
1.35 (0.83, 1.88) n = 20 |
1.68 (1.27, 2.09)Yn = 20 |
Cherry vs. placebo D30 (p = 0.02) |
f_Tannerellaceae; g_Parabacteroides |
1.78 (−0.07, 3.61) n = 18 |
1.57 (0.53, 2.62)Xn = 18 |
1.47 (0.93, 2.01) n = 20 |
1.78 (1.37, 2.20)Yn = 20 |
Cherry vs. placebo D30 (p = 0.05) |
f_Rikenellaceae
|
1.17 (0.49, 1.85) n = 17 |
1.23 (0.39, 2.07) Xn = 17 |
1.74 (0.89, 2.59)An = 19 |
2.61 (1.33, 3.88)B, Yn = 19 |
Placebo (p = 0.004) ↑D30 cherry vs. placebo D30 (p = 0.01) |
f_Rikenellaceae; g_Alistipes |
1.03 (0.41, 1.65) n = 17 |
1.12 (0.29, 1.95)Xn = 17 |
1.97 (1.02, 2.92)An = 19 |
2.79 (1.49, 4.09)B, Yn = 19 |
Placebo (p = 0.005) ↑D30; cherry vs. placebo D30 (p = 0.004) |
f_Rikenellaceae; g_Alistipes; s_A. shahii |
0.31 (0.10, 0.51) n = 12 |
0.24 (0.08, 0.40)Xn = 12 |
0.26 (0.07, 0.45)An = 13 |
0.57 (0.20, 0.94)B, Yn = 13 |
Placebo (p = 0.0002) ↑D30 cherry vs. placebo D30 (p = 0.005) |
f_Rikenellaceae; g_Alistipes; s_A. finegoldii |
0.41 (−0.08, 0.91) n = 10 |
0.36 (−0.02, 0.74) n = 10 |
0.47 (−0.11, 1.07)An = 11 |
1.00 (−0.03, 2.04)Bn = 11 |
Placebo (p = 0.003) ↑D30 |
f_Odoribacteraceae
|
0.39 (0.17, 0.60) n = 13 |
0.58 (0.26, 0.90) n = 13 |
0.55 (0.26, 0.84)An = 16 |
0.79 (0.53, 1.06)Bn = 16 |
Placebo (p = 0.03) ↑D30 |
f_Odoribacteraceae; g_Odoribacter |
0.33 (0.15, 0.50) n = 12 |
0.47 (0.13, 0.81) n = 12 |
0.42 (0.18, 0.65)An = 15 |
0.56 (0.34, 0.77)Bn = 15 |
Placebo (p = 0.02) ↑D30 |
f_Odoribacteraceae; g_Odoribacter; s_O. splanchnicus |
0.44 (0.23, 0.65) n = 12 |
0.63 (0.23, 1.04) n = 12 |
0.50 (0.23, 0.77)An = 14 |
0.72 (0.48, 0.97)Bn = 14 |
Placebo (p = 0.006) ↑D30 |
Phylum Actinobacteria
|
f_Bifidobacteriaceae
|
4.25 (2.17, 6.33) |
3.72 (1.48, 5.95) |
4.18 (2.07, 6.29)An = 19 |
2.60 (1.10, 4.09)Bn = 19 |
Placebo (p = 0.02) ↓D30 |
f_Bifidobacteriaceae; g_Bifidobacterium |
4.62 (2.42, 6.82) |
3.97 (1.66, 6.28) |
4.67 (2.37, 6.98)An = 19 |
2.79 (1.17, 4.42)Bn = 19 |
Placebo (p = 0.007) ↓D30 |
f_Coriobacteriaceae; g_Collinsella |
2.50 (1.71, 3.29) n = 18 |
2.58 (1.58, 3.59) n = 18 |
3.64 (2.13, 5.15)An = 15 |
3.00 (1.35, 4.65)Bn = 15 |
Placebo (p = 0.03) ↓D30 |
f_Eggerthellaceae
|
0.21 (0.11, 0.31) n = 18 |
0.17 (0.12, 0.22) n = 18 |
0.20 (0.13, 0.28)An = 20 |
0.32 (0.12, 0.52)Bn = 20 |
Placebo (p = 0.04) ↑ D30 |
Phylum Proteobacteria
|
f_Desulfovibrionaceae
|
0.26 (0.15, 0.37) n = 18 |
0.39 (0.22, 0.55)Xn = 18 |
0.43 (0.25,0.60) An = 18 |
0.69 (0.42, 0.97)Y, Bn = 18 |
Placebo (p = 0.03) ↑ D30; cherry vs. placebo D30 (p = 0.01) |
f_Desulfovibrionaceae: g_Bilophila |
0.23 (0.12, 0.34) n = 18 |
0.29 (0.17, 0.42)Xn = 18 |
0.43 (0.23, 0.62)An = 18 |
0.67 (0.40, 0.95)B, Yn = 18 |
Cherry vs. placebo D30 (p = 0.01); placebo (p = 0.04) ↑ D30 |
f_Desulfovibrionace: g_Bilophila; s_B. wadsworthia |
0.39 (0.21, 0.56) n = 15 |
0.55 (0.31, 0.79) n = 15 |
0.51 (0.28, 0.74)An = 19 |
0.93 (0.52, 1.33)Bn = 19 |
Placebo (p = 0.01) ↑ D30 |
3.2.1 DSC supplementation prevented the increase in the abundance of inflammatory bacteria in obese adults.
Lachnospiraceae, which are known producers of SCFAs and facilitate the colonization resistance against intestinal pathogens, decreased in placebo at D30 vs. D1 (p = 0.03) (Table 2). Although the relative abundances of Lachnospiraceae were similar between D1 and D30 for the cherry group, significant changes were found at lower taxonomic levels. The Anaerostipes genus and Anaerostipes hadrus species decreased at D30 vs. D1 in the cherry group (Table 2). Moreover, considering that Anaerostipes was different between cherry and placebo groups at D1; the Δ values were computed to evaluate differences between treatments. Results showed a highly significant reduction in Δ Anaerostipes (p < 0.001) in the cherry group compared to placebo (Fig. 2A and ESI Table S3†) and a similar pattern was observed in Δ A. hadrus (p = 0.02) (Fig. 2B and ESI Table S3†). However, relative abundances decreases do not necessarily mean decreases in absolute abundance. Stratified analyses showed statistical differences were more significant for high BMI (p = 0.004), female (p = 0.003) and high HEI (p = 0.004) participants (Fig. 2C, D and E, respectively). Our results are consistent with a clinical study reporting a significant decrease of Anaerostipes in overweight and obese subjects after the consumption of a pomegranate extract (656 mg phenolics) for 3 weeks.26 This bacterial genus plays a vital role in maintaining gut health through the production of beneficial SCFAs. Its presence in the gut has been associated with improved gut barrier integrity, anti-inflammatory effects, and better metabolic health. Specifically, A. hadrus is known for its crucial role in butyrate production, which has been linked to enhanced gut barrier integrity, anti-inflammatory effects, and improved metabolic health.27 However, a study showed that the introduction of A. hadrus in mice treated with dextran sulfate sodium (DSS) exacerbated colitis and significantly elevated LBP levels.28 Therefore, even though A. hadrus generally promotes anti-inflammatory responses and supports gut barrier integrity, its presence might contribute to inflammation in dysbiosis conditions or in combination with other pro-inflammatory factors. Similarly, Δ Blautia (Lachnospiraceae family) showed a significant decrease in cherry compared to the placebo group (p = 0.04) (Fig. 3A and ESI Table S3†). The difference was significant in high BMI (p = 0.006) and equally significant only in male participants (p = 0.04) (Fig. 3B and C, respectively). The Blautia genus, known for its crucial role in maintaining gut health through the production of SCFAs, enhancement of gut barrier integrity, and modulation of immune responses, may have context-dependent roles. Some studies indicate a higher prevalence of Blautia in patients with type 2 diabetes (T2D)29 and obesity30 and a positive correlation with inflammatory cytokines (IL-1β, IL-6 and TNF-α) in a T2D-rat model.31 Under certain conditions, even typically beneficial bacteria can contribute to pro-inflammatory states due to the complex and context-dependent interactions between different microbial species and the host. In cases of dysbiosis, where the overall microbial balance is disrupted, the presence of butyrate-producing bacteria might be associated with inflammatory responses. This is because in an environment with prevalent pro-inflammatory metabolites, the net effect might be pro-inflammatory rather than beneficial. Therefore, further research is needed to understand the implications of DSC dietary supplementation and the decreased abundance of Blautia and Anaerostipes.
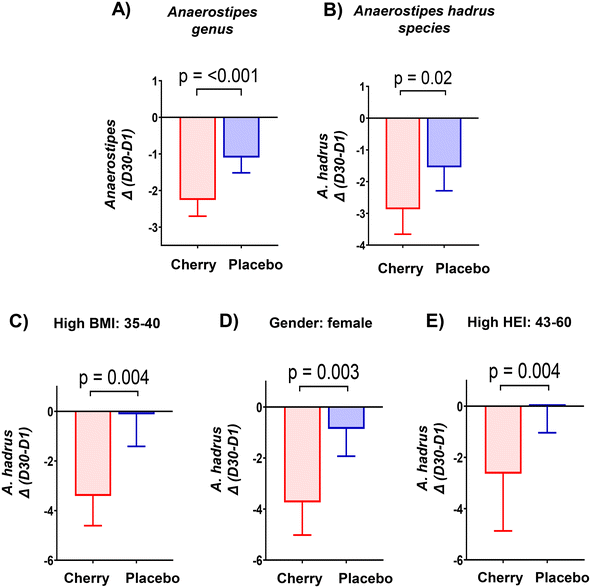 |
| Fig. 2 DSC supplementation decreased the abundance of Anaerostipes genus and Anaerostipes hadrus species. (A) Δ Anaerostipes, (B) Δ A. hadrus, (C) Δ A. hadrus stratified by high BMI (35–40), (D) gender (female) and (E) high HEI (43–60). Data are estimated marginal means (95% CI) obtained after adjustment for significant D1 values. Statistical differences between marginal means were determined using unpaired t test with Welch correction. A p value < 0.05 was considered statistically significant. Box plots show lower quartile, median, upper quartile, and Tukey whiskers. | |
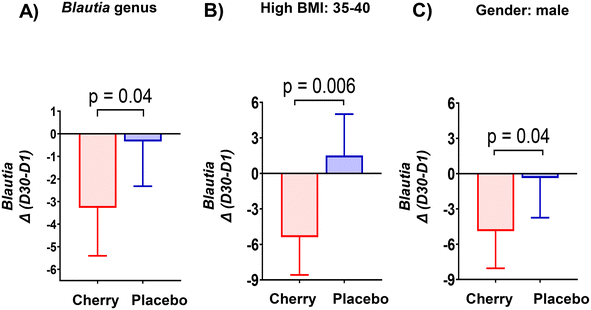 |
| Fig. 3 DSC supplementation decreased the abundance of Blautia genus. (A) Δ Blautia, (B) Δ Blautia stratified by high BMI (35–40) and (C) gender (male). Data are estimated marginal means (95% CI) obtained after adjustment for significant D1 values. Statistical differences between marginal means were determined using unpaired t test with Welch correction. A p value < 0.05 was considered statistically significant. Box plots show lower quartile, median, upper quartile, and Tukey whiskers. | |
Rikenellaceae family (Bacteroidetes phylum) abundance increased significantly in placebo (p = 0.004) at D30 vs. D1 and was higher than cherry at D30 (p = 0.01), even though levels in both groups were similar at D1 (Table 2). The increase in Rikenellaceae in the placebo group may be relevant because of its link to obesity and type 2 diabetes.32 In addition, significant microbiota changes found in placebo might be attributed to natural fluctuations due to various factors such as diet, lifestyle, and environmental factors. At a lower taxonomic level, Alistipes genus and Alistipes shahii species presented a similar pattern with a notable increase in the relative abundance in placebo at D30 as compared to D1 and to cherry group at D30 (Table 2 and Fig. 4A, B, respectively). Significant differences in A. shahii between placebo and cherry at D30 were attributed to the differences in females only (p = 0.03) (Fig. 4C). A. finegoldii also increased in placebo (p = 0.003) at D30 vs. D1, and was higher than the cherry group at D30, although the difference did not reach significance due to high variability (Table 2 and Fig. 4D). A shahii, has shown pro-inflammatory activity by TLR-4-priming/TNF production that may increase local inflammation in cancer treatment,33 while A. finegoldii has been reported as a potential driver for gut barrier dysfunction and inflammation in patients with elevated blood pressure.33 Thus, the maintenance of A. shahii and A, finegoldii levels in the cherry group may suggest that DSC helped obese subjects to mitigate the abundance of pro-inflammatory bacteria. However, these bacterial species have also shown protective roles in liver fibrosis, cardiovascular diseases, and colitis.33 Additional research is warranted to explore the significance of DSC modulation on A. shahii and A. finegoldii within obese adults.
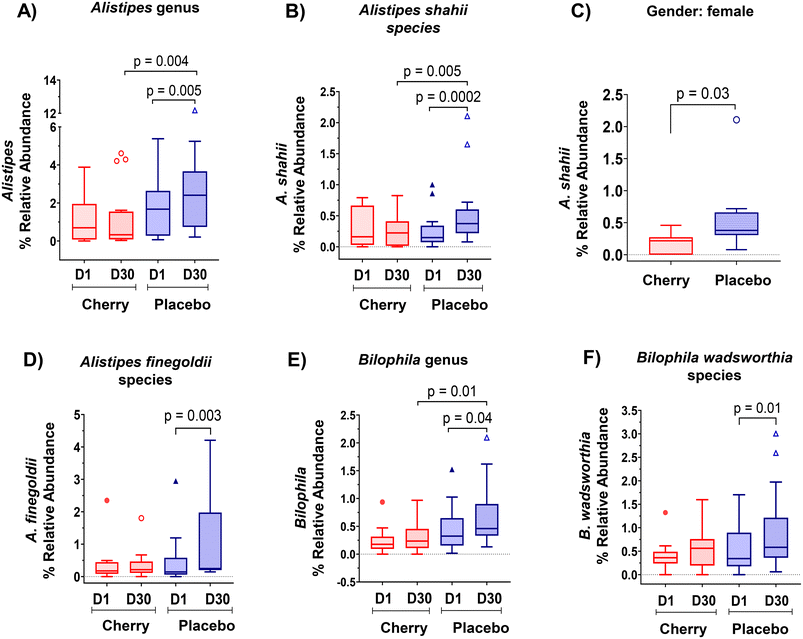 |
| Fig. 4 DSC supplementation prevented the increase in the abundance of inflammatory bacteria in obese adults. (A) Alistipes, (B) A. shahii, (C) A. shahii stratified by gender: female, (D) A. finegoldii, (E) Bilophila and (F) B. wadsworthia. The Wilcoxon matched-pairs rank test was performed to compare values within treatments. The Mann–Whitney test was assessed to evaluate differences between cherry and placebo treatments. A p value < 0.05 was considered statistically significant. Box plots show lower quartile, median, upper quartile, and Tukey whiskers. | |
Members of the proteobacteria phylum are known to induce and sustain a pro-inflammatory environment causing the impairment of the intestinal barrier function.34 Interestingly, results revealed a significant increase in the abundance of Desulfovibrionaceae family in the placebo group at D30 vs. D1 (p = 0.03) and a higher abundance at D30 compared to the cherry group at the same time point (p = 0.01) (Table 2). A similar pattern was found at lower taxa for Bilophila genus (Table 2 and Fig. 4E). Notably, within this taxa group, a significant increase in the B. wadsworthia species was observed only in the placebo group at D30 compared to D1 (Fig. 4F). The increase in B. wadsworthia in placebo is of particular interest because research suggests a higher abundance of Bilophila, particularly B. wadsworthia, in obese individuals. This increase may be linked to dietary habits and metabolic changes associated with obesity. High-fat diets, especially those rich in saturated fats, have been found to promote the growth of Bilophila, and such diets are common among obese individuals. B. wadsworthia thrives in environments rich in bile acids, which are more prevalent in high-fat diets. An increase in Bilophila, through its production of harmful metabolites, may compromise gut barrier function, allowing endotoxins to enter the bloodstream and promote inflammation, which is often observed in obesity.35,36 This was supported by a study demonstrating that B. wadsworthia aggravates high fat diet induced metabolic dysfunctions and was associated with intestinal inflammation and gut barrier dysfunction.37 In contrast, phytochemicals in plant-based foods have specific and possibly prebiotic effects on the microbiome. Bioactive compounds in DSC, include phenolics that have been proved to correlate with the antioxidant capacity of the fruit pulp extracts,38 especially anthocyanins, have been widely reported for beneficial effects in microbiota composition. A study reported that anthocyanins extracted from dehydrated blackberries and strawberries were effective in reducing populations of the pro-inflammatory B. wadsworthia in rats challenged with 2 doses of azoxymethane (10 mg kg−1) and two treatments with DSS.39 Moreover, a recent meta-analysis highlighted the supplementation of anthocyanins as an effective strategy to modulate the intestinal microbiota with subsequent improvements in intestinal barrier function and reduced potential risk of inflammation.40 Therefore, results from this study suggest that anthocyanins in DSC might prevent an increase in the abundance of inflammatory bacteria in individuals with obesity.
3.2.2 DSC supplementation promoted the growth of bacteria that support a healthy gut environment.
Roseburia genus showed a trend to increase in the cherry group (data not shown). Moreover, at species level, results from Δ R. intestinalis, showed no significant differences between cherry and placebo groups (ESI Table S3†). However, R. intestinalis species, a butyrate-producing bacterium known for its preventive effect on intestinal inflammation,41 notably increased in the cherry group at D30 (p = 0.01) (Table 2 and Fig. 5A). These findings align with those of Xian et al.,42 who reported a significant increase in Roseburia in an animal model of diet-induced obesity after the intake of raspberry polyphenolic extracts for 16 weeks. The increase in R. intestinalis after DSC supplementation is relevant because this bacterium is markedly decreased in obese adults.43 Therefore, DSC polyphenols (phenolic acids and anthocyanins) likely played a role in enhancing the prevalence of R. intestinalis within the cherry group because these compounds might exhibit prebiotic-like functions that selectively foster the growth of beneficial bacteria.44 These results imply potential intestinal health-promoting effects of DSC in obese individuals.
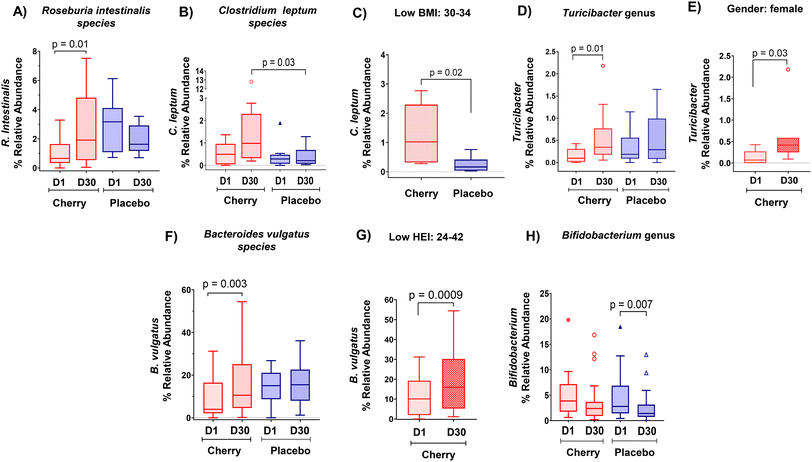 |
| Fig. 5 DSC supplementation promoted the abundance of SCFA bacteria producer in obese adults. Relative abundance of (A) R. intestinalis, (B) C. leptum (C) C. leptum stratified by low BMI: 30–34, (D) Turicibacter, (E) Turicibacter stratified by gender: female, (F) B. vulgatus, (G) B. vulgatus stratified by low HEI: 24–42 and (H) Bifidobacterium. The Wilcoxon matched-pairs rank test was performed to compare values within treatments. The Mann–Whitney test was assessed to evaluate differences between cherry and placebo treatments. A p value < 0.05 was considered statistically significant. Box plots show lower quartile, median, upper quartile, and Tukey whiskers. | |
Clostridium leptum species and the Agathobaculum genus share a common lineage within Clostridium cluster IV, indicating their phylogenetic relationship. Both C. leptum and Agathobaculum are involved in fermenting dietary fibers in the gut and producing SCFAs like butyrate. These bacterial species belong to the Ruminococcaceae family within the Firmicutes phylum. Results showed that relative abundance of Agathobaculum tends to decrease in placebo at D30 vs. D1 (Table 2); while levels in cherry remained unchanged. Agathobaculum is of interest as it has been linked to neuroprotective effects in animal models of Parkinson's disease.45 At species level, the relative abundance of C. leptum, which has been reported to be reduced in obese women,46 increased at D30 in the cherry group compared to the placebo group (p = 0.03) (Table 2 and Fig. 5B). This difference was more apparent in participants with low BMI (p = 0.02) (Fig. 5C). Our results are consistent with a previous study that observed increased levels of C. leptum after Goji berry supplementation for 10 weeks in IL-10 deficient mice.47
Erysipelotrichaceae family (Firmicutes phylum) abundance increased in placebo at D30 vs. D1 (p = 0.01) (Table 2). In addition, at genus level, Turicibacter showed a significant increase only in cherry (p = 0.01 at D30 vs. D1) (Table 2 and Fig. 5D), a result contributed by the changes found only in females (p = 0.03) (Fig. 5E). Some strains of Turicibacter signal bidirectionally with the host serotonergic system to promote their own fitness in the gut.48 Our results align with a previous study which reported an increase in Turicibacter abundance in rats fed with diet-induced obesity following supplementation with anthocyanin-rich Davidson's plum.49 Notably, Turicibacter abundance was correlated with enhanced intestinal barrier function induced by raspberry polysaccharides in obese mice.50 Therefore, the modulation of Turicibacter by DSC might potentially contribute to improving intestinal barrier function in obese adults.
Regarding Bacteroidaceae family (Bacteroidetes phylum) and lower taxa levels, no significant changes were found in the relative abundance within both experimental groups, except for Bacteroides vulgatus species whose abundance increased by DSC intake (p = 0.003) (Table 2 and Fig. 5F). This difference was highly significant in low HEI participants (p = 0.0009) (Fig. 5G) implying that obese adults with poor adherence to healthy dietary guidelines might benefit from DSC intake in hosting B. vulgatus. The modulation of B. vulgatus by DSC is relevant because this bacterium was shown to play a crucial role in sustaining a healthy gut ecosystem by reducing LPS activity and is depleted in individuals with obesity.51,52
Bifidobacteriaceae family (Actinobacteria phylum) plays an important role in preserving the integrity of the gut barrier function by protecting against the adhesion of pathogenic bacteria to the intestinal mucosa.53 Notably, consistent with changes at phylum level, Bifidobacteriaceae abundance decreased at D30 vs. D1 only in placebo (p = 0.02) (Table 2), with no significant changes detected in the cherry group. A similar trend was found for Bifidobacterium genus (Table 2 and Fig. 5H), which is known to help maintain the mucosal barrier and modulates LPS levels in the intestine.54 In contrast, the Eggerthellaceae family, which has been associated with initial stages of intestinal mucosa damage and enriched in adults having hyperplastic polyps,55 increased significantly in placebo over the intervention period (p = 0.04) while no changes were detected in the cherry group. Although the relative abundances of Bifidobacterium did not increase and Eggerthellaceae did not decrease significantly in the cherry group, 25% of participants showed a trend toward an increase in Bifidobacterium suggesting that DSC juice intake helped improve the relative abundances of these bacteria. Anthocyanins exert their beneficial effects as prebiotic substrate,44 which might have contributed to the observed outcomes in the cherry group.
Collectively, these results indicate complex associations between the native gut microbiome, diet, and gender-derived physiological differences.
3.3 DSC intake did not affect alpha diversity and beta diversity in obese adults
Alpha diversity (α) refers to the structure of a microbial community with respect to its richness (number of taxonomic groups), evenness (distribution of abundances of the groups) or both.56 In this study, there were no significant differences between cherry and placebo groups as determined by Shannon, observed OTUS, Chao 1, and Simpson index (ESI Table S4†). Beta diversity (β) summarizes which samples differ from one another by considering sequences abundances or considering only the presence-absence of sequences.56 The analysis within treatments (e.g., cherry D1 vs. D30 and placebo D1 vs. D30) revealed no significant differences for Bray Curtis, weighted and unweighted Unifrac and Jaccard distances (ESI Table S5†). Similarly, there were no significant differences in unweighted UniFrac and Jaccard distances between cherry and placebo groups at similar time points. In contrast, the weighted UniFrac distances, which considers the abundance of each taxon, showed significant differences between cherry and placebo at D1 and D30 (p = 0.04 in each group) despite similar age, gender proportions and BMI of the participants (ESI Fig. 2A†). Similarly, Bray–Curtis distances showed significant differences between cherry and placebo at D1 (p = 0.006) and D30 (p = 0.003) (ESI Fig. 2B†). Previous studies conducted with polyphenol-rich products have shown similar outcomes, suggesting that microbial richness and diversity seem associated with an individualized gut microbiota response.57 In this context, individuals with a resilient microbiota influenced by consistent dietary patterns are less likely to benefit from any given dietary intervention.
3.4 Biomarkers of intestinal inflammation and permeability were not altered by DSC intake
LBP is considered a surrogate marker of metabolic endotoxemia since the determination of plasma LPS is limited by the presence of endogenous inhibitors.26,58 Previous clinical studies have demonstrated an association between obesity and increased levels of LBP.59,60 In this study, the mean LBP levels in cherry and placebo groups were higher than those reported in healthy individuals (5–10 μg mL−1)61 and similar to those reported in obese subjects (8.5–17.1 μg mL−1);58,59 however, results showed no significant changes in plasma LBP in either group. The L/M test measures the flux rate of lactulose and mannitol, which are nonmetabolized sugars, across the intestinal epithelium.21 Interestingly, the L urinary excretion increased by D30 vs. D1 only in the cherry group (p = 0.0081), but the increase in L/M ratio did not reach significance (ESI Table S6†), with mean values within normal ranges (<0.07)58 at D1 and D30. In the placebo group instead, the mean L/M ratio at D1 was above normal range (0.11), but levels were lessened by D30 without reaching significance (ESI Table S6†). In general, the relationship between cherry intake and increase in L excretion should be further investigated while controlling the variety of factors influencing the increase in L excretion, including physiological, pathological, and environmental influences that were not accounted for in this study.
Additionally, REG-4 and IL-22 mRNA levels have emerged as potential biomarkers for gut inflammation.62 REG-4 is involved in mucosal repair and regeneration, which is essential for maintaining mucosal integrity in the context of gut inflammation.63 IL-22 is crucial for maintaining the integrity of the epithelial barrier in the gut. It promotes the production of antimicrobial peptides, mucus secretion, and epithelial cell survival, all of which are vital for protecting against pathogens and maintaining gut homeostasis.64 Results showed that REG-4 and IL-22 mRNA levels in fecal samples were not modulated by DSC intake, with no difference in the fold change found between cherry and placebo groups (ESI Table S6†). Therefore, based on the collected data (plasma LBP, L/M test, and fecal mRNA), it is suggested that intestinal permeability was not compromised in the obese participants enrolled in this study.
3.5 Correlation analyses of significant bacterial taxa and biomarkers assessed during intervention
In our previous study, we found that DSC supplementation reduced systolic and diastolic blood pressure (SBP and DBP respectively), and pro-inflammatory interferon gamma (IFNγ) in obese adults.14 Spearman correlation analysis was performed to determine whether there was a link between changes in the gut microbiota and SBP, DBP and IFNγ following DSC supplementation. Interestingly, Δ A. shahii and Δ Bilophila abundance were positively correlated with Δ IFNγ in the cherry group (Fig. 6). These results align with Li and colleagues65 who reported consistent positive correlations between Bilophila and pro-inflammatory cytokines including IFNγ in DSS-treated mice following resveratrol consumption. Moreover, significant negative correlations were found between Δ R. intestinalis, whose abundance increased only in cherry, and both Δ SBP and Δ Bilophila in the cherry group (Fig. 6). Collectively, these results suggest that gut microbial changes induced by DSC juice intake may contribute to both anti-inflammatory effect and blood-pressure lowering effects in obese adults. In the placebo group, Δ A. shahii exhibited significant negative correlation with Δ Turicibacter and Δ A. hadrus, while Δ B. vulgatus showed a positive correlation with Δ Bilophila. In addition, Δ Bilophila and Δ B. vulgatus were negatively correlated with Δ DBP (Figure not shown). Such correlations were not found in the cherry group.
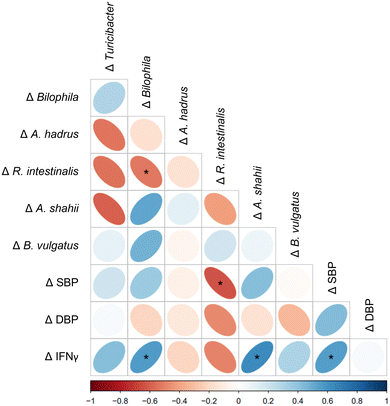 |
| Fig. 6 Correlations between gut microbiota and obesity-related host parameters. Spearman's correlations between relative abundance of bacterial taxa and host metabolic markers previously reported in the cherry group. Direction of ellipses represents positive (blue) or negative (orange) correlations, and the width represents the strength of correlation (narrow ellipse = stronger correlation). Correlations are considered significant if r coefficients are >0.4 and p < 0.05. Statistically significant correlations are marked by (*). | |
This study is, to our knowledge, the first evaluating DSC effects on the modulation of the gut microbiota and intestinal barrier function in healthy obese adults. The strengths of this study include the assessment of the dietary patterns for 15 days, which allowed a better estimation of nutrients that could impact gut microbiota. A follow-up study should consider the evaluation of microbial metabolites (i.e., SCFAs) in stool and plasma samples because it is well established that metabolites produced after fiber and polyphenol intake are better absorbed and might have specific biological effects on the host. Furthermore, the evaluation of multiple biomarkers for intestinal permeability and inflammation (such as the L/M ratio and fecal mRNA markers) could have provided a better insight into the impact of DSC on obesity-associated barrier dysfunction if participants had presented the condition. Future work may need to focus on obese adults with greater IBF impairment, who might benefit from DSC supplementation. Despite these limitations, this study provides a foundation for future exploration of DSC interventions on obesity-associated dysbiosis and intestinal barrier dysfunction.
4. Conclusions
This study demonstrated that DSC supplementation for 30 days may selectively modulate relevant bacterial taxa in the context of obesity. These effects include reducing the abundance of A. hadrus, Blautia that may be linked to intestinal inflammation, and metabolic disorders and preventing the increase of pro-inflammatory bacteria A. shahii and Bilophila. Additionally, DSC intake enhanced the growth of SCFA-producing bacteria such as R. intestinalis, C. leptum B. vulgatus and Turicibacter. Furthermore, DSC intake also helped to sustain the abundance of probiotic bacteria Bifidobacterium, which was reduced in the placebo group. Our results also add valuable information about the possible impact of DSC intake on gut microbiota by gender, adherence to dietary guidelines, and obesity grade, with BMI ≥ 35 showing to benefit the most. Intestinal permeability, evaluated by the LBP quantification, urinary excretion of L/M, and fecal mRNA biomarkers, were not affected by DSC supplementation. These results may be partly due to the study population not showing intestinal permeability alterations. In summary, this study advocates for additional research on the prevention of obesity-associated dysbiosis by employing DSC with metabolically unhealthy morbidly obese subjects to corroborate the current findings.
Author contributions
Study conception and design: G.N; funding acquisition: G.N; data acquisition: S.A and G.N; study supervision: G.N; analysis and data interpretation: S.A and G.N; writing-original draft preparation: S.A; writing – review and editing: S.A, G.N and S.M.T resources: G.N and S.M.T; critical review: G.N, S.M.T and S.T. All authors have read and agreed to the published version of the manuscript.
Data availability
• The DNA raw sequencing data is available in NCBI Bioproject under the Accession Number PRJNA859373.
• Data for this article, including the nutritional and physicochemical characteristics of DSC concentrated juice, DSC powder, and formulation of placebo concentrated drink can be accessed at https://www.mdpi.com/article/10.3390/nu15030681/s1.
• The anthropometric and physiological measurements, as well as nutrient intake and the HEI results were previously reported in detail and can be accessed at https://www.mdpi.com/2072-6643/15/3/681.
• The data supporting this article have been included as part of the ESI.†
Conflicts of interest
The authors declare no conflict of interest.
Acknowledgements
This study was funded by Northwest Cherry Growers. The authors would like to thank all participants for contributing their time and effort in this study. We also acknowledge Michael Perez for his support in the 16S rRNA sequencing analysis.
References
- L. Du, X. Lei, J. Wang, L. Wang, Q. Zhong, X. Fang, P. Li, B. Du, Y. Wang and Z. Liao, Lipopolysaccharides derived from Gram-negative bacterial pool of human gut microbiota promote inflammation and obesity development, Int. Rev. Immunol., 2022, 41, 45–56 CrossRef CAS PubMed.
- G. Muscogiuri, E. Cantone, S. Cassarano, D. Tuccinardi, L. Barrea, S. Savastano and A. Colao, E. R. on behalf of the Obesity Programs of nutrition and g. Assessment, Gut microbiota: a new path to treat obesity, Int. J. Obes. Suppl., 2019, 9, 10–19 CrossRef PubMed.
- F. Blando and B. D. Oomah, Sweet and sour cherries: Origin, distribution, nutritional composition and health benefits, Trends Food Sci. Technol., 2019, 86, 517–529 CrossRef CAS.
- M. F. Faienza, F. Corbo, A. Carocci, A. Catalano, M. L. Clodoveo, M. Grano, D. Q. Wang, G. D'Amato, M. Muraglia, C. Franchini, G. Brunetti and P. Portincasa, Novel insights in health-promoting properties of sweet cherries, J. Funct. Foods, 2020, 69, 103945 CrossRef CAS PubMed.
- N. N. Lage, M. A. A. Layosa, S. Arbizu, B. P. Chew, M. L. Pedrosa, S. Mertens-Talcott, S. Talcott and G. D. Noratto, Dark sweet cherry (Prunus avium) phenolics enriched in anthocyanins exhibit enhanced activity against the most aggressive breast cancer subtypes without toxicity to normal breast cells, J. Funct. Foods, 2020, 64, 103710 CrossRef CAS.
- D. S. Kelley, Y. Adkins and K. D. Laugero, A Review of the Health Benefits of Cherries, Nutrients, 2018, 10, 368 CrossRef PubMed.
- R. Lear, M. O’Leary, L. O’Brien Andersen, C. C. Holt, C. R. Stensvold, M. van der Giezen and J. L. Bowtell, Tart Cherry Concentrate Does Not Alter the Gut Microbiome, Glycaemic Control or Systemic Inflammation in a Middle-Aged Population, Nutrients, 2019, 11, 1063 CrossRef CAS PubMed.
- A. R. Hillman and B. C. R. Chrismas, Thirty Days of Montmorency Tart Cherry Supplementation Has No Effect on Gut Microbiome Composition, Inflammation, or Glycemic Control in Healthy Adults, Front. Nutr., 2021, 8, 733057 CrossRef PubMed.
- A. C. Mayta-Apaza, E. Pottgen, J. De Bodt, N. Papp, D. Marasini, L. Howard, L. Abranko, T. Van de Wiele, S. O. Lee and F. Carbonero, Impact of tart cherries polyphenols on the human gut microbiota and phenolic metabolites in vitro and in vivo, J. Nutr. Biochem., 2018, 59, 160–172 CrossRef CAS PubMed.
- T. A. Suzuki and M. Worobey, Geographical variation of human gut microbial composition, Biol. Lett., 2014, 10, 20131037 CrossRef PubMed.
- A. Kaur, B. A. Ojo, S. Y. Wong, S. E. Alake, M. Pastor, G. Davila-El Rassi, D. Lin, B. J. Smith and E. A. Lucas, Montmorencytart cherry supplementation improved markers of glucose homeostasis but has modest effects on indicators of gut health in mice fed a Western diet, Nutr. Res., 2022, 99, 66–77 CrossRef CAS PubMed.
- A. Al Othaim, D. Marasini and F. Carbonero, Impact of increasing concentration of tart and sweet cherries juices concentrates on healthy mice gut microbiota, Food Front., 2020, 1, 224–233 CrossRef.
- J. F. Garcia-Mazcorro, N. N. Lage, S. Mertens-Talcott, S. Talcott, B. Chew, S. E. Dowd, J. R. Kawas and G. D. Noratto, Effect of dark sweet cherry powder consumption on the gut microbiota, short-chain fatty acids, and biomarkers of gut health in obese db/db mice, PeerJ, 2018, 6, e4195 CrossRef PubMed.
- S. Arbizu, S. U. Mertens-Talcott, S. Talcott and G. D. Noratto, Dark Sweet Cherry (Prunus avium) Supplementation Reduced Blood Pressure and Pro-Inflammatory Interferon Gamma (IFNχ) in Obese Adults without Affecting Lipid Profile, Glucose Levels and Liver Enzymes, Nutrients, 2023, 15, 681 CrossRef CAS PubMed.
- C. Y. Lim and J. In, Randomization in clinical
studies, Korean J. Anesthesiol., 2019, 72, 221–232 CrossRef PubMed.
- S. M. Krebs-Smith, T. E. Pannucci, A. F. Subar, S. I. Kirkpatrick, J. L. Lerman, J. A. Tooze, M. M. Wilson and J. Reedy, Update of the Healthy Eating Index: HEI-2015, J. Acad. Nutr. Diet., 2018, 118, 1591–1602 CrossRef PubMed.
- K. He, H. Fujiwara, C. Zajac, E. Sandford, P. Reddy, S. W. Choi and M. Tewari, A Pipeline for Faecal Host DNA Analysis by Absolute Quantification of LINE-1 and Mitochondrial Genomic Elements Using ddPCR, Sci. Rep., 2019, 9, 5599 CrossRef PubMed.
- A. E. Schriefer, B. Kumar, A. Zolty, R. Preetam, A. Didier, M. Nirmal, N. Nadiv, M. Perez, S. K. Mahankuda and P. Kumar, M-CAMP™: A cloud-based web platform with a novel approach for species-level classification of 16S rRNA microbiome sequences, Curr. Bioinf., 2021, 18, 21–39 Search PubMed.
- J. Brignardello, P. Morales, E. Diaz, J. Romero, O. Brunser and M. Gotteland, Pilot study: alterations of intestinal microbiota in obese humans are not associated with colonic inflammation or disturbances of barrier function, Aliment. Pharmacol. Ther., 2010, 32, 1307–1314 CrossRef CAS PubMed.
- P. Kubica, A. Kot-Wasik, A. Wasik, J. Namiesnik and P. Landowski, Modern approach for determination of lactulose, mannitol and sucrose in human urine using HPLC-MS/MS for the studies of intestinal and upper digestive tract permeability, J. Chromatogr. B: Anal. Technol. Biomed. Life Sci., 2012, 907, 34–40 CrossRef CAS PubMed.
- M. A. Musa, M. Kabir, M. I. Hossain, E. Ahmed, A. Siddique, H. Rashid, M. Mahfuz, D. Mondal, T. Ahmed, W. A. Petri and R. Haque, Measurement of intestinal permeability using lactulose and mannitol with conventional five hours and shortened two hours urine collection by two different methods: HPAE-PAD and LC-MSMS, PLoS One, 2019, 14, e0220397 CrossRef CAS PubMed.
- S. Agapova, K. Stephenson, M. Manary, A. Weisz, P. I. Tarr, R. Mkakosya, K. Maleta, R. J. Shulman, M. Manary and N. Shaikh, Detection of low-concentration host mRNA transcripts in Malawian children at risk for environmental enteropathy, J. Pediatr. Gastroenterol. Nutr., 2013, 56, 66–71 CrossRef CAS PubMed.
- T. D. Schmittgen and K. J. Livak, Analyzing real-time PCR data by the comparative C(T) method, Nat. Protoc., 2008, 3, 1101–1108 CrossRef CAS PubMed.
- V. F. Rodrigues, J. Elias-Oliveira, Í.S Pereira, J. A. Pereira, S. C. Barbosa, M. S. G. Machado and D. Carlos, Akkermansia muciniphila and gut immune system: a good friendship that attenuates inflammatory bowel disease, obesity, and diabetes, Front. Immunol., 2022, 13, 934695 CrossRef CAS PubMed.
- P. D. Cani, C. Depommier, M. Derrien, A. Everard and W. M. de Vos, Akkermansia muciniphila: paradigm for next-generation beneficial microorganisms, Nat. Rev. Gastroenterol. Hepatol., 2022, 19, 625–637 CrossRef PubMed.
- A. González-Sarrías, M. Romo-Vaquero, R. García-Villalba, A. Cortés-Martín, M. V. Selma and J. C. Espín, The endotoxemia marker lipopolysaccharide–binding protein is reduced in overweight–obese subjects consuming pomegranate extract by modulating the gut microbiota: a randomized clinical trial, Mol. Nutr. Food Res., 2018, 62, 1800160 CrossRef PubMed.
- V. Singh, G. Lee, H. Son, H. Koh, E. S. Kim, T. Unno and J.-H. Shin, Butyrate producers,“The Sentinel of Gut”: Their intestinal significance with and beyond butyrate, and prospective use as microbial therapeutics, Front. Microbiol., 2023, 13, 1103836 CrossRef PubMed.
- Q. Zhang, Y. Wu, J. Wang, G. Wu, W. Long, Z. Xue, L. Wang, X. Zhang, X. Pang, Y. Zhao, L. Zhao and C. Zhang, Accelerated dysbiosis of gut microbiota during aggravation of DSS-induced colitis by a butyrate-producing bacterium, Sci. Rep., 2016, 6, 27572 CrossRef CAS PubMed.
- D. Kashtanova, O. Tkacheva, E. Doudinskaya, I. Strazhesko, Y. Kotovskaya and A. Popenko, Gut microbiota in patients with different metabolic statuses: Moscow study, Microorganisms, 2018, 6, 98 CrossRef CAS PubMed.
- M. Duan, Y. Wang, Q. Zhang, R. Zou, M. Guo and H. Zheng, Characteristics of gut microbiota in people with obesity, PLoS One, 2021, 16, e0255446 CrossRef CAS PubMed.
- L. Zhu, L. Sha, K. Li, Z. Wang, T. Wang, Y. Li, P. Liu, X. Dong, Y. Dong, X. Zhang and H. Wang, Dietary flaxseed oil rich in omega-3 suppresses severity of type 2 diabetes mellitus via anti-inflammation and modulating gut microbiota in rats, Lipids Health Dis., 2020, 19, 1–16 CrossRef PubMed.
- J. Companys, M. J. Gosalbes, L. Pla-Paga, L. Calderon-Perez, E. Llaurado, A. Pedret, R. M. Valls, N. Jimenez-Hernandez, B. A. Sandoval-Ramirez, J. M. Del Bas, A. Caimari, L. Rubio and R. Sola, Gut Microbiota Profile and Its Association with Clinical Variables and Dietary Intake in Overweight/Obese and Lean Subjects: A Cross-Sectional Study, Nutrients, 2021, 13, 2032 CrossRef CAS PubMed.
- B. J. Parker, P. A. Wearsch, A. C. M. Veloo and A. Rodriguez-Palacios, The Genus Alistipes: Gut Bacteria With Emerging Implications to Inflammation, Cancer, and Mental Health, Front. Immunol., 2020, 11, 906 CrossRef CAS PubMed.
- E. Rinninella, P. Raoul, M. Cintoni, F. Franceschi, G. A. D. Miggiano, A. Gasbarrini and M. C. Mele, What is the healthy gut microbiota composition? A changing ecosystem across age, environment, diet, and diseases, Microorganisms, 2019, 7, 14 CrossRef CAS PubMed.
- S. A. Joyce and C. G. Gahan, The gut microbiota and the metabolic health of the host, Curr. Opin. Gastroenterol., 2014, 30, 120–127 CrossRef CAS PubMed.
- W. J. Dahl, D. Rivero Mendoza and J. M. Lambert, Diet, nutrients and the microbiome, Prog. Mol. Biol. Transl. Sci., 2020, 171, 237–263 CAS.
- J. M. Natividad, B. Lamas, H. P. Pham, M. L. Michel, D. Rainteau, C. Bridonneau, G. da Costa, J. van Hylckama Vlieg, B. Sovran, C. Chamignon, J. Planchais, M. L. Richard, P. Langella, P. Veiga and H. Sokol, Bilophila wadsworthia aggravates high fat diet induced metabolic dysfunctions in mice, Nat. Commun., 2018, 9, 2802 CrossRef PubMed.
- M. L. Clodoveo, P. Crupi, M. Muraglia, M. Y. Naeem, R. Tardugno, F. Limongelli and F. Corbo, The main phenolic compounds responsible for the antioxidant capacity of sweet cherry (Prunus avium L.) pulp, LWT, 2023, 185, 115085 CrossRef CAS.
- J. Fernandez, L. Garcia, J. Monte, C. J. Villar and F. Lombo, Functional Anthocyanin-Rich Sausages Diminish Colorectal Cancer in an Animal Model and Reduce Pro-Inflammatory Bacteria in the Intestinal Microbiota, Genes, 2018, 9, 133 CrossRef PubMed.
- T. A. Verediano, H. Stampini Duarte Martino, M. C. Dias Paes and E. Tako, Effects of Anthocyanin on Intestinal Health: A Systematic Review, Nutrients, 2021, 13, 1331 CrossRef CAS PubMed.
- K. Nie, K. Ma, W. Luo, Z. Shen, Z. Yang, M. Xiao, T. Tong, Y. Yang and X. Wang, Roseburia intestinalis: A Beneficial Gut Organism From the Discoveries in Genus and Species, Front. Cell. Infect. Microbiol., 2021, 11, 757718 CrossRef CAS PubMed.
- Y. Xian, R. Fan, J. Shao, A. Mulcahy Toney, S. Chung and A. E. Ramer-Tait, Polyphenolic fractions isolated from red raspberry whole fruit, pulp, and seed differentially alter the gut microbiota of mice with diet-induced obesity, J. Funct. Foods, 2021, 76, 104288 CrossRef CAS.
- Z. Tamanai-Shacoori, I. Smida, L. Bousarghin, O. Loreal, V. Meuric, S. B. Fong, M. Bonnaure-Mallet and A. Jolivet-Gougeon, Roseburia spp.: a marker of health?, Future Microbiol., 2017, 12, 157–170 CrossRef CAS PubMed.
- A. M. Alves-Santos, C. S. A. Sugizaki, G. C. Lima and M. M. V. Naves, Prebiotic effect of dietary polyphenols: A systematic review, J. Funct. Foods, 2020, 74, 104169 CrossRef CAS.
- J. E. Kenna, E. G. Chua, M. Bakeberg, A. Tay, S. McGregor, A. Gorecki, M. Horne, B. Marshall, F. L. Mastaglia and R. S. Anderton, Changes in the Gut Microbiome and Predicted Functional Metabolic Effects in an Australian Parkinson's Disease Cohort, Front. Neurosci., 2021, 15, 756951 CrossRef PubMed.
- T. Teixeira, Ł.M Grześkowiak, S. Salminen, K. Laitinen, J. Bressan and M. D. C. G. Peluzio, Faecal levels of Bifidobacterium and Clostridium coccoides but not plasma lipopolysaccharide are inversely related to insulin and HOMA index in women, Clin. Nutr., 2013, 32, 1017–1022 CrossRef PubMed.
- Y. Kang, G. Yang, S. Zhang, C. F. Ross and M. J. Zhu, Goji Berry Modulates Gut Microbiota and Alleviates Colitis in IL-10-Deficient Mice, Mol. Nutr. Food Res., 2018, 62, e1800535 CrossRef PubMed.
- T. C. Fung, H. E. Vuong, C. D. G. Luna, G. N. Pronovost, A. A. Aleksandrova, N. G. Riley, A. Vavilina, J. McGinn, T. Rendon, L. R. Forrest and E. Y. Hsiao, Intestinal serotonin and fluoxetine exposure modulate bacterial colonization in the gut, Nat. Microbiol., 2019, 4, 2064–2073 CrossRef PubMed.
- O. D. John, P. Mouatt, I. Prasadam, Y. Xiao, S. K. Panchal and L. Brown, The edible native Australian fruit, Davidson's plum (Davidsonia pruriens), reduces symptoms in rats with diet-induced metabolic syndrome, J. Funct. Foods, 2019, 56, 204–215 CrossRef CAS.
- Y. Huang, J. Hu, Q. Xia, M. Tang, Y. Wang, G. Wang, X. Shao, H. Yuan, S. Li, P. Huang, C. Peng, J. Guo and S. Gui, Amelioration of obesity and inflammation by polysaccharide from unripe fruits of raspberry via gut microbiota regulation, Int. J. Biol. Macromol., 2024, 261, 129825 CrossRef CAS PubMed.
- N. Yoshida, T. Yamashita, T. Osone, T. Hosooka, M. Shinohara, S. Kitahama, K. Sasaki, D. Sasaki, T. Yoneshiro, T. Suzuki, T. Emoto, Y. Saito, G. Ozawa, Y. Hirota, Y. Kitaura, Y. Shimomura, Y. Okamatsu-Ogura, M. Saito, A. Kondo, S. Kajimura, T. Inagaki, W. Ogawa, T. Yamada and K. I. Hirata, Bacteroides spp. promotes branched-chain amino acid catabolism in brown fat and inhibits obesity, iScience, 2021, 24, 103342 CrossRef CAS PubMed.
- C. Wang, Y. Xiao, L. Yu, F. Tian, J. Zhao, H. Zhang, W. Chen and Q. Zhai, Protective effects of different Bacteroides vulgatus strains against lipopolysaccharide-induced acute intestinal injury, and their underlying functional genes, J. Adv. Res., 2022, 36, 27–37 CrossRef CAS PubMed.
- A. Riviere, M. Selak, D. Lantin, F. Leroy and L. De Vuyst, Bifidobacteria and Butyrate-Producing Colon Bacteria: Importance and Strategies for Their Stimulation in the Human Gut, Front. Microbiol., 2016, 7, 979 Search PubMed.
- M. R. Pinzone, B. M. Celesia, M. Di Rosa, B. Cacopardo and G. Nunnari, Microbial translocation in chronic liver diseases, Int. J. Microbiol., 2012, 2012, 694629 Search PubMed.
- S. Ruiz-Saavedra, S. Arboleya, A. M. Nogacka, C. Gonzalez Del Rey, A. Suarez, Y. Diaz, M. Gueimonde, N. Salazar, S. Gonzalez and C. G. de Los Reyes-Gavilan, Commensal Fecal Microbiota Profiles Associated with Initial Stages of Intestinal Mucosa Damage: A Pilot Study, Cancers, 2023, 16, 104 CrossRef PubMed.
- J. G. Kers and E. Saccenti, The power of microbiome studies: Some considerations on which alpha and beta metrics to use and how to report results, Front. Microbiol., 2022, 12, 796025 CrossRef PubMed.
- G. R. Healey, R. Murphy, L. Brough, C. A. Butts and J. Coad, Interindividual variability in gut microbiota and host response to dietary interventions, Nutr. Rev., 2017, 75, 1059–1080 CrossRef PubMed.
- B. Seethaler, M. Basrai, A. M. Neyrinck, J. A. Nazare, J. Walter, N. M. Delzenne and S. C. Bischoff, Biomarkers for assessment of intestinal permeability in clinical practice, Am. J. Physiol.: Gastrointest. Liver Physiol., 2021, 321, G11–G17 CrossRef CAS PubMed.
- J. Moreno-Navarrete, F. Ortega, M. Serino, E. Luche, A. Waget, G. Pardo, J. Salvador, W. Ricart, G. Frühbeck and R. Burcelin, Circulating lipopolysaccharide-binding protein (LBP) as a marker of obesity-related insulin resistance, Int. J. Obes., 2012, 36, 1442–1449 CrossRef CAS PubMed.
- K. E. Kim, Y. S. Cho, K. S. Baek, L. Li, K.-H. Baek, J. H. Kim, H.-S. Kim and Y. H. Sheen, Lipopolysaccharide-binding protein plasma levels as a biomarker of obesity-related insulin resistance in adolescents, Korean J. Pediatr., 2016, 59, 231 CrossRef CAS PubMed.
- L. Meng, Z. Song, A. Liu, U. Dahmen, X. Yang and H. Fang, Effects of Lipopolysaccharide-Binding Protein (LBP) Single Nucleotide Polymorphism (SNP) in Infections, Inflammatory Diseases, Metabolic Disorders and Cancers, Front. Immunol., 2021, 12, 681810 CrossRef CAS PubMed.
- S. Agapova, K. Stephenson, M. Manary, A. Weisz, P. I. Tarr, R. Mkakosya, K. Maleta, R. J. Shulman, M. Manary and N. Shaikh, Detection of low-concentration host mRNA transcripts in Malawian children at risk for environmental enteropathy, J. Pediatr. Gastroenterol. Nutr., 2013, 56, 66–71 CrossRef CAS PubMed.
- C. Sun, X. Wang, Y. Hui, H. Fukui, B. Wang and H. Miwa, The Potential Role of REG Family Proteins in Inflammatory and Inflammation-Associated Diseases of the Gastrointestinal Tract, Int. J. Mol. Sci., 2021, 22, 7196 CrossRef CAS PubMed.
- O. B. Parks, D. A. Pociask, Z. Hodzic, J. K. Kolls and M. Good, Interleukin-22 Signaling in the Regulation of Intestinal Health and Disease, Front. Cell Dev. Biol., 2015, 3, 85 Search PubMed.
- F. Li, Y. Han, X. Cai, M. Gu, J. Sun, C. Qi, T. Goulette, M. Song, Z. Li and H. Xiao, Dietary resveratrol attenuated colitis and modulated gut microbiota in dextran sulfate sodium-treated mice, Food Funct., 2020, 11, 1063–1073 RSC.
|
This journal is © The Royal Society of Chemistry 2024 |
Click here to see how this site uses Cookies. View our privacy policy here.