DOI:
10.1039/D4FO00906A
(Paper)
Food Funct., 2024,
15, 5785-5796
Efficient regioselective enzymatic acylation of troxerutin: difference characterization of in vitro cellular uptake and cytotoxicity†
Received
26th February 2024
, Accepted 9th April 2024
First published on 11th April 2024
Abstract
In this study, we developed a multi-site acylation strategy to improve the lipophilicity and cellular uptake of troxerutin, a natural flavonoid with many health-promoting bioactivities. By clarifying the acylation properties of troxerutin catalyzed by lipases from different sources, a series of troxerutin ester derivatives acylated at different sites was synthesized, including troxerutin dipropyl (TDP), tripropyl (TTP), tetrapropyl (TEP), dibutyl (TDB), monohexyl (TMH), monooctyl (TMO) and monodecyl (TMD) esters. Interestingly, the troxerutin esters acylated at multiple sites with shorter fatty chains (TDP, TTP and TEP) had similar lipophilicity to the mono-acylated esters bearing longer fatty chains (TMH, TMO and TMD, respectively) and meanwhile demonstrated surprisingly lower cytotoxicity than that of the long fatty-chain mono-esters. In particular, the multi-acylated esters with shorter fatty chains showed remarkably higher cellular uptake than the mono-esters with long fatty chains. In vitro gastrointestinal digestion suggested that the multi-acylated esters of troxerutin were more resistant to gastrointestinal degradation than the mono-esters. These results indicated that multi-site acylation with short fatty chains could be an effective alternative to introducing one-site mono-acylation for the modification of troxerutin and other flavonoid compounds.
1. Introduction
Troxerutin (TX) is a natural flavonoid present in tea, coffee, cereal grains and pagoda tree.1–3 TX, also known as vitamin P4, exhibits a plethora of biological activities, including antioxidant, anti-thrombotic, anti-diabetic, and anti-inflammatory properties.3,4 Studies have shown that the lipophilicity of low molecular weight phenolic compounds plays a significant role in determining their passive permeability through the lipid bilayer.5 The low lipophilicity of TX, however, presents a challenge for its dissolution in non-aqueous phases and its permeation through cell membranes, thereby restricting its potential applications in the food, cosmetic, and pharmaceutical industries.6,7
Acylation of hydroxyl groups has been proved to be efficient in improving the lipophilicity of flavonoid glycosides.8–11 Besides lipophilicity, the acylation of flavonoid glycosides may also improve their bioavailability and bioactivities.6 In contrast to these beneficial effects of acylation, recent studies indicate that some phenolic glycoside mono-esters bearing medium or long alkyl chains exhibit nonselective cytotoxicity due to the membrane-disrupting properties conferred by these fatty chains.12 Thus an issue arose as to how to increase the lipophilicity of compounds without increasing the toxicity caused by medium or long fatty chains when applying the acylation strategy. Bala et al. found that di-acylation at the C10 and C20 positions of 7-ethyl-10-hydroxy camptothecin was more effective than mono-acylation in reducing the cytotoxicity associated with the medium length fatty chains.13 This suggested that multi-site acylation may be an alternative to mono-acylation taking the cytotoxicity into consideration.
Acylation can be achieved through chemical or enzymatic catalysis, and the latter is generally preferred for the modification of natural products due to its moderate reaction conditions, low environmental impact, and high regioselectivity.14 Previous research have tried to selectively acylate flavonoids. However, due to the selectivity of the enzymes, most acylation of flavonoid glycosides occurred at one or two sites, giving mono-esters or sometimes di-esters.15–17 In our previous study, we explored a whole-cell catalytic strategy for catalyzing the acylation of troxerutin and the propionyl group was successfully introduced to troxerutin, giving a mono-propionyl ester and a di-propionyl ester both acylated at the primary hydroxyl groups. As a typical flavonoid glycoside, TX has three primary hydroxyl groups on its ethoxy groups at the aromatic trimeric heterocyclic structure and many secondary hydroxyl groups on the sugar ring. Although these hydroxyl groups can be targeted for acylation, only mono-esters and di-esters of TX were reported in current studies. Selective acylation of TX at different hydroxyl groups with varying alkyl chain-length acyl donors still remains surprisingly unexplored. The relationships between the structures/acyl sites of the acylated flavonoids and their physicochemical properties and biological activities are also largely unclear.
Herein, we developed an efficient enzymatic method to cope with the challenges in multi-site acylation of TX. The regioselective acylation properties of TX with various lipases (EC 3.1.1.3) derived from different sources were successfully elucidated, and a series of troxerutin derivatives was synthesized with different degrees of acylation. Subsequently, the lipophilicity of these troxerutin ester derivatives was evaluated on the basis of their oil–water partition coefficients. The cytotoxicity and cellular uptake ability of these ester derivatives were also investigated using Caco-2 cell models and the digestive stability of these troxerutin derivatives was evaluated using a simulated gastrointestinal model in vitro. This study will provide new insights into the controlled acylation of flavone glycosides by enzymes, and new approaches to produce modified natural compounds with enhanced biological activities.
2. Materials and methods
2.1 Materials
Troxerutin (97% purity) was purchased from Aladdin (Los Angeles, CA, USA). Novozym 435 (Candida Antarctica lipase B), Lipase 40086 (Rhizopus miehei lipase), Lipozyme TL IM (Thermomyces lanuginosus lipase), and Lipex 100T were purchased from Novozyms Co. Ltd (Bagsvaerd, Denmark). Lipase PS IM (Pseudomonas cepacian lipase), Lipase A (Aspergillus niger lipase), and Lipase AY30 (Candida. rugosa lipase) were purchased from Amano Co. Ltd (Japan). Vinyl propionate (VP), vinyl butyrate (VB), vinyl hexanoate (VH), and vinyl octanoate (VO), and vinyl decanoate (VD) were purchased from TCI (Tokyo, Japan). Fetal bovine serum (FBS), phosphate buffered saline (PBS, pH 7.4), Dulbecco's modified Eagle's medium (DMEM), penicillin and streptomycin were purchased from Gibco Life Technologies Co. Ltd (Grand Island, NY, USA). Caco-2 cells were provided by the Medical College of Sun Yat-Sen University (Guangzhou, China). All other chemicals were obtained from commercial sources and were of the highest available purity.
2.2 General procedure for lipase-mediated acylation of troxerutin
In brief, TX (30 mmol L−1) was dissolved in n-heptane/pyridine (1 mL, 35/65 v/v). This solution was mixed with defined acyl donor stoichiometries and lipase enzymes in a 5 mL Erlenmeyer flask equipped with a rubber serum cap. The acylation mixture was incubated by shaking at 180 rpm and 50 °C for a defined duration. Samples (20 μL) were collected and diluted 50-fold (to 2% original concentration) with methanol before high performance liquid chromatography (HPLC) analysis. A blank control was established in the absence of lipase and each experiment was performed in triplicate. Finally, the reaction medium and acyl donor were separated by centrifugation and spin distillation.
2.3 HPLC analysis
An Agilent 1260 UV detector and Agilent 1260 quat pump (Agilent Corp. California, USA) were applied with an Agilent Zorbax SB-C18 (4.6 mm × 250 mm, 5 μm) analytical column maintained at 30 °C and a detection wavelength of 350 nm. The mobile phase consisted of two components: A – 0.2% phosphoric acid aqueous solution; and B – methanol. For TX, troxerutin dipropyl (TDP), troxerutin tripropyl (TTP), troxerutin tetrapropyl (TEP), and troxerutin dibutyl (TDB) esters, the elution conditions were 0–7.5 min with 62% B, 7.5–17 min with 73% B, and 17–20 min with 80% B. For troxerutin monohexyl (TMH), troxerutin monooctyl (TMO), and troxerutin monodecyl (TMD) esters, the elution conditions were 0–5 min with 62% B and 5–15 min with 90% B. The flow rate was 0.9 mL min−1. The retention times of TX, TDP, TTP, TEP, TDB, TMH, TMO, TMD were 3.21, 11.84, 15.55, 17.61, 13.84, 10.45, 11.18 and 12.24 min, respectively.
The formulae to calculate initial velocity, substrate conversion rate, regioselectivity and yield were as follows:
| Initial velocity (mmol L−1 h−1) = (C0 – Ct)/t | (1) |
| Conversion (%) = (S0 − S)/S0 × 100 | (2) |
| Regioselectivity (%) = (S1/St) × 100 | (3) |
| Yield (%) = C/C0 × 100 | (4) |
where
C0 (mmol L
−1) is the initial concentration of TX,
Ct (mmol L
−1) is the concentration of TX after a period of reaction,
C (mmol L
−1) is the concentration of the products,
S0 and
S are the peak areas of TX before and after the reaction, and
S1 and
St are the peak areas of the target products and total ester derivatives after the reaction.
2.4 Separation and structural characterization of the products
Acylation mixtures were purified using thin layer chromatography (TLC) with ethyl acetate/methanol/H2O as the eluent, 10
:
1
:
0.5 v/v/v for TDP/TTP/TDB and 20
:
1
:
0.5 v/v/v for TEP. Finally, a total of seven troxerutin derivatives were successfully synthesized and isolated as powders. Structural characterization was carried out by high-resolution mass spectrometry (HRMS), Fourier transform infrared (FT-IR), and NMR carbon (13C-NMR) spectroscopy. Molecular weights were determined by HRMS using electrospray ionization in positive ion mode with a scanning range of m/z 100–1000. The specific ester derivative functional groups (C
O) were identified by FT-IR spectroscopy. 600 MHz 13C-NMR spectrometry was used to identify the acylation reaction site. Full characterization results were given in the ESI.†
2.4.1 Troxerutin (TX).
13C-NMR (600 MHz, DMSO-d6) δ 177.93 (C-4), 165.11 (C-7), 161.29 (C-9), 157.04 (C-5), 156.90 (C-2), 151.31 (C-4′), 147.95 (C-3′), 134.12 (C-3), 122.99 (C-1′), 122.82 (C-6′), 114.80 (C-5′), 113.19 (C-2′), 105.51 (C-10), 101.76 (C-1′′), 101.39 (C-1′′′), 98.83 (C-6), 93.33 (C-8), 76.82 (C-3′′), 76.40 (C-5′′), 74.61 (C-2′′), 72.20 (C-4′′′), 71.05 (C-3′′′), 70.98 (C-2′′′), 70.94 (C-4′′), 70.78 (C-A′1), 70.76 (C-A′2), 70.64 (C-A), 68.74 (C-5′′′), 67.61 (C-6′′), 60.00 (C-B′1), 59.96 (C-B′2), 59.76 (C-B), 18.16 (C-6′′′).
2.4.2 Troxerutin dipropyl ester (TDP).
13C-NMR (600 MHz, DMSO-d6) δ 177.65 (C-4), 174.17 (C
O), 174.12 (C
O), 164.40 (C-7), 162.75 (C-9), 157.18 (C-5), 156.41 (C-2), 150.69 (C-4′), 148.08 (C-3′), 134.46 (C-3), 123.51 (C-1′), 122.89 (C-6′), 115.13 (C-5′), 113.75 (C-2′), 106.31 (C-10), 101.84 (C-1′′), 101.40 (C-1′′′), 99.03 (C-6), 92.62 (C-8), 76.92 (C-3′′), 76.44 (C-5′′), 74.62 (C-2′′), 72.24 (C-4′′′), 71.09 × 2 (C-3′′′, C-2′′′), 70.75 (C-4′′), 70.59 (C-A′1), 68.73 (C-5′′′), 67.59 (C-6′′), 67.25 (C-A′2), 67.00 (C-A), 62.86 (C-B′2), 62.61 (C-B), 59.98 (C-B′1), 27.21 (CH2), 27.17 (CH2), 18.18 (C-6′′′), 9.41 × 2 (CH3 × 2).
2.4.3 Troxerutin tripropyl ester (TTP).
13C-NMR (600 MHz, DMSO-d6) δ 177.84 (C-4), 174.12 × 3 (C
O × 3), 164.47 (C-7), 161.93 (C-9), 157.00 (C-5), 156.58 (C-2), 150.93 (C-4′), 147.62 (C-3′), 134.46 (C-3), 123.68 (C-1′), 123.42 (C-6′), 115.69 (C-5′), 113.92 (C-2′), 105.97 (C-10), 101.91 (C-1′′), 101.35 (C-1′′′), 98.90 (C-6), 93.16 (C-8), 76.86 (C-3′′), 76.43 (C-5′′), 74.64 (C-2′′), 72.23 (C-4′′′), 71.10 (C-3′′′), 70.78 (C-2′′′), 70.62 (C-4′′), 68.72 (C-5′′′), 67.52 (C-6′′), 67.47 (C-A′1), 67.26 (C-A′2), 67.10 (C-A), 62.84 (C-B′1), 62.83 (C-B′2), 62.58 (C-B), 27.19 (CH2), 27.17 × 2 (CH2 × 2), 18.15 (C-6′′′), 9.40 × 2 (CH3 × 2), 9.38 (CH3).
2.4.4 Troxerutin tetrapropyl ester (TEP).
13C NMR (600 MHz, DMSO-d6) δ 177.84 (C-4), 174.11 (C
O), 174.08 (C
O × 2), 173.76 (C
O), 164.51 (C-7), 162.20 (C-9), 156.96 (C-5), 156.33 (C-2), 150.94 (C-4′), 147.69 (C-3′), 134.31 (C-3), 123.57 (C-1′), 123.41 (C-6′), 115.62 (C-5′), 113.99 (C-2′), 105.89 (C-10), 101.72 (C-1′′), 101.29 (C-1′′′), 98.88 (C-6), 92.98 (C-8), 76.89 (C-3′′), 76.14 (C-5′′), 74.68 (C-4′′′), 73.90 (C-2′′), 70.78 (C-3′′′ C-2′′′), 70.42 (C-4′′), 67.76 (C-6′′), 67.49 (C-A′1), 67.30 (C-A′2), 67.13 (C-A), 66.29 (C-5′′′), 62.80 (C-B′1 C-B′2), 62.56 (C-B), 27.19 (CH2 × 2), 27.16 (CH2 × 2), 17.54 (C-6′′′), 9.43 (CH3), 9.39 (CH3 × 2), 9.38 (CH3).
2.4.5 Troxerutin dibutyl ester (TDB).
13C NMR (600 MHz, DMSO-d6) δ 177.87 (C-4), 173.30 (C
O), 173.23 (C
O), 164.46 (C-7), 161.86 (C-9), 156.98 (C-5), 156.75 (C-2), 150.77 (C-4′), 148.10 (C-3′), 134.34 (C-3), 123.41 (C-1′), 122.99 (C-6′), 115.15 (C-5′), 113.73 (C-2′), 105.93 (C-10), 101.80 (C-1′′), 101.39 (C-1′′′), 98.88 (C-6), 93.15 (C-8), 76.88 (C-3′′), 76.44 (C-5′′), 74.62 (C-2′′), 72.22 (C-4′′′), 71.08 (C-3′′′, C-2′′′), 70.78 (C-A′1), 70.60 (C-4′′), 68.73 (C-5′′′), 67.58 (C-6′′), 67.23 (C-A′2), 67.12 (C-A), 62.75 (C-B′2), 62.47 (C-B), 60.00 (C-B′1), 35.73 (CH2), 35.68 (CH2), 18.37 (CH2 × 2), 18.17 (C-6′′′), 13.87 (CH3), 13.82 (CH3).
2.4.6 Troxerutin monohexyl ester (TMH).
13C-NMR (600 MHz, DMSO-d6) δ 177.97 (C-4), 173.36 (C
O), 164.50 (C-7), 161.39 (C-9), 157.11 (C-5), 156.86 (C-2), 151.35 (C-4′), 147.98 (C-3′), 134.16 (C-3), 123.00 (C-1′), 122.79 (C-6′), 114.76 (C-5′), 113.20 (C-2′), 105.72 (C-10), 101.75 (C-1′′), 101.39 (C-1′′′), 98.79 (C-6), 93.48 (C-8), 76.83 (C-3′′), 76.41 (C-5′′), 74.61 (C-2′′), 72.20 (C-4′′′), 71.06 (C-3′′′), 70.99 (C-2′′′), 70.78 (C-A′1), 70.77 (C-A′2), 70.60 (C-4′′), 68.73 (C-5′′′), 67.56 (C-6′′), 67.17 (C-A), 62.48 (C-B), 60.00 (C-B′1), 59.95 (C-B′2), 33.82 (CH2), 31.06 (CH2), 24.58 (CH2), 22.24 (CH2), 18.15 (C-6′′′), 14.23 (CH3).
2.4.7 Troxerutin monooctyl ester (TMO).
13C-NMR (600 MHz, DMSO-d6) δ 177.97 (C-4), 173.34 (C
O), 164.51 (C-7), 161.41 (C-9), 157.11 (C-5), 156.86 (C-2), 151.36 (C-4′), 147.98 (C-3′), 134.16 (C-3), 122.99 (C-1′), 122.79 (C-6′), 114.76 (C-5′), 113.19 (C-2′), 105.71 (C-10), 101.76 (C-1′′), 101.38 (C-1′′′), 98.77 (C-6), 93.46 (C-8), 76.83 (C-3′′), 76.41 (C-5′′), 74.60 (C-2′′), 72.21 (C-4′′′), 71.07 (C-3′′′), 70.99 (C-2′′′), 70.78 × 2 (C-A′1, C-A′2), 70.58 (C-4′′), 68.72 (C-5′′′), 67.52 (C-6′′), 67.19 (C-A), 62.45 (C-B), 60.00 (C-B′1), 59.95 (C-B′2), 33.88 (CH2), 31.61 (CH2), 28.98 (CH2), 28.85 (CH2), 24.97 (CH2), 22.49 (CH2), 18.14 (C-6′′′), 14.35 (CH3).
2.4.8 Troxerutin monodecyl ester (TMD).
13C NMR (600 MHz, DMSO-d6) δ 177.66 (C-4), 173.36 (C
O), 164.41 (C-7), 162.84 (C-9), 157.15 (C-5), 156.57 (C-2), 151.21 (C-4′), 147.93 (C-3′), 134.36 (C-3), 122.91 (C-1′), 122.86 (C-6′), 114.70 (C-5′), 113.15(C-2′), 106.22 (C-10), 101.87 (C-1′′), 101.39 (C-1′′′), 98.98 (C-6), 92.59 (C-8), 76.94 (C-3′′), 76.42 (C-5′′), 74.62 (C-2′′), 72.26 (C-4′′′), 71.10 (C-3′′′), 70.98 (C-2′′′), 70.74 (C-A′1, C-A′2), 70.49 (C-4′′), 68.72 (C-5′′′), 67.45 (C-6′′), 67.04 (C-A), 62.50 (C-B), 59.95 (C-B′1), 59.91 (C-B′2), 33.88 (CH2), 31.72 (CH2), 29.32 (CH2), 29.17 (CH2), 29.10 (CH2), 28.84 (CH2), 24.92 (CH2), 22.53 (CH2), 18.18 (C-6′′′), 14.38(CH3).
2.5 Determination of the lipophilicity of troxerutin ester derivatives
The shake flask method18 was employed to determine the 1-octanol/water partition coefficient (log
P), a commonly used parameter for assessing the lipophilicity of compounds. Briefly, equal volumes of 1-octanol and water were thoroughly mixed and pre-saturated for 24 h. Excess TX or its derivatives were then added to the water-saturated 1-octanol (2 mL) until visible precipitation occurred. After centrifugation, the supernatant (1 mL) was collected and mixed with an equal volume of 1-octanol-saturated water before being incubated in a thermostatic shaker (37 °C, 180 rpm) for another 24 h. Samples were collected from the 1-octanol and water layers for HPLC analysis, and log
P was calculated using the following equation: | Log P = log (Coil/Cwater) | (5) |
where Coil (mmol L−1) represents the concentration of TX or its ester derivatives in 1-octanol, and Cwater (mmol L−1) represents their concentration in water.
2.6 Measurement of Caco-2 cytotoxicity
We referred to the method of Zhang Z. et al.,19 and made some minor modifications. Caco-2 cells were cultured in DMEM supplemented with 1% penicillin–streptomycin and 10% FBS, and maintained in an incubator at 37 °C and 5% CO2. 100 μL of cells were seeded into each well of a 96-well microplate at a density of 1 × 104 cells per well. After 24 h of culture, the Caco-2 cells reached confluence state and were treated with TX or its derivatives at a defined concentration (0–1000 μmol L−1) for another 24 h. Then, after 1 h of treatment with the cell counting kit-8 (CCK-8) solution (10 μL), the absorbance of each well was detected at 450 nm (OD450). Negative controls were included. The cell viability (C%) was calculated using the following formula:where Ct and Cn represent the OD450 values of tested samples and negative controls, respectively.
2.7 Measurement of Caco-2 cellular uptake
Initially, 100 μL of well cultured cells were seeded into each well of a 6-well microplate at a density of 1 × 104 cells per well for 24 h until confluence was reached.20 Subsequently, TX and its esters were dissolved in DMSO and diluted accordingly to a concentration 100 μmol L−1 in DMEM (DMSO conc. <1‰), then the culture medium was replaced with DMEM containing TX or its esters for a duration of 2 h before rinsing with PBS. Afterwards, the cells were digested with trypsin, and were centrifuged to obtain the cell pellets. Then, 0.1% Triton X-100 was used to lyse the cells, followed by centrifugation to obtain the cell contents for HPLC analysis. The cellular uptake was calculated using the following formula: | Cellular uptake (%) = n1/n0 × 100 | (7) |
where n1 and n0 represent the amounts of compounds in the cell lysate and initial cell culture medium, respectively.
2.8 Determination of in vitro gastrointestinal digestive stability of troxerutin esters
To measure the gastrointestinal digestive stabilities of TX ester derivatives in vitro, the INFOGEST method established by André Brodkorb et al.21 was adopted. The in vitro gastrointestinal digestive behavior of TX and its derivatives was divided into two phases: (i) gastric digestion and (ii) small intestinal digestion.
During gastric digestion, simulated gastric fluid (SGF) was prepared by adjusting 2 mg mL−1 NaCl solution to pH 3.0 and subsequently adding pepsin (2000 U mL−1). TX or its esters (500 μmol L−1) were added to SGF (5 mL), and the pH was adjusted to 3.0 before incubating the mixture in a thermostatic incubator (37 °C, 180 rpm) for 2 h.
Similarly, simulated intestinal fluid (SIF) was prepared to evaluate small intestinal digestion. SIF contained NaCl (8.8 mg mL−1), KH2PO4 (6.8 mg mL−1), bile powder (10 mg mL−1) and trypsin (100 U mL−1) adjusted to pH 7.2. Then 3.5 times concentrated SIF (2 mL) was added into the gastric digestion product (adjusted to pH 7.2 with NaOH). The mixture was incubated in a thermostatic incubator (37 °C, 180 rpm) to simulate digestion for another 2 h.
Sampling and analysis were carried out at defined intervals to determine the yield of TX on total ester hydrolysis and to study the evolution of the hydrolysis process. To determine the TX yield after ester hydrolysis, the simulated digestive mixture (500 μL) was diluted 3-fold with methanol. After centrifugation (16
000g, 15 min), the supernatant (20 μL) was taken for HPLC analysis.
The yield of TX after ester hydrolysis (Y%), expressed by the degree of conversion of troxerutin ester derivatives into TX, was calculated as follows:
where
CTX is the concentration of TX after troxerutin derivative hydrolysis, and
C is the concentration of the starting esters.
To measure the evolution of the troxerutin derivatives hydrolysis process, the intestinal digestive reactions were terminated at 10, 20, 30, 60 and 120 min. Subsequently, the samples were processed using the above method.
2.9 Statistical analysis
Sampling was carried out in triplicate and the experimental results were presented as mean ± standard deviation (SD). Using SPSS Statistic 19.0 software, the statistical significance was determined by one-way ANOVA and Duncan test. P values less than 0.05 were considered statistically significant.
3. Results and discussion
3.1 Regioselectivity of different lipases towards the acylation of troxerutin
To identify lipases with TX acylation activity, the behavior of eight microbially derived lipases was evaluated (Table 1). The blank control showed that acylation did not take place in the absence of the lipase. Among the 8 lipases tested, only Novozym 435, Lipozyme TL IM, Lipase PS IM and Lipozyme 40086 exhibited catalytic activity towards TX acylation. Notably, the initial rate attained by Novozym 435 was 20–30 times higher than that of other lipases. Most lipases possess a hydrophobic pocket that is obstructed by a polypeptide chain known as the “lid”, which isolates the active center from the reaction medium. Only when the lid is displaced by an oil–water interface can the lipase exhibit catalytic activity. However, Novozym 435 only has a small lid which cannot fully isolate the active center,22 resulting in a faster catalytic reaction rate. In addition, Novozym 435 has broad specificity for substrates with large groups attached to carboxyl or hydroxyl sites. Therefore, based on its interface activation properties and broad substrate specificity, Novozym 435 exhibits higher catalytic activity than other lipases in most cases.
Table 1 Comparison of troxerutin acylation catalyzed by different enzymes
Type of enzyme |
Time (h) |
Initial rate (mmol L−1 h−1) |
Conversion (%) |
Product |
Regioselectivity (%) |
Experiments were performed in triplicate, and data were presented as the mean ± SD. NA = no activity. |
None |
24 |
0 |
0 |
NA |
0 |
Lipase AY 30 |
24 |
NA |
NA |
NA |
NA |
Lipase 100T |
24 |
NA |
NA |
NA |
NA |
Lipase from Aspergillus oryzae |
24 |
NA |
NA |
NA |
NA |
Lipase A |
24 |
NA |
NA |
NA |
NA |
Lipase PS IM |
24 |
12.1 ± 0.45 |
89.9 ± 0.03 |
TMP |
96.6 ± 0.05 |
Lipozyme 40086 |
24 |
9.68 ± 0.71 |
90.3 ± 1.05 |
TDP |
58.4 ± 0.85 |
TTP |
38.5 ± 2.13 |
Lipozyme TL IM |
24 |
7.56 ± 0.19 |
62.2 ± 0.08 |
TTP |
88.6 ± 0.17 |
Novozym 435 |
1 |
280 ± 3.28 |
98.1 ± 0.57 |
TDP |
47.9 ± 1.25 |
TEP |
6.94 ± 0.02 |
Fig. 1 showed the HPLC results of the propionylation of TX catalyzed by Novozym 435, Lipozyme TL IM, Lipase PS IM and Lipozyme 40086. Interestingly, when lipases of different origin were employed, the resulting chromatographic peaks differed in number, retention time and integral area. This meant that various lipases may exhibit different selectivities towards TX, leading to various products with differing yields. Lipase PS IM gave only one product peak with a retention time of 6.64 min. The products were identified by HRMS, FT-IR, and 13C-NMR (ESI Fig. S4–S6†). HRMS showed that the molecular weight of the product with a retention time of 6.64 min was 821.2508 [(M + Na)+, m/z], suggesting that it was troxerutin monopropyl ester (TMP). In the FT-IR analysis, new peaks appeared at 2975 and 1737 cm−1 compared with TX, which was attributed to the C–H and C
O characteristic peaks and further confirmed the introduction of a fatty chain on TX through ester bonds. To identify the acylation site of TX, 13C-NMR spectra showed that the mono-acylation of CB–OH led to an over 2 ppm upfield shift of C-B and a downfield shift of C-A compared to TX (ESI Table S1†). Thus, the product with the retention time of 6.64 min was TMP, and the acylation site was the hydroxyl group of the A-ring hydroxyethyl group. Hence, Lipase PS IM showed high chemo- and regioselectivity (96.6%) for the troxerutin mono-ester.
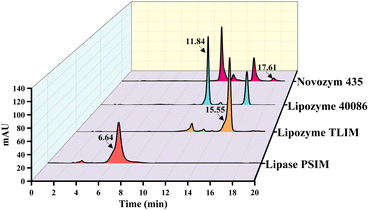 |
| Fig. 1 HPLC chromatogram of troxerutin with vinyl propionate catalyzed by different lipases. | |
Lipozyme 40086 and Lipozyme TL IM gave two ester derivatives with retention times of 11.84 and 15.55 min, identified as troxerutin dipropyl (TDP) and tripropyl (TTP) esters (ESI Fig. S7–S12†), respectively. However, selectivities differed for these two enzymes. Lipozyme TL IM provided TTP with a high selectivity of 88.6%, but Lipozyme 40086 showed a lower 38.5% selectivity towards TTP while it showed 58.4% selectivity towards TDP.
After just 1 h of reaction, Novozym 435 catalyzed the acylation of TX to produce three products including TDP, TTP, and the tetrapropyl ester (TEP), the latter having a retention time of 17.61 min. This indicated that Novozym 435 had the potential to catalyze the multi-site acylation of TX. It was also found that changing the concentration of Novozym 435 and the reaction time affected the regioselectivity, forming different ester derivatives. This indicated that it may be possible to achieve efficient and highly regioselective syntheses of TDP and TEP by varying the key parameters of the Novozym 435-mediated acylation.
Overall, among the lipases tested, only Novozym 435, Lipozyme TL IM, Lipase PS IM and Lipozyme 40086 exhibited catalytic activity towards TX acylation, with varying regioselectivities. Under the reaction conditions of this experiment, Lipozyme PS IM was selective for troxerutin mono-ester, Lipozyme TL IM for the triester, and Novozym 435 for the di- and tetraesters. Lipozyme 40086 can synthesize both diesters and triesters, but its transformation efficiency was lower than that of Novozym 435 for the diester and Lipozyme TL IM for the triester.
3.2 Efficient and controlled lipase-mediated synthesis of troxerutin esters with different structures
Before we proceeded with the acylation of TX with different chain-length acyl donors, the hydrophilic/lipophilic balance and the bioavailability of the TX esters were evaluated firstly by theoretically calculating their log
P. Log
P is a key physical parameter widely employed to assess the hydrophilic/lipophilic balance and the bioavailability of xenobiotics.11 Studies have shown that oral small-molecule drugs can be predicted to have optimal intestinal permeability when their log
P values were in the range of 0–3.23 The log
P values of ester derivatives of TX with different chain lengths and acylation sites were calculated using ChemBioDraw software. The esters with calculated log
P (Clog
P) values in the range of 0–3 were shown to be TDP, TTP, TEP, TDB, TMH, TMO and TMD, with Clog
P values of 0.36, 1.78, 2.51, 1.42, 0.52, 1.58, and 2.63, respectively.
Then, the effects of three key reaction parameters (substrate molar ratio, reaction time and enzyme loading) on the propionylation of TX with VP were investigated by using Novozym 435, Lipozyme TL IM and Lipozyme PS IM as the catalysts (Fig. 2). Many studies have reported that increasing the concentration of the acyl donor facilitated the reaction, generating a higher quantity of acylated derivatives.24,25 As shown in Fig. 2A1–2D1, TX conversion reached more than 90% for all the ester derivatives. The regioselectivity and yield of TMP and TDP increased and then decreased with increasing acylation donor stoichiometry, with maximum values at the molar substrate: VP ratios of 1
:
20 and 1
:
15, respectively. The use of a larger excess of acyl donor VP favored over-acylation at additional acylation sites, resulting in decreased regioselectivity and yield. The regioselectivity and yield of TTP and TEP increased with increasing VP concentration, with maximum values at substrate: VP ratios of 1
:
50–1
:
60. The higher reactant requirement for TTP and TEP was a natural consequence of their higher degree of acylation and the increasing steric challenged to occupy more acylation sites. Hence, higher acyl donor stoichiometry enabled the generation of multi-site TX esters, confirming our previous results.6
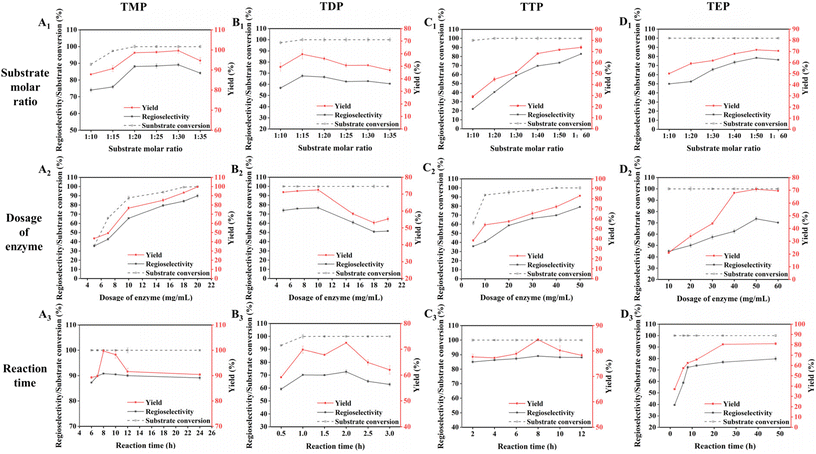 |
| Fig. 2 Effects of key reaction conditions on the synthesis of troxerutin propyl ester with different acylation sites. (A1–D1): effect of substrate molar ratio on the synthesis of TMP, TDP, TTP, and TEP; (A2–D2): effect of dosage of enzyme on the synthesis of TMP, TDP, TTP, and TEP; (A3–D3): effect of reaction time on the synthesis of TMP, TDP, TTP, and TEP. | |
Fig. 2A2–D2 showed the effect of lipase dosage on TX acylation. For Lipase PS IM and Lipozyme TL IM, the yield and regioselectivity towards TMP and TTP increased with increased enzyme loading (5–20 mg mL−1 for Lipozyme PS IM and 5–50 mg mL−1 for Lipozyme TL IM) (Fig. 2A2, C2). This was expected as a higher lipase concentration would provide more active sites for the formation of acyl-enzyme intermediates. For Novozym 435, the enzyme loading for optimal yield and regioselectivity differed for TDP and TEP. TDP was quickly generated using only 10 mg mL−1 of Novozym 435, with a satisfactory 100% conversion, 72.5% yield, and 76.9% regioselectivity in only 2 h (Fig. 2B2). When the loading of Novozym 435 was increased to 50 mg mL−1, TEP was selectively generated with 100% conversion, 70.8% yield and 73.6% regioselectivity in 24 h. With increasing reaction time, the yield of TMP, TDP and TTP increased and then decreased (Fig. 2A3–D3). This can be attributed to over-acylation, leading to multi-esters at additional acylation sites as the reaction time was extended. For the synthesis of TEP, there was no obvious difference in the yield (80.3%) after 24 h.
The optimal reaction conditions were then applied for the acylation of TX with longer alky chain-length vinyl esters. And the desired ester products with Clog
P of 0–3 were synthesized selectively with high yields (Table 2). Fig. 3 exhibited the recognition properties of the different lipases towards TX, as well as the synthesis pathways of seven ester derivatives possessing varying chain lengths and acylation sites. Lipozyme PS IM and Lipozyme TL IM can be employed as efficient catalysts for producing the mono- and triester, respectively, while Novozym 435 was the best choice for the production of the di- and tetraesters of TX.
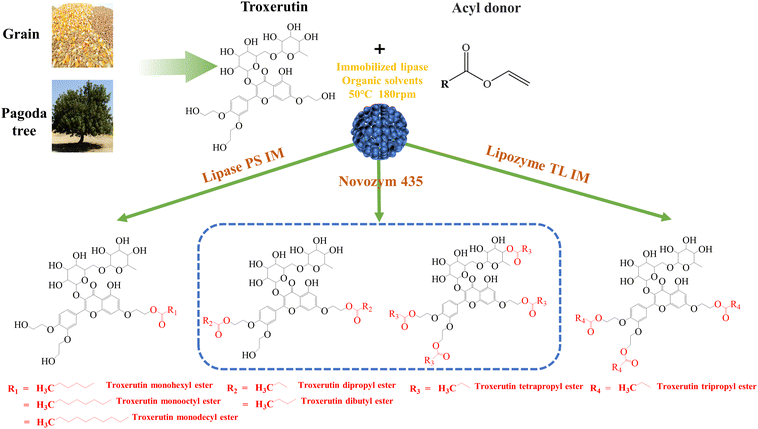 |
| Fig. 3 General scheme of lipase-mediated troxerutin acylation. | |
Table 2 The optimal conditions for enzymatic acylation of troxerutin with different acyl donors and the product yields
Acyl donor |
Molar ratio of substrate (mol : mol) |
Enzyme |
Enzyme loading (mg mL−1) |
Time (h) |
Acylated product |
Yield (%) |
Experiments were performed in triplicate, and data were presented as the mean ± SD. |
VP |
1 : 15 |
Novozym 435 |
10 |
2 |
TDP (di-ester) |
72.5 ± 0.33 |
VP |
1 : 60 |
Lipozyme TL IM |
50 |
8 |
TTP (tri-ester) |
84.4 ± 0.59 |
VP |
1 : 50 |
Novozym 435 |
50 |
24 |
TEP (tetra-ester) |
80.4 ± 1.32 |
VB |
1 : 15 |
Novozym 435 |
10 |
2 |
TDB (di-ester) |
71.8 ± 0.08 |
VH |
1 : 20 |
Lipase PS IM |
20 |
10 |
TMH (mono-ester) |
98.8 ± 0.01 |
VO |
1 : 20 |
Lipase PS IM |
20 |
10 |
TMO (mono-ester) |
99.5 ± 0.01 |
VD |
1 : 20 |
Lipase PS IM |
20 |
12 |
TMD (mono-ester) |
99.3 ± 0.08 |
3.3 The lipophilicity of troxerutin and its esters
The lipophility of TX and its ester derivatives was further assessed by measuring their log
P values. As shown in Fig. 4, log
P for TX in this study was −2.27, which was similar to the reported value of −2.04,6 and reflected its poor intestinal absorption. The log
P values for the seven troxerutin ester derivatives were all higher than that of TX, measuring 0.53, 1.83, 2.37, 1.48, 0.64, 1.67, and 2.68, respectively, which were close to the Clog
P values (Fig. 4). Notably, the log
P values for these ester derivatives were all in the range of 0–3, indicating the potential for better intestinal absorption.
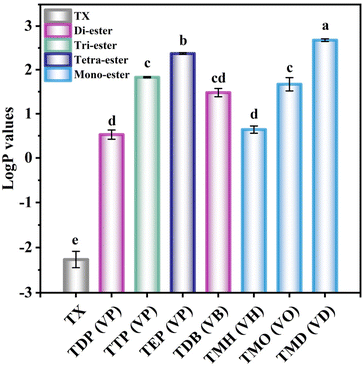 |
| Fig. 4 The log P values of troxerutin and its esters. Values with different letters (a, b, c, d, and e) in the assay showed significant differences from one another (p < 0.05) in a multiple-range analysis. | |
Previous studies mainly focused on the influence of the length of the fatty acid chains attached to flavonoids on their lipophilicity. Here, we showed the relationships between the lipophilicity of TX esters and the numbers/length of aliphatic acyl groups introduced to TX. It was found that introducing a number of short-chain aliphatic acyl groups had a similar effect on the increase in lipophilicity to introducing a single long-chain aliphatic acyl group. For example, the introduction of two propionyl groups (2 × CH3CH2CO-, 0.53) and the introduction of one hexanoyl group (CH3(CH2)4CO-, 0.64) resulted in similar log
P values. Likewise, the log
P values of three propionyl groups (3 × CH3CH2CO-, 1.83) and two butyl groups (2 × CH3(CH2)CO-, 1.48) were similar to that of one octyl group (CH3(CH2)5CO-, 1.67). The introduction of four propionyl groups (4 × CH3CH2CO-, 2.37) and one decyl group (CH3(CH2)7CO-, 2.68) also gave similar log
P values.
3.4 Cytotoxicity of troxerutin and its esters
Previous studies have shown that the introduction of medium-long fatty chains through mono-acylation can increase cytotoxicity compared to the parent compound, but multi-site acylation with short fatty chains can mitigate this effect.13 In a human intestinal cell Caco-2 model, the toxicity of TX and its esters with different fatty chain lengths and acylation sites at concentrations of 0–1000 μmol L−1 was evaluated using the CCK-8 assay. When the cell viability is higher than 90%, the compound can be considered non-toxic to cells at a certain concentration.26 As depicted in Fig. 5, the cytotoxicity of both TX and its ester derivatives towards Caco-2 cells increased in a concentration-dependent manner. At concentrations above 125 μmol L−1, TX exhibited toxic effects on Caco-2 cells. For the mono-ester derivatives, the cytotoxicity of TMH, TMO, and TMD was higher than that of TX, and their cell viabilities at 1000 μmol L−1 were only 57.4%, 39.8%, and 34.5%, respectively. These results supported previous reports that the introduction of medium-long fatty chains increased the cytotoxicity of the parent compound. This was mainly due to the fact that the medium length fatty chain will disrupt the cell membrane structure, leading to the leakage of the cell contents.27–29
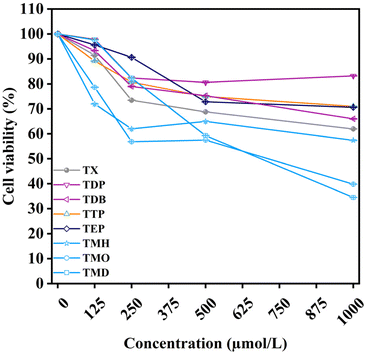 |
| Fig. 5 Cytotoxicity of troxerutin and its esters towards Caco-2 cells. | |
However, esters bearing short fatty chains on multi-site acylation including TDP, TTP, TEP and TDB exhibited lower toxicity than TX. The cytotoxicity of TDP to Caco-2 was the lowest at 1000 μmol L−1, maintaining at least 83.2% cell viability. This was consistent with results reported by Bala et al. that the cytotoxicity of ester derivatives with poly-acylation sites was lower than that of the parent compounds and their mono-acylation products.13 Hence, short fatty chains appeared to mitigate the disruption of the cell membrane structures, and multi-site acylation conferred an increased steric hindrance. Thus, from the perspective of cytotoxicity, multi-site acylation was more advantageous than mono-acylation.
3.5 Cellular uptake of troxerutin and its esters
To assess the membrane-permeating ability of troxerutin esters with different fatty chain lengths and acylation sites, the accumulation of TX and its esters in Caco-2 cells was investigated. According to the cytotoxicity results, TX and its esters at a concentration of 100 μmol L−1 did not exhibit cytotoxic effects, so this concentration was used for the cellular uptake assay. As depicted in Fig. 6, 0.25% accumulation of TX in Caco-2 cells was extremely low, confirming its poor intestinal absorption.7,30,31 After acylation, the intracellular accumulation of troxerutin esters was higher than that of TX, in decreasing order as follows: TEP (10.15%) > TDB (0.78%) > TMD (0.63%) > TDP (0.62%) > TTP (0.58%) ≈ TMO (0.58%) > TMH (0.56%). These percentages indicated improved membrane penetration and accumulation of troxerutin esters in cells. The accumulation of multi-esters at multiple acylation sites was higher than that of mono-ester derivatives. In particular, the accumulation of TEP was 40.6 times higher than that of TX, indicating that enhanced lipophilicity improved cell membrane penetration, increasing ester molecule concentration within the cell.
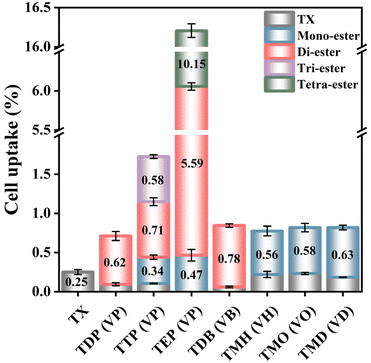 |
| Fig. 6 Cellular uptake of troxerutin and its esters in Caco-2 cells. | |
It was noteworthy that when Caco-2 cells were treated with multi-ester derivatives, TX and lower acylated esters were also detected. For example, a certain amount of TX, TMP, and TDP were also detected in the TTP-treated group, indicating the existence of hydrolases in the cell (such as lipase and carboxylesterases) that can hydrolyze ester bonds to generate derivatives with a lower acylation degree and TX.28 Furthermore, ester derivatives with more acylation sites were difficult to hydrolyze into TX, possibly due to steric hindrance, thus increasing their cellular accumulation (Fig. 6). For example, the percentage of TX in Caco-2 cells from the hydrolysis of TDP, TTP and TEP were 0.1, 0.1, and 0%, respectively. Despite being hydrolyzed, the membrane-permeating ability of these esters was still higher than that of TX. Therefore, the total amount of TX and its esters in cells treated with multi-esters was much higher than that of TX-treated cells.
Notably, the lipophilicities of TMO (log
P = 1.67) and TMD (log
P = 2.68) were similar to those of TTP (log
P = 1.83) and TEP (log
P = 2.37), but the cellular accumulation of TMO and TMD was lower than that of TTP and TEP. A possible explanation for this was that mono-esters with medium-long fatty chains were more susceptible to hydrolysis to TX (supported by the accumulation of TX produced by hydrolysis), and inserted into the membrane's phospholipid bilayer, preventing entry into the cell. In short, multi-esters with multiple acylation sites underwent less conversion into the parent compound during uptake by Caco-2 cells. Hence, it can be inferred that, for ester derivatives with similar lipophilicity, esters with multiple acylation sites showed more obvious advantages than mono-esters at a single acylation site.
3.6 Gastrointestinal digestive stability of troxerutin ester
The stability of troxerutin esters in simulated gastrointestinal digestion was determined to predict their stability after oral administration. As depicted in Fig. 7, after 4 h of in vitro simulated gastrointestinal digestion, all troxerutin esters were hydrolyzed by pancreatic lipases present in the intestine.32–34 However, the yields of TX after ester hydrolysis differed. For the TX mono-ester with one acylation site, the yield of TX increased with increasing fatty chain length (C6–C10), with conversions of 61.6%, 81.9%, and 89.2%, respectively. Interestingly, when TX was modified with multiple acylation, the rate of hydrolysis into TX significantly decreased with an increasing number of acylation sites. For example, the yield of TX from the hydrolysis of TDP, TTP, and TEP decreased successively (61.5%, 26.9% and 15.8%, respectively). This phenomenon can be attributed to increased steric hindrance caused by multi-site acylation, making enzymatic ester hydrolysis more challenging.
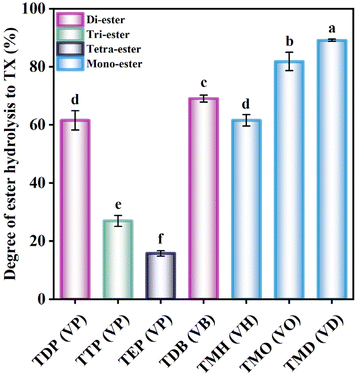 |
| Fig. 7 Conversion degree to TX by hydrolysis of troxerutin esters during in vitro gastrointestinal simulated digestion. Values with different letters (a, b, c, d, e, and f) in the assay showed significant differences from one another (p < 0.05) in a multiple-range analysis. | |
To reveal the differences in the digestive processes of the various esters in this study, the hydrolysis process was studied for 2 h of intestinal digestion. Fig. 8 showed the HPLC chromatograms for the esters after 0, 10, 20, 30, 60 and 120 min of simulated intestinal digestion. During intestinal digestion, multi-esters were hydrolyzed into esters with fewer acylation sites, and eventually to TX, while mono-acylation esters were directly hydrolyzed to TX. For example, after 10 min, TDP was hydrolyzed into TMP and a metabolite with a retention time of 3.7 min, which was inferred to be a mono-ester acylated at a different site to TMP from LC-MS results (Fig. S28†). This was then converted into TX on further digestion (Fig. 8A). Similarly, TTP was gradually hydrolyzed to its diester, mono-ester, and TX, while TEP was gradually hydrolyzed to its triester, diester, and mono-ester. Notably, most TEP was hydrolyzed to mono-ester and diester instead of TX after 2 h of digestion, highlighting the advantage of multi-site acylation.
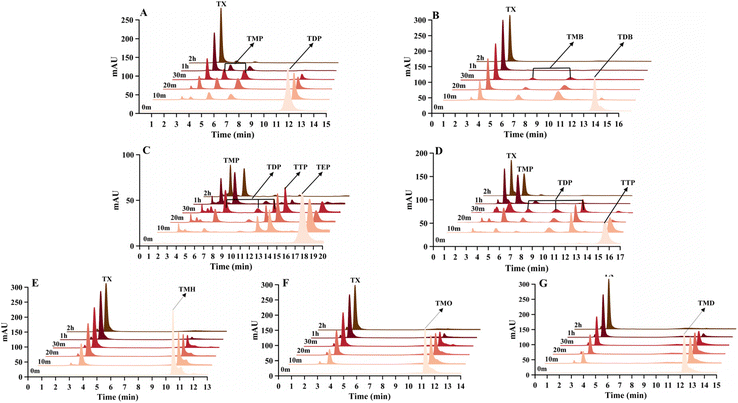 |
| Fig. 8 Hydrolysis process diagram of troxerutin esters (A–G showed the hydrolysis process diagrams of TDP, TTP, TEP, TDB, TMH, TMO, and TMD, respectively). | |
As is well known, intestinal absorption and digestion are mutually reinforcing and closely related processes. While being hydrolyzed, esters also undergo intestinal absorption through transmembrane transport, and if TX can remain in the gastrointestinal tract for a longer period in the form of its ester, it would be more beneficial for its absorption. Comparing multi- and mono-esters (Fig. 8A–D and E–G), the latter were completely hydrolyzed into TX after 1 h, while those with multiple acylation sites can be retained in the intestine for longer periods, which may lead to more absorption. Overall, esters with multiple acylation sites were more gradually hydrolyzed into lower acylated esters and TX during intestinal digestion, extending the retention time of troxerutin in the intestine, and confirming the advantage of flavonoid multi-ester derivatives with multiple acylation sites.
4. Conclusion
In this study, a series of TX ester derivatives with different fatty chain lengths and acylation sites were efficiently and selectively synthesized using a facile lipase strategy with high regioselectivity and yield. The high efficiency and controllable regioselectivity of this method depended on the specific lipase used and the regulation of key reaction parameters. The lipophilicity of the ester derivatives was related to the length and number of fatty chains introduced. Strikingly, it was found that for esters with similar lipophilicity, those with multiple acylation sites had lower cytotoxicity and conferred higher cellular accumulation and better digestive absorption characteristics than mono-esters. These results confirm the advantages of controlled multi-site acylation of TX, and provide a new insight into the acylation strategy for the development of food and pharmaceuticals.
Author contributions
Li-sha Hao: conceptualization, methodology, data curation, investigation, formal analysis, writing – original draft, and visualization. Meng-meng Zhang: conceptualization, methodology, data curation, and writing – review and editing. Xiao-feng Li: conceptualization, methodology, data curation, resources, writing – review and editing, and funding acquisition. Xuan Xin: conceptualization, methodology, writing – review and editing, and funding acquisition. Guang-lei Zhao: supervision, project administration, and funding acquisition.
Conflicts of interest
There are no conflicts to declare.
Acknowledgements
This work was supported by the Open Project Program of the Provincial Key Laboratory of Green Processing Technology and Product Safety of Natural Products (202212), National Natural Science Foundation of China (22278159), GuangDong Basic and Applied Basic Research Foundation (2022A1515110710), and Science and Technology Projects in Guangzhou (2023A04J0745).
References
- N. A. Panat, D. K. Maurya, S. S. Ghaskadbi and S. K. Sandur, Troxerutin, a plant flavonoid, protects cells against oxidative stress-induced cell death through radical scavenging mechanism, Food Chem., 2016, 194, 32–45 CrossRef CAS PubMed.
- N. S. Thomas, K. George and A. A. A. Selvam, Troxerutin subdues hepatic tumorigenesis via disrupting the MDM2-p53 interaction, Food Funct., 2018, 9, 5336–5349 RSC.
- X. H. Xue, Y. T. Chen, Y. Wang, J. D. Zhan, B. Chen, X. Y. Wang and X. Y. Pan, Troxerutin suppresses the inflammatory response in advanced glycation end-product-administered chondrocytes and attenuates mouse osteoarthritis development, Food Funct., 2019, 10, 5059–5069 RSC.
- X. Q. Wang, Y. Z. Gao, L. Wang, D. Yang, W. Bu, L. S. Gou, J. J. Huang, X. Y. Duan, Y. Pan, S. Y. Cao, S. Y. Gao, C. Cheng, Z. J. Feng, J. Xie and R. Q. Yao, Troxerutin Improves Dextran Sulfate Sodium-Induced Ulcerative Colitis in Mice, J. Agric. Food Chem., 2021, 69, 2729–2744 CrossRef CAS PubMed.
- H. Peng and F. Shahidi, Metabolic, toxicological, chemical, and commercial perspectives on esteri-fication of dietary polyphenols: a review, Crit. Rev. Food Sci. Nutr., 2023, 1–40 CrossRef PubMed.
- X. Xin, M. M. Zhang, X. F. Li, F. R. Lai and G. L. Zhao, Biocatalytic synthesis of acylated derivatives of troxerutin: their bioavailability and antioxidant properties in vitro, Microb. Cell Fact., 2018, 17, 130 CrossRef PubMed.
- M. Xu, Q. Yu, Q. R. Zhao, W. Chen, Y. J. Lin and Y. Jin, Development and in vitro-in vivo evaluation of a water-in-oil microemulsion formulation for the oral delivery of troxerutin, Drug Dev. Ind. Pharm., 2016, 42, 280–287 CrossRef CAS PubMed.
- Ziaullah, K. S. Bhullar, S. N. Warnakulasuriya and H. P. V. Rupasinghe, Biocatalytic synthesis, struct-ural elucidation, antioxidant capacity and tyrosinase inhibition activity of long chain fatty acid acylated derivatives of phloridzin and isoquercitrin, Bioorg. Med. Chem., 2013, 21, 684–692 CrossRef CAS PubMed.
- J. H. Salem, C. Humeau, I. Chevalot, C. Harscoat-Schiavo, R. Vanderesse, F. Blanchard and M. Fick, Effect of acyl donor chain length on isoquercitrin acylation and biological activities of corresponding esters, Process Biochem., 2010, 45, 382–389 CrossRef CAS.
- L. Mo, G. L. Zhao, X. F. Li, X. L. Xiao, N. He, J. J. Ma and Y. G. Yu, Evaluation of the digestion and transport profiles and potential immunocompetence of puerarin and its acylated derivatives, Food Funct., 2021, 12, 5949–5958 RSC.
- A. S. Marquez-Rodriguez, M. Guimaraes, N. Mateus, V. De Freitas, M. L. Ballinas-Casarrubias, M. E. Fuentes-Montero, E. Salas and L. Cruz, Disaccharide anthocyanin delphinidin 3-O-sambubioside from Hibiscus sabdariffa L.: Candida antarctica lipase B-catalyzed fatty acid acylation and study of its color properties, Food Chem., 2021, 344, 128603 CrossRef CAS PubMed.
- D. M. Pereira, T. C. Silva, S. Losada-Barreiro, P. Valentao, F. Paiva-Martins and P. B. Andrade, Toxi-city of phenolipids: Protocatechuic acid alkyl esters trigger disruption of mitochondrial membrane potential and caspase activation in macrophages, Chem. Phys. Lipids, 2017, 206, 16–27 CrossRef CAS PubMed.
- V. Bala, S. S. Rao, P. Li, S. D. Wang and C. A. Prestidge, Lipophilic Prodrugs of SN38: Synthesis and in Vitro Characterization toward Oral Chemotherapy, Mol. Pharm., 2016, 13, 287–282 CrossRef CAS PubMed.
- M. E. M. B. de Araujo, Y. E. M. Franco, M. C. F. Messias, G. B. Longato and J. A. Pamphile, Biocatalytic Synthesis of Flavonoid Esters by Lipases and Their Biological Benefits, Planta Med., 2017, 83, 7–22 CAS.
- Y. M. Xiao, L. R. Yang, P. Mao, Z. Zhao and X. F. Lin, Ultrasound-promoted enzymatic synthesis of troxerutin esters in nonaqueous solvents, Ultrason. Sonochem., 2011, 18, 303–309 CrossRef CAS PubMed.
- Y. M. Xiao, P. Mao, Z. Zhao, L. R. Yang and X. F. Lin, Regioselective enzymatic acylation of troxerutin in nonaqueous medium, Chin. Chem. Lett., 2010, 21, 59–62 CrossRef CAS.
- X. Xin, X. F. Li, X. L. Xiao, Y. Q. Tang and G. L. Zhao, Facile and Efficient Acylation of Bioflavonoids Using Whole-Cell Biocatalysts in Organic Solvents, ACS Sustainable Chem. Eng., 2017, 5, 10662–10672 CrossRef CAS.
- J. A. Rothwell, A. J. Day and M. R. A. Morgan, Experimental determination of octanol-water partition coefficients of quercetin and related flavonoids, J. Agric. Food Chem., 2005, 53, 4355–4360 CrossRef CAS PubMed.
- Z. D. Zhang, Q. Tao, L. X. Bai, Z. Qin, X. W. Liu, S. H. Li, Y. J. Yang, W. B. Ge and J. Y. Li, The Transport and Uptake of Resveratrol Mediated via Glucose Transporter 1 and Its Antioxidant Effect in Caco-2 Cells, Molecules, 2023, 28, 4569 CrossRef CAS PubMed.
- X. Zhang, Y. D. Xiao and Q. L. Huang, Investigation of cellular uptake and transport capacity of Cordyceps sinensis exopolysaccharide-selenium nanoparticles with different particle sizes in Caco-2 cell monolayer, Int. J. Biol. Macromol., 2024, 262, 130060 CrossRef CAS PubMed.
- A. Brodkorb, L. Egger, M. Alminger, P. Alvito, R. Assuncao, S. Ballance, T. Bohn, C. Bourlieu-Lacanal, R. Boutrou, F. Carrière, A. Clemente, M. Corredig, D. Dupont, C. Dufour, C. Edwards, M. Golding, S. Karakaya, B. Kirkhus, S. Le Feunteun, U. Lesmes, A. Macierzanka, A. R. Mackie, C. Martins, S. Marze, D. J. McClements, O. Ménard, M. Minekus, R. Portmann, C. N. Santos, I. Souchon, R. P. Singh, G. E. Vegarud, M. S. J. Wickham, W. Weitschies and I. Recio, INFOGEST static in vitro simulation of gastrointestinal food digestion, Nat. Protoc., 2019, 14, 991–1014 CrossRef CAS PubMed.
- C. Ortiz, M. L. Ferreira, O. Barbosa, J. C. S. dos Santos, R. C. Rodrigues, A. Berenguer-Murcia, L. E. Briand and R. Fernandez-Lafuente, Novozym 435: the “perfect” lipase immobilized biocatalyst?, Catal. Sci. Technol., 2019, 9, 2380–2420 RSC.
- S. D. Kramer, Absorption prediction from physicochemical parameters, Pharm. Sci. Technol. Today, 1999, 2, 373–380 CrossRef CAS PubMed.
- C. Y. Jiang, L. Wang, X. Huang, S. Zhu, C. Y. Ma and H. X. Wang, Structural characterization and antioxidant property of enzymatic-transesterification derivatives of (-)-epigallocatechin-3-O-gallate and vinyl laurate, J. Food Sci., 2021, 86, 4717–4729 CrossRef CAS PubMed.
- L. S. Hao, M. M. Zhang, X. F. Li, X. Xin, F. L. Lei, X. N. Lai, G. L. Zhao and H. Wu, Highly efficient whole-cell biosynthesis and cytotoxicity of esculin esters, J. Biotechnol., 2021, 337, 46–56 CrossRef CAS PubMed.
- M. M. Zhang, X. Xin, G. L. Zhao, Y. C. Zou and X. F. Li, In vitro absorption and lipid-lowering activity of baicalin esters synthesized by whole-cell catalyzed esterification, Bioorg. Chem., 2022, 120, 105628 CrossRef CAS PubMed.
- M. M. Zhang, X. Xin, F. R. Lai, X. Y. Zhang, X. F. Li and H. Wu, Cellular Transport of Esculin and Its Acylated Derivatives in Caco-2 Cell Monolayers and Their Antioxidant Properties in Vitro, J. Agric. Food Chem., 2017, 65, 7424–7432 CrossRef CAS PubMed.
- S. Trier, L. Linderoth, S. Bjerregaard, T. L. Andresen and U. L. Rahbek, Acylation of Glucagon-Like Peptide-2: Interaction with Lipid Membranes and In Vitro Intestinal Permeability, PLoS One, 2014, 9, 109939 CrossRef PubMed.
- D. Sanver, A. Sadeghpour, M. Rappolt, F. DiMeo and P. Trouillas, Structure and Dynamics of Dioleoyl -Phosphatidylcholine Bilayers under the Influence of Quercetin and Rutin, Langmuir, 2020, 36, 11776–11786 CrossRef CAS PubMed.
- F. Liu, Y. Xu, L. Rui, S. Gao, H. J. Dong and Q. X. Guo, Liquid chromatography/tandem mass spectrometry assay for the quantification of troxerutin in human plasma, Rapid Commun. Mass Spectrom., 2006, 20, 3522–3526 CrossRef CAS PubMed.
- X. Y. Cui, M. L. Zhang, X. Guan, L. Yin, Y. T. Sun, J. P. Fawcett and J. K. Gu, LC-MS-MS, Determination of Troxerutin in Plasma and Its Application to a Pharmacokinetic Study, Chromatographia, 2011, 73, 165–169 CrossRef CAS.
- T. P. Sari, B. Mann, R. Kumar, R. R. B. Singh, R. Sharma, M. Bhardwaj and S. Athira, Preparation and characterization of nanoemulsion encapsulating curcumin, Food Hydrocolloids, 2015, 43, 540–546 CrossRef CAS.
- H. D. Silva, E. Beldíková, J. Poejo, L. Abrunhosa, A. T. Serra, C. M. M. Duarte, T. Brányik, M. A. Cerqueira, A. C. Pinheiro and A. A. Vicente, Evaluating the effect of chitosan layer on bioaccessibility and cellular uptake of curcumin nanoemulsions, J. Food Eng., 2019, 243, 89–100 CrossRef CAS.
- S. Acquistapace, L. Patel, A. Patin, E. Forbes-Blom, B. Cuenoud and T. J. Wooster, Effects of interesterified lipid design on the short/medium chain fatty acid hydrolysis rate and extent (in vitro), Food Funct., 2019, 10, 4166–4176 RSC.
Footnote |
† Electronic supplementary information (ESI) available: HRMS, FT-IR and 13C NMR spectra of TX and its esters (Fig. S1–S27). The LC-MS diagram of esters formed after simulated digestion in vitro (Fig. S28–S32). The chemical shift comparison of TX and its esters in 13C-NMR (Table S1). See DOI: https://doi.org/10.1039/d4fo00906a |
|
This journal is © The Royal Society of Chemistry 2024 |