DOI:
10.1039/D4FO00267A
(Paper)
Food Funct., 2024,
15, 7951-7960
Novel methodology to enrich medium- and short-chain fatty acids in milk fat to improve metabolic health†
Received
16th January 2024
, Accepted 16th June 2024
First published on 20th June 2024
Abstract
Dietary short- and medium-chain fatty acids have been shown to elevate circulating ketone bodies and confer metabolic health benefits. Cow milk fat contains these lipids in a balanced mix but in relatively low concentrations. Enriching them could amplify health benefits of dairy products. Here, we used a volatility-based workflow to produce milk fat with a 2-fold enrichment of medium- and short-chain fatty acids (referred to as MSFAT). Our proof-of-concept studies in mice demonstrated that intake of MSFAT increased circulating ketone bodies, reduced blood glucose levels, and suppressed food intake. In humans, ingestion of MSFAT resulted in increased circulating ketone bodies, trended to attenuate (p = 0.07) postprandial glucose excursion, and acutely elevated energy expenditure. Our findings show that milk products enriched with MSFAT may hold significant metabolic advantages.
1. Introduction
The ongoing obesity crisis, accompanied by its dire consequences such as the widespread prevalence of metabolic, lifestyle-related diseases, e.g. type 2 diabetes, could benefit from innovative, cost-effective, and accessible solutions for promoting metabolic health. This has spurred significant efforts to develop “functional foods”—foods providing health benefits beyond the basic nutritional value.1 These foods have the potential to influence metabolic health through changes in diet alone or in conjunction with pharmacological interventions. In this context, incorporation of special dietary fats such as short-chain fatty acids (SCFAs) and medium-chain fatty acids (MCFAs) into dairy products might help to induce weight loss and improve glycemic control in overweight people with insulin resistance and/or type 2 diabetes.2–8
SCFAs and MCFAs, defined as fatty acids with 2–6 and 8–12 carbon atoms in the acyl chain, respectively, have garnered significant attention for their potential benefits in inducing weight loss.2,4,5,9 SCFAs, such as acetate, propionate, and butyrate, play a significant role in appetite regulation by influencing the release of appetite-regulating hormones, such as glucagon-like peptide-1 (GLP-1) and peptide YY (PYY), leading to decreased food intake and increased feelings of satiety.2 Recently, it was shown that SCFA supplementation can effectively combat obesity in adolescents.3 On the other hand, MCFAs, like capric and caprylic acids, are readily converted by the liver to release energy and have been shown to boost thermogenesis, leading to increased calorie burning.4 Moreover, intake of MCFAs has also been repeatedly shown to lower food intake,5 an effect that might involve the induction of anorectic factors such as growth differentiation factor 15 (GDF15).9
Studies have also shown that SCFAs and MCFAs positively affect insulin sensitivity and glucose regulation.2,6,10 Notably, SCFAs, such as acetate, propionate, and butyrate, seem to enhance insulin signaling and glucose uptake in peripheral tissues,11 offering potential interventions for ameliorating insulin resistance. Similarly, MCFAs, including capric and caprylic acids, exhibit similar favorable effects on glucose metabolism, having been shown to improve glycemic control in subjects with type 2 diabetes.7
Characterized by their shorter carbon chain length, SCFAs and MCFAs have unique biochemical properties compared to long-chain fatty acids (LCFAs), which are the most abundant type of fatty acid (FA) in Western diet and the human body. For example, while dietary LCFAs enter the circulation via lymphatic absorption,12 SCFAs and MCFAs are directly absorbed into the portal vein, facilitating direct entry into the liver.13 This rapid delivery to and metabolism within the liver of SCFAs and MCFAs is thought to cause an excess production of acetyl-CoA that induces ketogenesis.13 In agreement, dietary intake of SCFAs and MCFAs has been shown to rapidly increase circulating ketone bodies.14,15 This is significant because ketone bodies, through receptor-dependent and independent mechanisms, have garnered the attention of the scientific community as potential therapeutics for cancer treatment, neurological, heart, and liver diseases, as well as for managing obesity-related and metabolic diseases.16
SCFAs are primarily produced through the fermentation of dietary fibers by the gut microbiota.17 MCFAs are abundant in coconut oil and palm kernel oil,18 which are not commonly consumed in the Western diet. Additionally, the typical Western diet often contains less dietary fiber than recommended.19 This presents an opportunity. Enriching Western diet food staples with these special dietary fats can increase the intake of SCFAs and MCFAs and convey their metabolic benefits to many. We sought to produce a cow milk product enriched in SCFAs and MCFAs. Cow milk naturally contains SCFAs and MCFAs in a balanced mix, but the concentrations of C4–12 FAs are relatively low, comprising 14–17% (∼15%) of the FAs in bovine milk,20 although there is considerable variability depending on season, feed and cow breed.21–23
To produce milk products with a high content of SCFAs and MCFAs, we selected raw milk from Jersey cows, because this breed produces milk naturally high in SCFA and MCFA content,21 and employed a volatility-based enrichment process for SCFAs and MCFAs, which we refined for large-scale production. The result is a novel milk fat, safe for human consumption, referred to as MSFAT, which contains a striking 100% increase in both SCFA and MCFA content. We conducted tests on mice and humans using MSFAT and obtained promising results for its efficacy to positively impact metabolic health. MSFAT demonstrated its efficacy in promoting nutritional ketogenesis and significantly improving glycaemia and energy balance end points in both species.
2. Materials and methods
2.1. Development of MSFAT
2.1.1. Separation of fat from milk.
For the laboratory-scale production of MSFAT, raw Jersey milk was used and collected at a local farm (Havdal Farm, Central Jutland, DK) and transported cold to Aarhus University. Upon arrival at our laboratories, the milk was heated to 35 °C in a water bath, and the cream was separated by using a cream separator (Milky FJ 130 ERR, Althofen, Austria). The cream was cooled to approximately 10 °C before being churned into butter using a mixer (Hobart-N50, North York, Ontario, Canada). To isolate the fat from the butter, the butter was melted and heated to 60 °C before centrifugation (4500 rpm, 35 °C, 30 min). The fat was collected and stored at 4 °C overnight. The fat was heated to 40 °C followed by a second centrifugation (4500 rpm, 35 °C, 30 min) for removing residual water and proteins. The fat layer was collected. The process flow is shown in Fig. 1a. The water content of the fat was determined using a moisture analyzer (HR73, Mettler Toledo, Glostrup, Denmark). The moisture content of the used milk fat was approximately 0.16%.
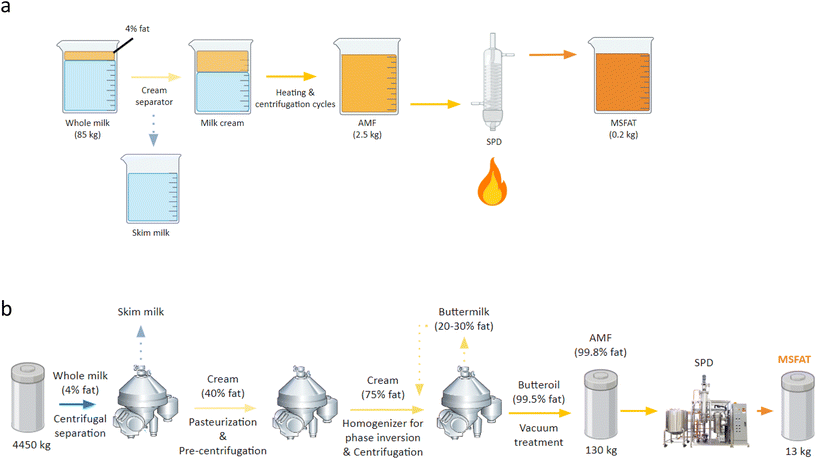 |
| Fig. 1 Schematic representation of the laboratory- (a) and production-scale (b) processes for producing a milk-fat fraction (MSFAT) enriched in medium- and short-chain fatty acids extracted from whole milk sourced from Jersey cows. The laboratory-scale experiment was conducted at a KD-5 SPD facility, while the production-scale experiment utilized the KD-10 SPD facility. | |
For the production-scale process, 130 kg of anhydrous milk fat (AMF) (from ∼4450 kg of milk) was generously donated by the Arla Innovation Centre (Aarhus, Denmark) and transported using a cooling chain to the facilities for production-scale short-path distillation (International Flavors & Fragrances, Inc. (IFF), Aarhus, Denmark). The industrial process flow from milk to distillate is shown in Fig. 1b.
2.1.2. Short-path distillation (SPD).
2.1.2.1. Laboratory-scale.
SPD was carried out in a KD-5 SPD Pilot Plant system (UIC GmbH, Germany) equipped with a vacuum system driven by a rotary vane pump and a diffusion pump. The AMF was heated to 65 °C and kept isothermally before being transferred into the sample addition funnel isothermally controlled by a circulating water bath at 65 °C. Distillation runs were performed at a flow rate of 1 mL min−1 under a pressure of 1.0 × 10−3 mbar, with a blade rotation speed of 300 rpm, and evaporator temperature of 190 °C. The distillate and retentate were collected and stored at 5 °C until analysis.
2.1.2.2. Production-scale.
The AMF was separated using a KD-10 system (UIC GmbH, Germany) at International Flavors & Fragrances, Inc. (IFF) (Aarhus, Denmark). The AMF was heated to 70 °C in a melting pot and kept isothermally for 1 hour. Distillation runs were performed at a flow rate of 5.5–6 kg h−1 under a pressure of 4.0 × 10−3 mbar, with a blade rotation speed of 150 rpm, and evaporator temperature at around 240 °C. The distillate and retentate were collected and stored at 5 °C until analysis.
2.1.3. Fatty acid composition.
Milk FAs were quantified using gas chromatography as described by Larsen et al.,24 except that heptane instead of pentane was used as solvent.
2.1.4. Crystallization and melting behavior analysis by differential scanning calorimetry (DSC).
Crystallization and melting behavior were analyzed using a differential scanning calorimeter (Q2000 DSC, TA Instruments, New Castle, DE, USA) in which nitrogen was used to purge the system. Samples of 15–20 mg of AMF were loaded in aluminum cups. An empty cup was used as a reference. The AMF was heated to 75 °C and kept under isothermal conditions for 15 min before cooling to −40 °C using a cooling rate of 10 °C min−1. Samples were kept at −40 °C for 10 min before heating to 75 °C using a heating rate of 20 °C min−1. DSC analyses were performed in duplicate. Crystallization onset, melting peaks, and offset were identified by using Universal Analysis software (TA Instruments, New Castle, DE, USA).
2.2. Mouse studies
C57BL/6J male mice were obtained from a commercial breeder (Janvier, France). Since female C57BL/6J mice are somewhat protected from diet-induced weight gain and insulin resistance,25 male mice were used. All experiments were performed at 22 °C with a 12
:
12 h light–dark cycle. Mice had ad libitum access to water and a high-fat, high-sucrose diet (HFHS) (D12331, Research Diets, US) for 30 weeks prior to the first experiment, at which point they weighed 62.8 ± 2.4 g. The experiments were approved by the Danish Animal Experimentation Inspectorate (#2020-15-0201-00628) and complied with the European convention for protection of vertebrate animals used for scientific purposes.
Single-housed, four-hours fasted male HFHS-fed DIO C57BL/6J mice (9 months old) were orally gavaged with 10 μL g−1 body weight of laboratory-scale produced MSFAT or corn oil as long-chain triacylglycerol (TG) control (Coop, Denmark). β-Hydroxybutyrate and glucose were measured using handheld meters with test strips (FreeStyle Precision β-ketone, Abbott Laboratories, Chicago, IL, USA and Contour XT, Bayer, Germany) on whole blood samples before and one hour after administration.
In a subsequent study, four-hour fasted single-housed male HFHS-fed DIO C57BL/6J mice (9.5 months of age) were orally gavaged with 10 μL g−1 body weight of laboratory-scale produced MSFAT or corn oil as long-chain triacylglycerol (LCT) control (Coop, Denmark) one hour prior to the dark phase. Throughout the experiment, mice were allowed ad libitum access to a HFHS diet that was weighed before and 6 and 18 hours after oil administration.
2.3. Human study
Nine (age 27 ± 2 years, mean ± SD) healthy, normal to overweight (BMI 26.7 ± 3.7 kg m−2) men were included in the study (Fig. 5a). Inclusion criteria were men, aged 20–45 years, with BMI 22–30 kg m−2 who were moderately physically active (1–4 weekly training sessions, defined as vigorous aerobic exercise or weightlifting). Exclusion criteria were high habitual intake of MCFAs (large amounts of coconut milk, coconut oil, or dairy products), intake of a ketogenic diet, known diabetes or pre-diabetes, use of medications, excessive alcohol consumption, and daily use of nicotine products. All participants provided informed written consent, and the study was approved by the ethics committee of the Capital Region of Denmark (H-21015422).
Participants underwent experimental test days investigating the effects of either MSFAT or LCT intake in a randomized order in a crossover design with at least 14 days wash out between experimental days. Participants were instructed to maintain their normal activity level and diet throughout the study. Two days prior to the experimental days the participants were instructed to consistently eat the same food, abstain from alcohol, and vigorous physical activity to ensure similar conditions.
On the experimental days, the participants arrived by passive transport in an overnight fasted state (12 h) at 8 a.m. On arrival, the participants were weighed, and a catheter was inserted into an antecubital vein. Following 30 min of supine rest, whole-body oxygen uptake was determined by indirect calorimetry (Vyntus CPX Canopy, Vyaire Medical, IL, USA), after which a basal venous blood sample was obtained. Subsequently, participants ingested a test drink containing 68 g of MSFAT (containing ∼21 g of SCFAs + MCFAs and ∼46 g of LCFAs) or LCT (from corn oil containing ∼68 g of LCFAs) dissolved in 90 g of heated low-fat, low-carb cocoa light. This test drink contained 2680 kJ of energy, with 94.5E% fat (68 g), 3.3E% carbohydrate (5 g), and 2.0E% protein (3 g). The test drink was ingested within five minutes and participants were then followed for four hours. To test postprandial glycemic control, participants consumed a mixed meal consisting of pasta with chicken and curry salad dressing together with juice water, with a total energy content of 3160 kJ. The macronutrient composition of this meal was 40.6E% fat (35 g), 42.2E% carbohydrate (78 g), and 15.1E% protein (28 g). To further study the effect of additional MSFAT intake, 90 min later (180 min after the first test drink) another similar test drink containing 68 g of MSFAT or LCT dissolved in cocoa light was ingested. 150 mL of water was provided together with each test drink and the meal.
Venous blood samples were drawn 30, 60, 90, 180, 210 and 240 min after consumption of the first test drink. β-Hydroxybutyrate concentrations in these whole blood samples were measured using a handheld meter with test strips (FreeStyle Precision β-ketone; Abbott Laboratories, Chicago, IL, USA and Contour XT; Bayer, Germany). Plasma glucose concentrations were measured by using an ABL 800 FLEX (Radiometer Medical, CPH, Denmark). At 60 and 240 min after ingestion of the first test drink, indirect calorimetry was applied. Participants rested on a bed throughout the test day.
The concentrations of plasma triacylglycerol (TG) (triacylglycerol GPO-PAP kit, Roche Diagnostics, Mannheim, Germany), high-density lipoprotein (HDL) cholesterol, low-density lipoprotein (LDL) cholesterol, and total cholesterol (HORIBA Medical, Kyoto, Japan) were measured colorimetrically using an autoanalyzer (Pentra C400 analyzer, Horiba, Japan) according to the manufacturers’ instructions.
2.4. Statistics
Data are presented as mean ± standard error of the mean (SEM) except participant characteristica which are presented as mean ± SD. The statistical methods applied are described in each figure legend. The Shapiro–Wilk test and/or box whiskers-plots, residual plots, and normal quantile plots were performed to confirm the assumption of normal distribution and variance homogeneity. Two-way repeated-measure ANOVAs were applied to test for the effect of FA type (MSFAT vs. LCT) and time. When ANOVA revealed an interaction, the Šidák post hoc test was used to test for differences between MSFAT and LCT. Paired Student's t-test was used when comparing MSFAT and LCT as shown in Fig. 5c and f. Differences were considered significant at p < 0.05. Statistical analyses were performed using GraphPad PRISM 8.
3. Results
3.1. Enrichment for SCFAs and MCFAs in bovine milk fat
At the laboratory-scale level, volatility-based separation using SPD led to an ∼2-fold increase (p < 0.0001) in SCFAs (from 7.41% to 15.90%) and MCFAs (9.76% to 17.94%) in the distillate fraction, hereafter referred to as MSFAT (Fig. 2a). Saturated long-chain fatty acid (LCFA) content was reduced (p < 0.0001) from 55.40% to 51.64% despite an enrichment of C13:0–C15:0. In particular, C14:0 was increased to 14.09% in MSFAT (compared to 11.22% in AMF) (Table S1†). For the retentate fraction, no significant changes in FA composition were observed compared to AMF (Table S1†).
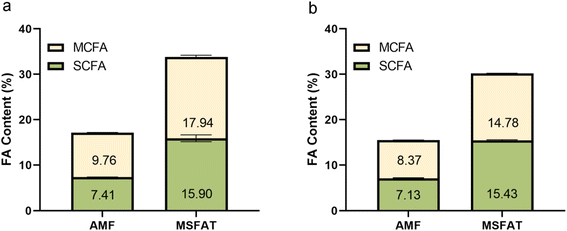 |
| Fig. 2 Proportion of SCFA and MCFA in MSFAT distillates and control AMF from (a) laboratory-scale and (b) production-scale experiments. Fatty acid composition is expressed as percentage by weight of total fatty acids. SCFA includes C4 and C6 FAs, while MCFA includes C8–C12 FAs. | |
Generally, we observed an enrichment of C4:0–C15:0 FAs in MSFAT, whereas the longer chain FA (C16:0–C24:0) content was reduced (Table S1†). Additionally, we found no separation of saturated and unsaturated LCFAs, suggesting that the vapor pressure of triacylglycerol is more heavily influenced by its molecular weight than the presence of double bonds and thus illustrating that the nature of SPD differs from other separation processes.26
At the production-scale level, starting with 130 kg of AMF, we produced 13 kg of food-grade MSFAT with an ∼2-fold increase in SCFAs and MCFAs (Fig. 2b). Overall, the pattern of changes in FA composition was similar to that of the laboratory-scale results, with SCFAs and MCFAs (C4–C15) being enriched, while the content of LCFAs was reduced (Table S1†).
We assessed crystallization and melting of MSFAT and compared its behavior to that of native AMF in order to ascertain whether the reduction in high-melting FAs would be followed by expected lower crystallization and melting offsets. In accordance with this, we observed that both laboratory- and production-scale MSFAT exhibited only one crystallization peak and both crystallization onsets and melting offsets were lower (p < 0.05) compared to those for the native AMF (Fig. 3a and b). Specifically, the crystallization onset of laboratory-scale MSFAT was 9 °C compared to 17 °C for the native milk fat (Fig. 3a); for the production-scale MSFAT, it was reduced from 12 °C to 9 °C (Fig. 3b). The melting offset of native milk fat was 38 °C and 36 °C, and subsequently, SPD MSFAT had a melting offset at 16 °C and 18 °C for laboratory- and production-scale samples, respectively. This confirms the expected differentiated functionality of MSFAT, e.g. meltdown in mouth, compared to native AMF.
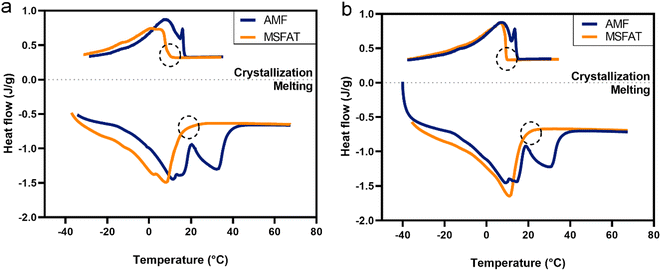 |
| Fig. 3 Differential scanning calorimetry, crystallization and melting curves of (a) laboratory-scale and (b) production-scale experiments. Fat was cooled at 10 °C min−1 and heated at 20 °C min−1. The dotted circles indicate the crystallization onset and melting offset of MSFAT. | |
3.2. Metabolic benefits of milk fat enriched in SCFAs and MCFAs in mice
To investigate the acute metabolic effects of MSFAT intake in a metabolically compromised mouse model, diet-induced obese (DIO) mice were orally gavaged with either 10 μL g−1 MSFAT or calorie-matched LCT oil as a control, which solely contained LCFAs (Fig. 4a). Within one hour, blood ketone body levels were increased 110% (p < 0.0001) after administration of MSFAT, whereas blood ketone body levels only increased 38% (p < 0.05) after administration of isocaloric amounts of LCT oil (Fig. 4b). Within the same period, plasma glucose levels decreased 1.0 mmol L−1 (−12%; p < 0.05) after administration of MSFAT, whereas plasma glucose levels remained unchanged after administration of LCT oil (Fig. 4c). Cumulative food intake of the HFHS diet over an 18-hour period was 42% lower (p < 0.01) after administration of 10 μL g−1 MSFAT compared with administration of isocaloric amounts of LCT oil (Fig. 4d). Together, this shows that oral administration of MSFAT increased circulating ketone bodies, reduced blood glucose levels, and suppressed food intake in obese mice.
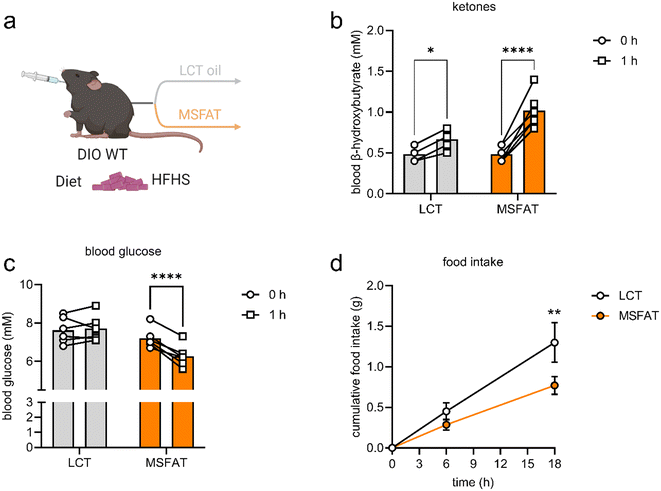 |
| Fig. 4 Metabolic benefits of milk fat enriched in medium- and short-chain fatty acids (MSFAT) in mice. (a) To investigate the effects of MSFAT, 9-month-old C57BL/6J mice with diet-induced obesity (DIO) on a high-fat, high-sucrose (HFHS) diet were orally gavaged with either 10 μL g−1 body weight of MSFAT or corn oil as a control (LCT) that solely contained long-chain fatty acids. (b) Blood β-hydroxybutyrate levels and (c) glucose levels were measured in tail-vein blood samples taken before (0 h) and one hour after gavage with 10 μL g−1 body weight of the indicated lipids. (d) Cumulative food intake over an 18-hour period on the HFHS diet was measured after the administration of 10 μL g−1 body weight of the indicated oils (n = 6–7). Two-way repeated-measure ANOVAs were applied to test for the effect of FA type (MSFAT vs. LCT) and time in (b–d). When ANOVA revealed an interaction, the Šidák post hoc test was used to test for individual effects of time within groups in (b and c) and between groups within time points in (d), respectively. *, ** and **** indicate significant differences (p < 0.05, p < 0.01, p < 0.0001) from 0 hours in (b and c) and from MSFAT within 18 hours in (d). | |
3.3. Metabolic benefits of milk fat enriched in SCFAs and MCFAs in humans
Next, we investigated the acute metabolic effects of MSFAT intake and its role on glycemic control after a mixed meal in healthy young men (Fig. 5a). Intake of MSFAT increased (p < 0.05) circulating ketone body levels 114% and 133% after 60 and 90 min, respectively, while intake of LCT oil had no effect (Fig. 5b). Circulating ketone body levels decreased back to fasting levels in response to a mixed meal but increased (p < 0.05) again by 30% and 55%, respectively, 30 and 60 min after additional ingestion of MSFAT, whereas circulating ketone body levels remained unchanged in the LCT oil group (Fig. 5b). Plasma glucose levels initially decreased similarly in response to ingestion of MSFAT and LCT oil, but, subsequent to a mixed meal, MSFAT ingestion trended to blunt (p = 0.07) the postprandial rise in glucose (Fig. 5c). After ingestion of MSFAT, whole-body oxygen consumption rate (VO2) increased by 54 mL min−1 more during the first hour compared to VO2 after ingestion of LCT oil (Fig. 5e and f). Plasma triacylglycerol (TG) levels increased by a similar extent following the ingestion of MSFAT or LCT (Fig. 5g). Plasma LDL, HDL and total cholesterol did not change during the four hours after MSFAT or LCT intake (Fig. 5h–j).
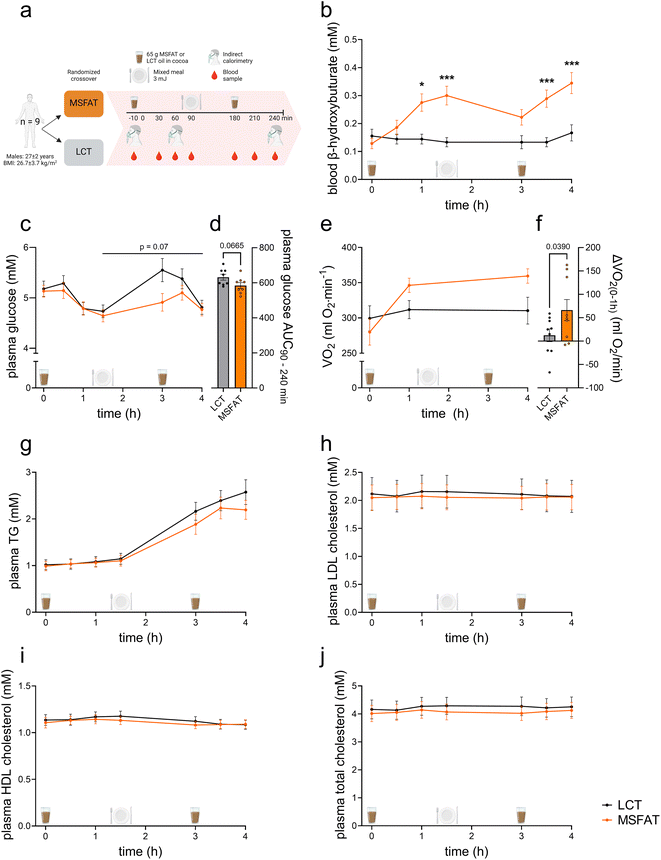 |
| Fig. 5 Intake of milk fat enriched in medium- and short-chain fatty acids (MSFAT) improves ketogenesis, glycaemia, and energy expenditure in healthy men. (a) Acute lipid challenge tests with either milk fat enriched in medium- and short-chain fatty acids (MSFAT) and long-chain triacylglycerol oil (LCT) in male participants (n = 9) in a randomized, crossover design. Participants ingested 68 g of MSFAT or LCT, 90 min later a 3160 kJ mixed meal, and again 90 min later another 68 g of MSFAT or LCT. Blood β-hydroxybutyrate levels (b), plasma glucose levels (c), and postprandial plasma glucose excursion expressed as the 120 min area under the curve (AUC) after the mixed meal (d), oxygen consumption rate (VO2) (e), delta VO2 during the first hour (f), plasma triacylglycerol (TG) (g), plasma LDL- (h), HDL- (i), and total (j) cholesterol determined at the indicated time points. Two-way repeated-measure ANOVAs were applied to test for the effect of FA type (MSFAT vs. LCT) and time in (b, c, e and g–j). When ANOVA revealed an interaction, the Šidák post hoc test was used to test for individual differences between MSFAT and LCT within time points. A paired t-test was applied in (c and f). * and *** indicate significant differences (p < 0.05, p < 0.001) from LCT. | |
Collectively, in humans, ingestion of MSFAT resulted in increased circulating ketone bodies, a trend for attenuated postprandial plasma glucose excursion (p = 0.07), and acutely elevated energy expenditure as compared to isocaloric administration of LCFA-containing fat.
4. Discussion
We herein created and tested a milk product rich in SCFAs and MCFAs. Our efforts culminated in the production of a milk fat, safe for human consumption, containing an ∼100% increase in these special lipids. To explore potential functionality, we conducted testing of our MSFAT product in both mice and humans, uncovering its promising efficacy in promoting nutritional ketogenesis and potentially improving end points related to glycaemia and energy balance in both species as compared to isocaloric administration of long-chain fatty acid rich fat.
Previous attempts to enhance dairy products with SCFAs and MCFAs have produced underwhelming results. In one study, cows were fed a low-fat diet, which led to an increase of about 20% in shorter chain fatty acids.27 This modest increase was insufficient to promote weight loss and improve insulin levels in individuals with obesity.27 Fractionating milk fat has been suggested as an alternative approach, but milk fat is highly complex due to the vast variety of triacylglycerol species it contains. As a result, milk fat is challenging to fractionate compared to simpler fats like palm oil. Conventional fractionation methods, which rely on differences in melting points to obtain soft and hard fat fractions, do not result in higher SCFA and MCFA content in any of the fractions.28,29 Therefore, we aimed to use a different approach based on the volatility of lipid molecules through short-path distillation.30 In the laboratory-scale experiment, we combined this method with the careful selection of raw milk fat from Jersey cows, which contains the most SCFAs and MCFAs compared to milk fat from Danish Holsteins and Swedish Reds.21 Despite different operational conditions between the laboratory- and production-scale processes, we successfully produced two novel milk fat fractions with two times more SCFAs and MCFAs. This comparable increase is attributed to the parallel increase in temperature and flow rate, resulting in similar evaporation patterns for the fatty acids at the laboratory scale and production scale. The two distillate fractions had similar thermal behaviors with lower melting peak and offset melting temperature, resulting in a fraction that was liquid at ambient temperature. Milk fat typically shows three endothermic peaks corresponding to the low melting fraction (below 10 °C), the medium melting fraction (from 10–19 °C), and the high melting fraction (above 20 °C).31 However, the melting profile of the distillate only showed one endothermic peak likely due to the higher content of SCFAs and MCFAs.
Our approach does not involve the use of harmful organic solvents, which are detrimental to the environment. Additionally, the remaining retentate in our process closely resembles regular milk fat, allowing for normal downstream processing and minimization of waste. To potentially further increase SCFA and MCFA content, one could consider using milk from cows fed a low-fat/high-carbohydrate diet. Moreover, milk from other ruminants, such as goats, naturally contains higher levels of MCFA and SCFA,32 making it an interesting raw material to further enrich for these beneficial lipids.
The present findings that MSFAT increases nutritional ketogenesis and improves end points related to glycaemia and energy balance in both mice and human individuals agree with previously reported effects of supplementation of commercial triacylglycerol oils containing MCFA, which also increase circulating ketone body levels,14,33–39 boost postprandial thermogenesis,33,40,41 and reduce plasma glucose.33,39,42 The induction of ketogenesis is particularly interesting, due to the recent accumulating body of evidence demonstrating beneficial effects of increasing circulating ketone body levels in cardiovascular diseases,43–46 cognitive disorders,47,48 and Alzheimer's disease.49 Easy-to-implement nutritional tools to induce ketogenesis are needed as an alternative to intravenous infusion, oral intake of ketone esters and ketogenic diets, which all have serious limitations.50 Importantly, previous studies using pure MCFA-based triacylglycerol oil (MCT oil) administer only one MCFA (almost exclusively C8:0-rich MCT), and although C8:0 has been suggested to be the most ketogenic in both mice9 and humans,14,38 C10:0 has been shown to be the most potent to lower blood glucose levels in mice,9 while C10:0 and C12:0 are the most potent MCFAs to activate the G-coupled protein receptor 84 (GPR84) and improve nonalcoholic steatohepatitis in mice.51 The mixture of various MCFAs in the MSFAT is hence likely appealing in potently inducing various beneficial physiological effects. Moreover, in those previous studies using commercial pure C8:0 MCT oil, participants ingested 20–64 g of MCT oil.14,33–42 Taking this into consideration, it could imply an astonishing potency of the MSFAT compared to pure MCT oils to induce these physiological effects, since we used a bolus containing 10 g of MCFAs in the 67 g of bolus for human consumption. Likewise, we observed lowered food intake in mice in response to oral administration of just ∼0.6 g of MSFAT containing ∼0.1 g of MCFAs, which is less than half of the amounts used in previous studies looking into MCT satiety effects of C8:0 MCT oils in mice.9,52 Furthermore, it has been shown that emulsification of commercial pure MCT oil into a lactose-free skim-milk matrix increased the ketogenic effect and diminished the gastrointestinal discomfort in humans that it is often observed when ingesting commercial pure MCT oil.34 Interestingly, previous investigations looking at health benefits of dietary SCFA and MCFA supplementation have largely focused on one or the other while the combined effects of supplementing both have rarely been addressed. Collectively, therefore, our observations are indicative of some sort of food/dairy matrix effect providing improved functionality and health effects compared with those exhibited by the MCFAs in isolation,53,54 which needs to be investigated and directly compared further in future studies.
In this initial proof-of-concept study, we investigated the effects of MSFAT in healthy, younger adult male subjects, to explore acute end points related to energy balance and glycemia. Whether these findings extend to women and/or persons who are overweight or obese will need to be investigated. It is also unclear whether these effects persist during several days of ingesting MSFAT.
In conclusion, we herein developed a novel, large-scale volatility-based workflow to enrich milk fat with shorter-chain fatty acids and through subsequent proof-of-concept studies in mice and humans indicated that fortified MSFAT milk products may hold significant metabolic advantages. Thus, volatility-based enrichment for SCFAs and MCFAs is a powerful tool in producing milk fat fractions with improved functional and health properties while simultaneously keeping a fraction (retentate fraction) with similar properties as native milk fat, thereby minimizing waste. Follow-up studies are warranted to understand chronic effects and also the interplay with factors such as age and sex.
Conflicts of interest
There are no conflicts to declare.
Acknowledgements
We thank Gitte Hald Kristiansen, Rita Albrechtsen, and Irene Bech Nielsen for skilled technical assistance. We would like to gratefully recognize the volunteers for participating in the study. This study was supported by the Novo Nordisk Foundation (grant no. NNF20OC0063744), Arla Food for Health, and the Danish Dairy Research Foundation. The PhD scholarship of J. M. K. was supported by a research grant from the Danish Cardiovascular Academy, which is funded by the Novo Nordisk Foundation (grant no. NNF20SA0067242) and the Danish Heart Foundation. A.-M. L. and A. M. F. were funded by the Danish Diabetes Academy, funded by the Novo Nordisk Foundation (grant no. NNF17SA0031406). A. M. F. was also funded directly by the Novo Nordisk Foundation (grant no. NNF22OC0074110). M. K. was supported by the Deutsche Forschungsgemeinschaft (DFG; KL 3285/5-1), the German Center for Diabetes Research (grant no. 82DZD03D03 and 82DZD03D1Y), the Novo Nordisk Foundation (grant no. NNF19OC0055192) and the Deutsche Diabetes Gesellschaft (DDG).
References
- I. Siró, E. Kápolna, B. Kápolna and A. Lugasi, Functional food. Product development, marketing and consumer acceptance – A review, Appetite, 2008, 51(3), 456–467 CrossRef PubMed.
- C. S. Byrne, E. S. Chambers, D. J. Morrison and G. Frost, The role of short chain fatty acids in appetite regulation and energy homeostasis, Int. J. Obes., 2015, 39(9), 1331–1338 CrossRef CAS PubMed.
- S. Coppola, R. Nocerino, L. Paparo, G. Bedogni, A. Calignano and C. Di Scala,
et al., Therapeutic Effects of Butyrate on Pediatric Obesity: A Randomized Clinical Trial, JAMA Network Open, 2022, 5(12), e2244912 CrossRef PubMed.
- M. P. St-Onge and P. J. Jones, Physiological effects of medium-chain triglycerides: potential agents in the prevention of obesity, J. Nutr., 2002, 132(3), 329–332 CrossRef CAS PubMed.
- T. Maher and M. E. Clegg, A systematic review and meta-analysis of medium-chain triglycerides effects on acute satiety and food intake, Crit. Rev. Food Sci. Nutr., 2021, 61(4), 636–648 CrossRef CAS PubMed.
- A. M. Lundsgaard, A. M. Fritzen, K. A. Sjøberg, M. Kleinert, E. A. Richter and B. Kiens, Small Amounts of Dietary Medium-Chain Fatty Acids Protect Against Insulin Resistance During Caloric Excess in Humans, Diabetes, 2021, 70(1), 91–98 CrossRef CAS PubMed.
- R. H. Eckel, A. S. Hanson, A. Y. Chen, J. N. Berman, T. J. Yost and E. P. Brass, Dietary substitution of medium-chain triglycerides improves insulin-mediated glucose metabolism in NIDDM subjects, Diabetes, 1992, 41(5), 641–647 CrossRef CAS PubMed.
- E. E. Canfora, J. W. Jocken and E. E. Blaak, Short-chain fatty acids in control of body weight and insulin sensitivity, Nat. Rev. Endocrinol., 2015, 11(10), 577–591 CrossRef CAS PubMed.
- J. M. Kanta, L. Deisen, K. Johann, S. Holm, A. Lundsgaard and J. Lund,
et al., Dietary medium-chain fatty acids reduce food intake via the GDF15-GFRAL axis in mice, Mol. Metab., 2023, 74, 101760 CrossRef CAS PubMed.
- S. Wein, S. Wolffram, J. Schrezenmeir, D. Gasperiková, I. Klimes and E. Seböková, Medium-chain fatty acids ameliorate insulin resistance caused by high-fat diets in rats, Diabetes/Metab. Res. Rev., 2009, 25(2), 185–194 CrossRef CAS PubMed.
- R. Tang and L. Li, Modulation of Short-Chain Fatty Acids as Potential Therapy Method for Type 2 Diabetes Mellitus, Can. J. Infect. Dis. Med. Microbiol., 2021, 2021, 6632266 Search PubMed.
- J. Iqbal and M. M. Hussain, Intestinal lipid absorption, Am. J. Physiol. Endocrinol. Metab., 2009, 296(6), E1183–E1194 CrossRef CAS PubMed.
- P. Schönfeld and L. Wojtczak, Short- and medium-chain fatty acids in energy metabolism: the cellular perspective, J. Lipid Res., 2016, 57(6), 943–954 CrossRef PubMed.
- V. St-Pierre, C. Vandenberghe, C. M. Lowry, M. Fortier, C. A. Castellano and R. Wagner,
et al., Plasma Ketone and Medium Chain Fatty Acid Response in Humans Consuming Different Medium Chain Triglycerides During a Metabolic Study Day, Front. Nutr., 2019, 6, 46 CrossRef PubMed.
- Y. Xu, X. Ye, Y. Zhou, X. Cao, S. Peng and Y. Peng,
et al., Sodium butyrate activates HMGCS2 to promote ketone body production through SIRT5-mediated desuccinylation, Front. Med., 2023, 17(2), 339–351 CrossRef PubMed.
- P. Puchalska and P. A. Crawford, Multi-dimensional Roles of Ketone Bodies in Fuel Metabolism, Signaling, and Therapeutics, Cell Metab., 2017, 25(2), 262–284 CrossRef CAS PubMed.
- G. den Besten, K. van Eunen, A. K. Groen, K. Venema, D. J. Reijngoud and B. M. Bakker, The role of short-chain fatty acids in the interplay between diet, gut microbiota, and host energy metabolism, J. Lipid Res., 2013, 54(9), 2325–2340 CrossRef CAS.
- B. Marten, M. Pfeuffer and J. Schrezenmeir, Medium-chain
triglycerides, Int. Dairy J., 2006, 16(11), 1374–1382 CrossRef CAS.
- L. Cordain, S. B. Eaton, A. Sebastian, N. Mann, S. Lindeberg and B. A. Watkins,
et al., Origins and evolution of the Western diet: health implications for the 21st century, Am. J. Clin. Nutr., 2005, 81(2), 341–354 CrossRef CAS PubMed.
- R. G. Jensen, The composition of bovine milk lipids: January 1995 to December 2000, J. Dairy Sci., 2002, 85(2), 295–350 CrossRef CAS PubMed.
- N. A. Poulsen, F. Gustavsson, M. Glantz, M. Paulsson, L. B. Larsen and M. K. Larsen, The influence of feed and herd on fatty acid composition in 3 dairy breeds (Danish Holstein, Danish Jersey, and Swedish Red), J. Dairy Sci., 2012, 95(11), 6362–6371 CrossRef CAS PubMed.
- M. K. Larsen, K. K. Andersen, N. Kaufmann and L. Wiking, Seasonal variation in the composition and melting behavior of milk fat, J. Dairy Sci., 2014, 97(8), 4703–4712 CrossRef CAS PubMed.
- N. R. Róin, G. M. S. Lokuge, M. B. Fredsted, U. K. Sundekilde, M. K. Larsen and L. B. Larsen,
et al., Variations in fatty acids, micronutrients and metabolites in discrete milk lines of Danish dairy milk, Int. Dairy J., 2023, 147, 105786 CrossRef.
- M. K. Larsen, U. Kidmose, T. Kristensen, P. Beaumont and G. Mortensen, Chemical composition and sensory quality of bovine milk as affected by type of forage and proportion of concentrate in the feed ration, J. Sci. Food Agric., 2013, 93(1), 93–99 CrossRef CAS PubMed.
- M. Kleinert, C. Clemmensen, S. M. Hofmann, M. C. Moore, S. Renner and S. C. Woods,
et al., Animal models of obesity and diabetes mellitus, Nat. Rev. Endocrinol., 2018, 14(3), 140–162 CrossRef PubMed.
- J. Arul, A. Boudreau, J. Makhlouf, R. Tardi and T. Bellavia, Fractionation of anhydrous milk fat by short-path distillation, J. Am. Oil Chem. Soc., 1988, 65(10), 1642 CrossRef CAS.
- M. Bohl, A. Bjørnshave, K. V. Rasmussen, A. G. Schioldan, B. Amer and M. K. Larsen,
et al., Dairy proteins, dairy lipids, and postprandial lipemia in persons with abdominal obesity (DairyHealth): a 12-wk, randomized, parallel-controlled, double-blinded, diet intervention study, Am. J. Clin. Nutr., 2015, 101(4), 870–878 CrossRef CAS PubMed.
- M. C. Michalski, N. Leconte, V. Briard-Bion, J. Fauquant, J. L. Maubois and H. Goudédranche, Microfiltration of raw whole milk to select fractions with different fat globule size distributions: process optimization and analysis, J. Dairy Sci., 2006, 89(10), 3778–3790 CrossRef CAS.
- G. A. van Aken, E. ten Grotenhuis, A. J. van Langevelde and H. Schenk, Composition and crystallization of milk fat fractions, J. Am. Oil Chem. Soc., 1999, 76(11), 1323–1331 CrossRef CAS.
- R. J. Campos, J. W. Litwinenko and A. G. Marangoni, Fractionation of milk fat by short-path distillation, J. Dairy Sci., 2003, 86(3), 735–745 CrossRef CAS PubMed.
- E. Frede, K. E. Kaylegian and R. C. Lindsay: Handbook of Milkfat Fractionation Technology and Application. XXIV and 662 pages, numerous figures and tables. AOCS Press, Champaign, Illinois, 1995. Price: 150.00 US $, Nahrung, 1996, 40(3), 158 CrossRef.
- E. Karrar, I. A. Mohamed Ahmed, T. Huppertz, W. Wei, J. Jin and X. Wang, Fatty acid composition and stereospecificity and sterol composition of milk fat from different species, Int. Dairy J., 2022, 128, 105313 CrossRef CAS.
- T. B. Seaton, S. L. Welle, M. K. Warenko and R. G. Campbell, Thermic effect of medium-chain and long-chain triglycerides in man, Am. J. Clin. Nutr., 1986, 44(5), 630–634 CrossRef CAS PubMed.
- A. Courchesne-Loyer, M. Fortier, J. Tremblay-Mercier, R. Chouinard-Watkins, M. Roy and S. Nugent,
et al., Stimulation of mild, sustained ketonemia by medium-chain triacylglycerols in healthy humans: estimated potential contribution to brain energy metabolism, Nutrition, 2013, 29(4), 635–640 CrossRef CAS PubMed.
- J. Norgren, S. Sindi, A. Sandebring-Matton, I. Kåreholt, M. Daniilidou and U. Akenine,
et al., Ketosis After Intake of Coconut Oil and Caprylic Acid-With and Without Glucose: A Cross-Over Study in Healthy Older Adults, Front. Nutr., 2020, 7, 40 CrossRef.
- C. Heidt, M. Fobker, M. Newport, R. Feldmann, T. Fischer and T. Marquardt, Beta-Hydroxybutyrate (BHB), Glucose, Insulin, Octanoate (C8), and Decanoate (C10) Responses to a Medium-Chain Triglyceride (MCT) Oil with and without Glucose: A Single-Center Study in Healthy Adults, Nutrients, 2023, 15(5), 1148 CrossRef CAS PubMed.
- F. X. Pi-Sunyer, S. A. Hashim and T. B. Van Itallie, Insulin and ketone responses to ingestion of medium and long-chain triglycerides in man, Diabetes, 1969, 18(2), 96–100 CrossRef CAS PubMed.
- C. Vandenberghe, V. St-Pierre, T. Pierotti, M. Fortier, C. A. Castellano and S. C. Cunnane, Tricaprylin Alone Increases Plasma Ketone Response More Than Coconut Oil or Other Medium-Chain Triglycerides: An Acute Crossover Study in Healthy Adults, Curr. Dev. Nutr., 2017, 1(4), e000257 CrossRef PubMed.
- P. Tantibhedhyangkul, S. A. Hashim and T. B. Van Itallie, Effects of ingestion of long-chain triglycerides on glucose tolerance in man, Diabetes, 1967, 16(11), 796–799 CrossRef CAS PubMed.
- M. E. Clegg, M. Golsorkhi and C. J. Henry, Combined medium-chain triglyceride and chilli feeding increases diet-induced thermogenesis in normal-weight humans, Eur. J. Nutr., 2013, 52(6), 1579–1585 CrossRef CAS PubMed.
- L. Scalfi, A. Coltorti and F. Contaldo, Postprandial thermogenesis in lean and obese subjects after meals supplemented with medium-chain and long-chain triglycerides, Am. J. Clin. Nutr., 1991, 53(5), 1130–1133 CrossRef CAS PubMed.
- M. P. St-Onge, B. Mayrsohn, M. O'Keeffe, H. R. Kissileff, A. R. Choudhury and B. Laferrère, Impact of medium and long chain triglycerides consumption on appetite and food intake in overweight men, Eur. J. Clin. Nutr., 2014, 68(10), 1134–1140 CrossRef CAS PubMed.
- R. Nielsen, N. Møller, L. C. Gormsen, L. P. Tolbod, N. H. Hansson and J. Sorensen,
et al., Cardiovascular Effects of Treatment With the Ketone Body 3-Hydroxybutyrate in Chronic Heart Failure Patients, Circulation, 2019, 139(18), 2129–2141 CrossRef CAS PubMed.
- R. Nielsen, K. H. Christensen, N. Gopalasingam, K. Berg-Hansen, J. Seefeldt and C. Homilius,
et al., Hemodynamic Effects of Ketone Bodies in Patients With Pulmonary Hypertension, J. Am. Heart Assoc., 2023, 12(10), e028232 CrossRef CAS PubMed.
- G. D. Lopaschuk and J. R. B. Dyck, Ketones and the cardiovascular system, Nat. Cardiovasc. Res., 2023, 2(5), 425–437 CrossRef.
- S. Takahara, S. Soni, K. Phaterpekar, T. T. Kim, Z. H. Maayah and J. L. Levasseur,
et al., Chronic exogenous ketone supplementation blunts the decline of cardiac function in the failing heart, ESC Heart Fail., 2021, 8(6), 5606–5612 CrossRef PubMed.
- M. Altayyar, J. A. Nasser, D. Thomopoulos and M. Bruneau Jr., The Implication of Physiological Ketosis on the Cognitive Brain: A Narrative Review, Nutrients, 2022, 14(3), 513 CrossRef PubMed.
- M. Fortier, C. A. Castellano, E. Croteau, F. Langlois, C. Bocti and V. St-Pierre,
et al., A ketogenic drink improves brain energy and some measures of cognition in mild cognitive impairment, Alzheimer's Dementia, 2019, 15(5), 625–634 CrossRef PubMed.
- P. Chatterjee, M. Fernando, B. Fernando, C. B. Dias, T. Shah and R. Silva,
et al., Potential of coconut oil and medium chain triglycerides in the prevention and treatment of Alzheimer's disease, Mech. Ageing Dev., 2020, 186, 111209 CrossRef CAS PubMed.
- S. Takahara, S. Soni, Z. H. Maayah, M. Ferdaoussi and J. R. B. Dyck, Ketone therapy for heart
failure: current evidence for clinical use, Cardiovasc. Res., 2021, 118(4), 977–987 CrossRef PubMed.
- R. Ohue-Kitano, H. Nonaka, A. Nishida, Y. Masujima, D. Takahashi and T. Ikeda,
et al., Medium-chain fatty acids suppress lipotoxicity-induced hepatic fibrosis via the immunomodulating receptor GPR84, JCI Insight, 2023, 8(2), e165469 CrossRef PubMed.
- V. R. Haynes, N. J. Michael, M. van den Top, F. Y. Zhao, R. D. Brown and D. De Souza,
et al., A Neural basis for Octanoic acid regulation of energy balance, Mol. Metab., 2020, 34, 54–71 CrossRef CAS PubMed.
- J. M. Aguilera, The food matrix: implications in processing, nutrition and health, Crit. Rev. Food Sci. Nutr., 2019, 59(22), 3612–3629 CrossRef CAS PubMed.
- C. M. Weaver, Dairy matrix: is the whole greater than the sum of the parts?, Nutr. Rev., 2021, 79(Suppl. 2), 4–15 CrossRef PubMed.
|
This journal is © The Royal Society of Chemistry 2024 |
Click here to see how this site uses Cookies. View our privacy policy here.