DOI:
10.1039/D3FO05297D
(Paper)
Food Funct., 2024,
15, 2906-2919
Improvement of fruit juice quality: novel endo-polygalacturonase II from Aspergillus tubingensis FAT 43 for enhanced liquefaction, clarification, and antioxidant potential
Received
30th November 2023
, Accepted 18th February 2024
First published on 19th February 2024
Abstract
This study focuses on the isolation, purification, and characterisation of endo-polygalacturonase II from Aspergillus tubingensis FAT43, particularly emphasising its potential applications in the fruit juice industry. A comprehensive screening test revealed the temporal dynamics of endo-polygalacturonase production during a 96-hour fermentation process. The purification process, involving ammonium sulfate and ethanol precipitation followed by ion-exchange chromatography, resulted in a 3.3-fold purification of PG II with a yield of 16% and a specific activity of 6001.67 U mg−1. Molecular analysis confirmed the identity of PG II, its gene (pgaII), and a high degree of sequence identity with Aspergillus tubingensis in the SWISS-PROT database. The optimal pH for PG II activity was 3.5–4.5, with robust stability across a broad pH spectrum (3–7). The enzyme exhibited optimal temperature activity at 45 °C, with a retention of 90% activity at 50 °C. The calculated activation energy for PG II was 62.1 kJ mol−1, indicating good stability. Inactivation kinetics revealed a half-life of 13.7 h at 40 °C, 5.4 h at 50 °C, and 0.85 h at 60 °C, with an activation energy of denaturation of 32.8 kJ mol−1. Compared to literature-reported PGs, PG II from A. tubingensis FAT43 demonstrated superior thermal stability. Hydrolysis experiments on different pectins revealed the highest specificity for non-methylated substrates (polygalacturonic acid). In fruit juice processing, PG II significantly increased juice yield and clarity, with the highest impact observed in strawberry juice. Antioxidant activity assays indicated enhanced antioxidant potential in enzyme-treated juices, especially strawberry, quince, and apple juices. The study highlights PG II's potential as an industrially valuable enzyme for fruit juice processing, offering improved thermostability and versatility across various fruit types.
1. Introduction
Pectic polysaccharides play a pivotal role in inducing cloudiness in fruit juices, posing a significant challenge within the fruit juice industry.1 These intricate carbohydrates are predominantly located in the middle lamella and cell walls of plant tissues.2,3 In a sample of fresh fruit material, pectin comprises between 0.5 and 4% of the total mass.4 The elaborate and diverse structures of pectin, characterised by various linkages and modifications, significantly contribute to fruit juices’ viscosity and cloudiness. This cloudiness emerges due to the interaction of pectic polysaccharides with other components present in the juice.5 If these polysaccharides are insufficiently broken-down during juice processing, they have the potential to form gels and fibrillar structures, thereby increasing viscosity.6 This phenomenon affects the visual clarity of the juice and impedes the efficient separation of liquid and solid phases during processing.7
Pectinolytic enzymes, commonly known as pectinases, constitute a vital group of enzymes that hydrolyse pectin by breaking the α-1,4 glycosidic bonds between D-galacturonic acid molecules. Three criteria are used in the literature to classify pectinases: substrate structure (pectin, pectic acid, or oligo-D-galacturonate), catalytic reaction site (random, endo-activity, or catalysis from the ends of polymer chains, exo-activity), and the type of reaction (hydrolase, lyase, or esterase). These enzymes can be isolated from various sources, including plants, bacteria, fungi, and yeasts.4,8
Filamentous fungi, particularly species of the Aspergillus genus, can produce pectinolytic enzymes at elevated concentrations.4 Certain Aspergillus species, during infection or culturing, produce enzymes such as polygalacturonase (PGs), pectin methylesterase (PMEs), and pectate lyase (PLs). Alkaline pectinases exhibit activity in the retting and degumming of plant material, proving valuable in fruit waste processing. Simultaneously, acidic pectinase finds widespread use in eliminating and clarifying fruit juice.9 The diverse applications of these enzymes highlight their significance in industrial and agricultural processes.10 Commercial pectinase is typically a mixture of enzymes. This is an advantage given the complex nature of plant tissues and the various chemical bonds that need to be hydrolysed. However, a more targeted approach may be essential in certain industrial scenarios, requiring a specific type of pectinase or a carefully selected enzyme mix.
To overcome the challenge of cloudiness, the fruit juice industry employs enzymes known as endo-pectinases, with endo-polygalacturonases (endo-PGs) being a prominent example. The filamentous fungus Aspergillus tubingensis FAT 43 was selected from optimised solid-state fermentation as a good endo-PG producer. Endo-PG targets the internal regions of pectin molecules, breaking them down into smaller, soluble fragments. This enzymatic action effectively reduces the viscosity of the juice by disrupting the complex pectin network, resulting in clearer and more transparent juices.
This study analysed the benefits of endopolygalacturonase II (PG II) from A. tubingensis FAT 43. Firstly, PG II enhances the clarity of fruit juices, meeting consumer preferences for visually appealing and transparent beverages. Secondly, these enzymes improve juice yield during pressing by breaking down pectic polysaccharides. This increased yield is valuable in optimising resource utilisation and reducing waste in fruit processing. Moreover, applying PG II positively influences the antioxidative attributes of the juices.11 Cloudiness removal improves product quality and marketability, meeting industry standards and consumer expectations.
2. Materials and methods
2.1 Enzyme production by solid state fermentation
The production of endo-PG involved the utilisation of the A. tubingensis strain FAT43, following the protocol outlined by Pavlović et al. (2023).12 Initially, the strain was cultivated on Potato Dextrose Agar (PDA) medium, maintaining controlled conditions (temperature 28 °C with a relative humidity of 60%) for five days until sporulation occurred.13 Subsequently, spores were harvested using a 0.1% Tween 20 solution, and their concentration was quantified at 2 × 106 spores per mL using a Shimadzu UV-Vis spectrophotometer, measuring optical density (OD) at a wavelength of 605 nm.14 Under optimised conditions (temperature 28 °C with a relative humidity of 60%), sugar beet pulp and wheat bran substrates were used for solid-state fermentation for 96 h. The fermentation was finished by extracting enzymes from the fermentation media using 25 mM acetate buffer at pH 4.5.
2.2 Diffusion assay for endo-PG activity
The endo-PG activity was detected by performing a diffusion test on a polyacrylamide gel with 0.2% low methylated pectin solution (in 50 mM acetate buffer pH 4.5). The gel was stained with 0.05% (w/w) Ruthenium Red dye, which enabled the detection of clear zones where the pectin is degraded due to enzymatic activity. The gel was prepared by punching wells after it was polymerised, and then 10 μL enzyme samples were added into the wells. Diffusion was set up in a humid environment at 45 °C for 1 h. After the incubation, the gel was washed with distilled water and stained with Ruthenium Red solution for 20 min. Clear zones around the wells indicated the presence of endo-PG activity.
2.3 Purification of endo-PG
The primary enzyme extract underwent a series of purification steps to obtain a partially purified enzyme. Initially, the concentration of enzymes was achieved by ultrafiltration, using 10 kDa molecular mass cut-off membranes. The resulting concentrated extract was then subjected to a cold (−20 °C) ethanol precipitation, followed by centrifugation at 13
000g for 10 min. The resulting precipitate was dissolved in 5 mL of 25 mM acetate buffer pH 6, and a subsequent centrifugation step was applied to eliminate insoluble particles, yielding a clarified enzyme solution.
Further purification involved ammonium sulfate (AS) precipitation at various concentrations (30%, 50%, and 70% saturation). The gradual addition of solid AS and continuous mixing with a magnetic stirrer resulted in a protein pellet after precipitation with 70% AS saturation. The pellet was dissolved in 5 mL of 25 mM acetate buffer pH 4.5. The clear, dissolved protein pellet was then subjected to size-exclusion chromatography on a Sephacryl S100HR (column, 530 × 30 mm; bed size, 450 mm; flow rate, 0.8 mL min−1; sample volume 1,5% of the column volume), pre-equilibrated with 25 mM acetate buffer, pH 4.5. The collected fractions were then analysed using the polygalacturonase activity assay and SDS PAGE to determine the size distribution of the proteins sizes in the sample.
Following size-exclusion chromatography, eluted active fractions underwent ion-exchange chromatography on a DEAE Sephadex column, 25 mM acetate pH 4.5. A gradient elution technique was applied after loading the column with samples showing endo-PG activity. A linear gradient ranging from 0 to 0.5 M NaCl was applied for enzyme elution. The collected fractions were analysed for endo-PG using enzyme activity assay and SDS PAGE.
The partially purified enzyme was subjected to a final polishing step on a Q Sepharose column to achieve final purification. The protein sample underwent buffer exchange, transitioning from acetate to 25 mM Tris-HCl pH 7. Q Sepharose column was pre-equilibrated in the same buffer. A linear gradient ranging from 0 to 0.5 M NaCl was applied for the enzyme elution. All collected fractions were subjected to the endo-PG activity assay, and SDS-PAGE was used to determine enzyme purity. The PG II purification scheme is shown in Fig. 1.
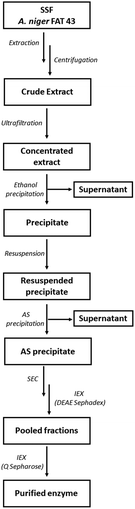 |
| Fig. 1 Flowchart depicting the extraction and purification procedure of endo-PG. | |
After completing the purification steps, the protein concentration of each fraction was determined using the Bradford method, with bovine serum albumin as a standard.15
2.4 Polygalaturonase activity assay
Polygalacturonase activity was determined using the DNS method described by Bernfeld in 1955.16 The reaction mixture was composed of 50 μL of sample and 450 μL of 0.5% (w/w) polygalacturonate in an acetate buffer with a pH of 4.5. The mixture was incubated for 15 min at 45 °C, and then the reaction was stopped by adding the DNS reagent and boiling for 5 min at 100 °C. The absorbance at 540 nm was measured using a spectrophotometer, and the enzyme activity was calculated using an enzyme activity standard curve for D-galacturonate. One unit of activity (1 U) was defined as the amount of enzyme capable of releasing 1 μmol of D-galacturonic acid per min.
2.5 Electrophoresis analysis
To assess the purity and composition of the active chromatographic fraction, SDS-PAGE was performed on a 10% (m/v) gel.17 The apparent molecular mass was determined using a standard protein mixture Blue Wide Range Protein Ladder (10–245 kDa, Cleaver Scientific).
2.6 Proteomic analysis: mass spectrometry characterisation
A 10 μL protein sample was reduced and alkylated in one step with 10 mM TCEP and 40 mM CAA in the dark at room temperature for 30 min. The sample was then diluted to 100 μL with 25 mM NH4HCO3, and 60 ng of trypsin was added for overnight hydrolysis at 37 °C. The reaction was terminated by the addition of 1 μL trifluoroacetic acid. The resulting peptides were analysed using a Bruker Daltonics Compact ESI-qTOF mass spectrometer, coupled to a Waters Acquity UPLC. A 2 μL hydrolysate was loaded onto a Waters ACQUITY UPLC M-Class Symmetry C18 trap column and washed with 0.1% (v/v) formic acid in water for 10 min. Peptides were separated on a Bruker DaltonicsPepSep C18 analytical column with a 30-minute gradient from 5% to 45% solvent B (0.1% formic acid in ACN), followed by a 1-minute gradient to 90% solvent B at 0.4 μL min−1. The electrospray ion source operated in positive mode and scanned the precursor MS in the m/z range 150–2200. Spectral data were acquired using the Compass HyStar 6.0 software package and exported as Mascot generic files. They were searched against the SwissProt database using MASCOT software (version 2.8) with the following parameters: 20 ppm peptide and 0.6 Da fragment mass error tolerance, 3 enzyme missed cleavages, 2+ and 3+ peptide charges, carbamidomethyl Cys as a fixed and oxidised Met as a variable modification, and an automatic Decoy database search.
2.7 Gene amplification
To confirm the presence of the A. tubingensis pgaII gene for polygalacturonase (PG II) two sets of primers were designed: one for first exon (PecAt_ex1_Fw1 ATCCCCCATCGAAGCCCGG and one PecAt_ex1_Rev1 CGCCAGAGTTGATCGCAAGACAG resulting in 570 bp PCR DNA fragment) and second pair of primers for exon II (PecAt_ex2_Fw2 CATCTGGTTTACCAGCGGC and PecAt_ex2_Rev2 CAAAAGCTTGTTAGCAAGTGCTACAACCG resulting in 502 bp PCR fragment) based on GenBank sequence X58894.
The total DNA of A. tubingensis strain FAT43 was extracted from the white mycelium of isolate grown on PDA (3 days at 28 °C) using the protocol Day and Shattock (1997) described.18 Polymerase chain reaction (PCR) was performed using Phusion High Fidelity DNA Polymerase (0.5 U per 50 μL reaction) with isolated total DNA of strain FAT43 and designed primers under the following conditions: initial denaturation 2 min at 98 °C, 30 cycles composed of the following steps: denaturation for 20 seconds at 98 °C, annealing for 30 s at 55 °C, polymerisation at 72 °C and final polymerisation for 5 min at 72 °C. The amplification success of according DNA fragments was analysed on a 1% agarose gel. The size of the fragments was determined based on the standard GeneRuler DNA Ladder Mix (Thermo Scientific).
2.8 PG characterisation
Purified PG II was biochemically characterised to optimise its efficiency and investigate its possible application in biotechnology.
2.8.1 Isoelectric focusing electrophoresis and zymography.
The pI value of PG II was determined using isoelectric focusing electrophoresis (IEF). The method was performed on a 6% polyacrylamide gel (PA) in accordance with the manufacturer's instructions (LKB Pharmacia, Sweden), at 1000 V, 4.8 Ma, and 5 W for 1.5 h. Calibration was done using ampholytes (3.5–9.5 pH). After IEF, the PA gel was washed with distilled water and 50 mM acetate buffer pH 4, and then incubated in 0.5% LM pectin solution (in 50 mM acetate buffer pH 4) for 1 h. The visualisation of PGII was achieved by using a 0.05% RR solution for 20 min.
2.8.2 Determination of temperature optimum, thermal stability and thermal inactivation kinetics.
The optimal temperature range (30 to 60 °C) for PG II was determined by assessing activity in 50 mM acetate buffer, pH 4. The activation energy was calculated using the Arrhenius equation after measuring enzyme activities at different temperatures by plotting ln
k versus 1/T, where k is a rate constant at temperature T (absolute temperature) according to the linear modification of Arrhenius equation:
Thermal stability and the kinetics of irreversible loss of enzyme activity were investigated: the purified enzyme was distributed into test tubes and subjected to incubation at temperatures of 40, 50, and 60 °C for varying time intervals (t). At specific time points, samples were collected, and the remaining activity was measured using the enzyme assay conditions. The inactivation rate constants, kd, were calculated using the following equation:
A
t/A0 is the residual PG II activity value after time t (At is the activity of heated PG II, and A0 is the initial activity of unheated PG II). The enzyme's half-life values were determined from the equation:
t1/2 = ln 2/kd |
The enzyme inactivation energy (Ed) was obtained from linear modification of the Arrhenius equation by plotting ln
kd against the inverse of absolute temperature (R is the universal gas constant and T is the absolute temperature).
t1/2 = ln 2/kd |
The thermodynamic properties of PG II can be approximated using the Eyring equation, which involves constants: R is the universal gas constant, 8.314 J mol−1 K−1kB (Boltzmann constant, 1.38 × 10−23 J K−1), T (absolute temperature), h (Planck constant, 6.63 × 10−34 Js), ΔH (enthalpy of activation in kJ mol−1), and ΔS (entropy of activation in J mol−1 K−1). The enthalpy of activation (ΔH), specified in the given equation, is determined by calculating the energies of enzyme denaturation, as detailed in the following equations.
The free energy of activation (ΔG) can also be derived from the given equation. Finally, as described, the entropy of activation (ΔS) is calculated using the enthalpy and free energies of activation, as presented in the given equation.
D-value, the decimal reduction time is the time needed for reduction of the initial activity to 10% residual at a given temperature and was calculated using the following equation:
The Z-value, sensitivity factor, represents the temperature increment required to reduce the D-value tenfold. It serves as the slope when the log of the D-value is plotted against the temperature.19
2.8.3 Determination of optimal pH activity and stability.
Enzyme activity in a 50 mM Britton–Robinson buffer series was examined under precise enzyme assay conditions to determine the optimal pH range for PG II. To evaluate pH stability, the enzyme was subjected to controlled incubation at specified pH values between 3.0 and 8.0 at constant temperature (25 °C) for a period of 1, 2 and 7 days. Samples were taken at the indicated time intervals and the residual activity was quantified using established enzyme assay conditions.
2.8.4 Thin layer chromatography.
Thin Layer Chromatography (TLC) was employed to analyse the hydrolysis products of different substrates. For this purpose, various substrates in the concentration of 1% (w/w), including high methylated pectin (HMP), low methylated pectin (LMP), and polygalacturonic acid (PGA), were incubated with a purified enzyme. The hydrolysis process was conducted for 1 and 3 h. Subsequently, the resulting products were analysed on TLC plates (Silica Gel 60 F254, Sigma-Aldrich) using a solvent system comprising n-butanol, ethanol, water, and acetic acid in a 5
:
3
:
2
:
0,5 (v/v/v) ratio. Standards such as monogalacturonate and digalacturonate were utilised. The products were visualised through the application of α-naphthol reagent and heating.
2.9 Fruit juice processing
The preparation of fresh fruit pulp, such as apples, quinces, strawberries, oranges, and bananas, as well as the enzymatic treatment of fruit juices, was carried out following the method described by Pavlović et al. (2023).12 Enzymatic processing of fruit juices aimed at enhancing liquefaction and clarification.
The liquefaction assay utilised the optimal temperature (40 °C) for the PG II from A. tubingensis FAT43. Juice yield was calculated using the equation:
Fresh pulps were homogenised, and enzyme treatment (2 IU per gram of pulp) was conducted at 40 °C for 60 min. Control samples, replacing the enzyme with an acetate buffer, were also prepared. After centrifugation, liquefaction was quantified by measuring the increase in juice volume compared to the control sample. Experiments were triplicated, and mean values with standard deviations were determined for each juice.
After liquefaction of the fruit pulps prepared with the above-described method, a clarification test was performed after filtering the supernatant through filter paper to remove all suspended particles. The transmittance was measured at 660 nm (UV-1800 UV/Vis spectrophotometer; Shimadzu, Kyoto, Japan) in the obtained juices. The percentage of clarification is expressed as the degree of reduction in transmittance compared to juices not treated with a PG II. Experiments were conducted in triplicate, and mean values with standard deviations were calculated for each juice.
2.9.1 Determination of total phenolic content.
The total phenolic content (TPC) of enzymatically treated and untreated juices was measured in accordance with Singleton and Rossi's (1965) method.20 Gallic acid equivalents (GAE) were used to express the TPC as mg per 100 mL juices. The polyphenol concentration in the samples was calculated using a standard curve. The obtained results before and after enzyme treatment were compared using a t-test.
2.9.2 Antioxidant activity of treated and untreated juices.
To investigate changes in the antioxidant properties of juices, FRAP (Ferric Reducing Antioxidant Power) and DPPH (2,2-diphenyl-1-picrylhydrazyl) assays were conducted before and after treatment with polygalacturonase.
To initiate the FRAP test, 100 μL of the sample is mixed with 900 μL of the FRAP reagent, composed of 50 mL of pH 3.6 acetate buffer, 5 mL of tripyridyltriazine (TPTZ), and 5 mL of FeCl3·6H2O. The resulting mixture is left in complete darkness for 5 min to allow the reaction to occur. After 5 min, the absorbance of the mixture is measured at 593 nm. The obtained value is inserted into the standard line to calculate the FRAP value, equivalent to ascorbic acid.
The DPPH test is initiated by combining 10 μL of the sample with 900 μL of the DPPH reagent (1 mM 2,2-diphenyl-1-picrylhydrazyl dissolved in methanol). The reaction is carried out in complete darkness for 15 min. The result of the reaction can be presented in two ways: as a percentage of discoloration or as an equivalent to a standard, such as ascorbic acid. The obtained results before and after enzyme treatment were compared using a t-test.
3. Results and discussion
Although endo-PGs have been extensively studied as enzymes in the commercial pectinolytic complex, there is still a global interest in discovering new and improved enzyme variants for industrial and biotechnological applications. These enzymes are primarily found in fungi, particularly in species of Aspergillus. For instance, various Aspergillus species, including A. niger, A. awamori, A. carbonarius, A. aculeatus, and A. flavus, have been isolated for their endo-PGs properties.21 There is still great demand for highly effective enzymes with a wide pH and temperature range in different industrial processes, such as the fruit juice industry. This study found that A. tubingensis FAT43 isolated from quince fruit surface secretes endo-PGs after four days of growth at 28 °C. Subsequently, these enzymes were detected using the diffusion assay method, which confirmed the presence of endo-PG activity at various time points during the 24, 48, 72, and 96 h of fermentation. The visual representation in the accompanying image illustrates the pectin in the gel stained with RR colour, revealing the hydrolysis of pectin in the illuminated areas (clear zones) due to endo-PG activity. Notably, the diameter of the enlightened (clear) zones indicates an increase in endo-PG activity with prolonged fermentation. The sample subjected to a 96-hour fermentation period exhibited the largest diameter of enlightenment, while the 24-hour sample presented the smallest clear zone diameter (Fig. 2). This comprehensive screening test provides a clear understanding of the temporal dynamics of endo-PG production during the fermentation process.
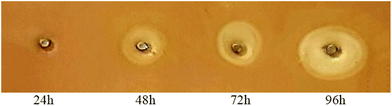 |
| Fig. 2 Diffusion assay for endo-PG activity. | |
3.1 Purification and characterisation of PG II
Polygalacturonase is usually purified from fermentation media in several steps, with standard fractionation methods such as ammonium sulfate (AS) and ethanol precipitation, followed by various column chromatography techniques. Standard methods were also utilised in our purification procedure, including ion-exchange chromatography on a DEAE Sephadex and Q-Sepharose column, to successfully purify the predominant isoform of endo-PG from the fermentation extract (Fig. 3 and Table 1). The two ion-exchange chromatography steps resulted in a purification fold of 3.3 for polygalacturonase with a yield of 16% and specific activity of 6001.67 U mg−1. Different methods were used to purify polygalacturonase from fungi compared to previous studies. For example, PG II was purified from A. tubingensis using DEAE Sephadex chromatography, which resulted in a remarkable 16.8-fold purification, albeit with a lower yield of 13%.22 Similarly, a semi-purification process applied to polygalacturonase from A. japonicas23 resulted in a 2.8-fold purification after two chromatography steps, which is similar to the semi-purified polygalacturonase from A. terreus with yields of 2.5% and 6.2%, respectively.24 These comparative results emphasise the effectiveness of the applied purification strategy in achieving a considerable purification fold for the polygalacturonase of interest, with a competitive yield and specific activity.
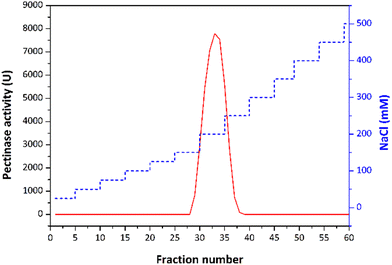 |
| Fig. 3 The elution curve in ion exchange chromatography on Q Sepharose. | |
Table 1 Purification steps PG II
Purification steps |
Total activity (U) |
Total protein (mg) |
Specific activity (U mg−1) |
Purification fold |
Yield (%) |
Crude extract |
255 000 |
140 |
1821.42 |
1 |
100 |
Ethanol precipitation |
182 362.5 |
109.5 |
1665.41 |
0.91 |
71.51 |
AS precipitation |
167 025 |
95.7 |
1709.57 |
0.94 |
65.50 |
SEC (Sephacryl S100 HR) |
92 182.5 |
22.04 |
4182.99 |
2.3 |
36.15 |
IEX (DEAE Spehadex) |
51 127.5 |
10.04 |
5092.33 |
2.8 |
20.50 |
IEX (Q Sepharose) |
40 800 |
6.80 |
6001.67 |
3.3 |
16 |
The crude enzyme preparation with high endo-PG activity was purified to electrophoretic semi-homogeneity, as shown in Fig. 4. The protein fraction with high endo-PG activity was reduced, alkylated, and digested with trypsin. ESI-qTOF-MS analysis identified two peptides of endo-PG II: DITVTGASGHLINCDGAR and LESVTGTVDSK, accounting for 8% of the sequence coverage. These results confirm that the predominant isoform of endo-PG is PG II, which is most abundantly produced in A. tubingensis FAT43. The apparent molecular mass of the protein of ∼38 kDa determined by SDS-gel electrophoresis (Fig. 4) was higher than the theoretically calculated 34.82 kDa, suggesting that it is significantly increased by N-glycosylation.
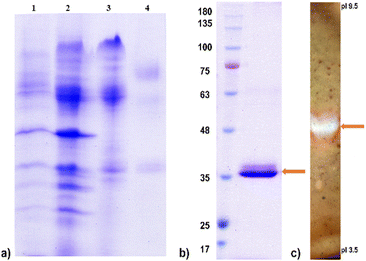 |
| Fig. 4 Protein profiles after electrophoresis separation. (a) Crude enzyme extract (1), resuspended precipitate after precipitation with 70% AS (2), after GF on Sephacryl S100 HR (3), semi-purified PG II by IEX on DEAE Sephadex (4) (b) SDS-PAGE: protein molecular weight markers and purified PG II (∼38 kDa) by IEC on Q Sepharose (c) Zymography analysis after isoelectric focusing. | |
An analysis of all available genome sequences of A. tubingensis from the SWISS-PROT protein sequence database was performed to find the gene coding for PG II (Fig. 5). The search revealed a gene pgaII that is 1086 bp long and codes for a PG II consisting of 362 amino acids. Using genomic DNA samples of A. tubingensis FAT43 and specific primers, two fragments were obtained by PCR. The fragments contained an open reading frame (ORF) that encodes 189 and 153 amino acids, respectively. It is worth noting that the mature protein contains 342 amino acids. Comparative analysis of the PG II sequence is a recurring characteristic observed in different strains of Aspergillus species. However, a comprehensive characterisation of only a limited number of PG II enzymes from the genus Aspergillus can be found in the literature.25
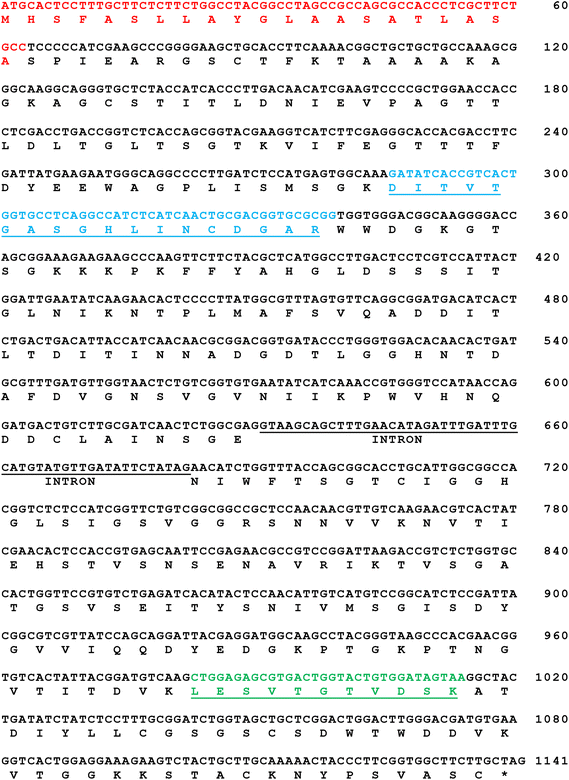 |
| Fig. 5 Gene sequence of PG II (342 amino acids) with ORF fragments encoding 189 and 153 amino acids. The black lined sequence denotes the intronic region, and red marks indicate the leader peptide. Peptide fragments from MS analysis are highlighted in blue and green. | |
Since the two peptides identified by MS analysis show 100% identity with the corresponding part of the sequence of the known PG II from A. tubingensis (GenBank accession number X58894), we investigated whether the FAT43 strain has an identical gene in its genome. Appropriate primers based on the known sequence (for the first and the second exon) were designed and PCR amplification was performed under stringent conditions. The successful amplification of DNA fragments of the expected size (570 and 500 bp) indicates with high confidence that strain FAT 43 possesses a pgaII gene that is highly identical to that of A. tubingensis (GenBank accession number X58894).
The expression of the pgaII gene is strongly regulated by the carbon source. In a minimal medium containing simple sugars such as sucrose, glucose, xylose, or galacturonic acid, both A. niger N400 and A. tubingensis NW756 showed minimal or negligible PG activity. Interestingly, a substantial induction of endo-PG production was observed in A. tubingensis FAT 43 when pectin-containing substances such as sugar beet pulp were used as a carbon source, similar to the trend observed in A. niger N400 and A. tubingensis NW756.25
Five different endo-PGs from A. niger and A. tubingensis have been described in the literature, with PG I and PG II proving to be the predominant enzymatic activities with isoelectric points around 5–6 and 3.5, respectively.25 The lower isoelectric point probably corresponds to PG I. PGs with higher isoelectric points from A. tubingensis FAT 43 are very similar to A. tubingensis NW756 and A. niger N400 PGs and have an apparent molecular mass identical to that of PG II from A. tubingensis FAT 43 (∼38 kDa). However, these enzymes differ, as shown by the slightly higher isoelectric point of both A. tubingensis FAT43 and A. tubingensis NW756 PG II (∼6.0) compared to A. niger N400 PG II (5.2).25
3.2 Optimal pH and temperature for the PG II activity and stability
The optimal temperature for fungal PG varies depending on the source and environmental conditions. However, these enzymes generally are active within a wide temperature range. The catalytic activity of PG II was systematically investigated at temperatures between 30 and 60 °C, with the highest enzyme activity observed at 45 °C and 90% of the maximum activity maintained at 50 °C (Fig. 6a). It is noteworthy that for PGs of F. oxysporum and A. tubingensis isolated from banana and peach, respectively, a congruent temperature optimum in the range of 40–50 °C was observed.22 The activation energy calculated from the Arrhenius equation is an important parameter and indicates the stability of the enzyme to temperature change. The calculated Ea of isolated PG II from the Arrhenius plot was 62.1 kJ mol−1.
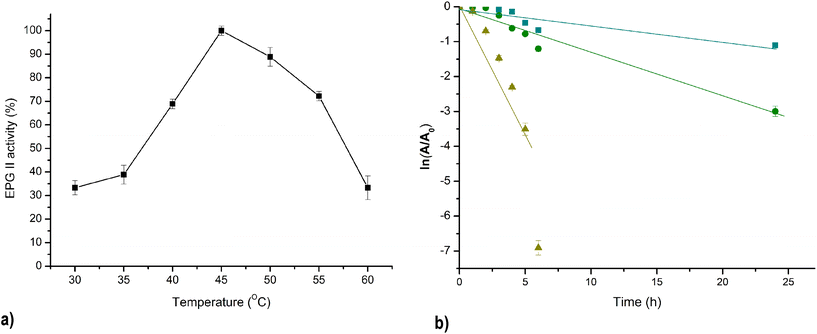 |
| Fig. 6 Effect of temperature on PG II activity: T optimum (a) and thermal deactivation; – 40 °C, – 50 °C, – 60 °C(b). | |
Fig. 6b shows the inactivation kinetics of PG II at different incubation temperatures of 40 °C, 50 °C, and 60 °C. The logarithmic transformation of the data shows a linear relationship, indicating that the thermal inactivation of PG II is a first-order kinetic process. The enzyme's half-life at 40 °C and 50 °C was 13.7 h and 5.4 h, respectively. However, at a higher temperature of 60 °C, the enzyme's half-life was significantly decreased to 0.85 h. The obtained values for the half-life at 40 °C are higher than those previously published in the literature, as well as at temperatures of 50 °C and 60 °C, except in the case of Pectinase from A. niger NRC1ami, which exhibits better thermostability at 50 °C and the same as PG II at 60 °C (Table 2). The thermal denaturation process requires a minimum energy, referred to as the activation energy of denaturation (Ed). When the initial energy input is below Ed, the unstable intermediate, which begins to desaturate, has the capability to fold to the native enzyme structure upon cooling. However, once this Ed barrier is surpassed, the enzyme undergoes denaturation and loses its ability to fold back to its native form. The calculated energy of denaturation PG II was 32.8 kJ mol−1 which is a much higher value compared to Ed of pectinase isolated from A. niger NRC1ami.26 Because Ed is directly related to the activation enthalpy of denaturation (ΔH), which expresses the total amount of energy required to denature the enzyme, large and positive values of Ed and ΔH should be associated with high enzyme thermostability.27Table 2 shows the results of ΔH and other thermodynamic parameters of thermal denaturation of PG II at temperatures ranging from 40 to 60 °C. Negative ΔS and ΔH positive values suggested that the denaturation process is not spontaneous at a given temperature and, the enzyme inactivation can be reversible.28 The same relation between calculated ΔS and ΔH was reported previously for pectinase derived from A. niger isolated from orange, but the stability of this enzyme was low even after a chemical modification that resulted in a minimal increase in enzyme stability.26 The thermal stability of the enzyme isolated in this research was higher than previously reported based on the D-values comparison, which is higher than other research groups reported.19,26
Table 2 Thermodynamic and kinetic parameters for thermal deactivation of PG II
|
Temperature |
Inactivation rate constants, kd (h−1) |
t
1/2 (h) |
ΔH (kJ mol−1) |
ΔG (kJ mol−1) |
ΔS (J mol−1 K−1) |
D value (h) |
Z value ( °C) |
Ref. |
PG II, Aspergillus tubingensis FAT43 |
40 °C |
0.05062 |
13.7 |
30.20 |
63.24 |
−105.6 |
45.5 |
16.58 |
This paper |
50 °C |
0.1294 |
5.36 |
30.11 |
62.82 |
−101.3 |
17.8 |
60 °C |
0.8144 |
0.85 |
30.03 |
59.76 |
−89.27 |
2.83 |
Rapidase C80 (Gist–Brocades) |
40 °C |
0.1980 |
3.50 |
143 |
102–98.5 |
130 |
|
|
29
|
50 °C |
1.2378 |
0.56 |
60 °C |
5.3319 |
0.13 |
Pectinase CCM (Biocon) |
40 °C |
0.0990 |
7.00 |
73.9 |
104–105 |
−95.5 |
|
|
|
50 °C |
0.1873 |
3.70 |
60 °C |
0.6730 |
1.03 |
Pectinex 3XL (Novozyme) |
40 °C |
0.1540 |
4.50 |
157 |
103–99.8 |
176 |
|
|
|
50 °C |
1.4440 |
0.48 |
60 °C |
|
|
Pectinase, Aspergillus nigerNRC1ami |
50 °C |
0.3466 |
2.00 |
11.89 |
85.6 |
−4.22 |
6.60 |
|
26
|
60 °C |
0.8155 |
0.85 |
11.81 |
85.97 |
−3.70 |
2.45 |
Endo-PG (PG8fn), Achaetomium sp. strain Xz8 |
50 °C |
0.4332 |
1.6 |
|
|
|
|
|
30
|
55 °C |
1.2603 |
0.55 |
|
|
|
|
PG, E. carotovora subsp. carotovora (Ecc) BR1 |
40 °C |
0.3332 |
2.08 |
42.60 |
84.23 |
−133.04 |
|
|
31
|
50 °C |
0.3707 |
1.87 |
85.56 |
|
|
60 °C |
0.9002 |
0.77 |
86.10 |
|
|
The prevailing characterisation of PGs currently describes a predominant pattern of significantly low thermal stability. A study by da Câmara Rocha et al. (2020) showed that A. niger exhibits sustained PG activity over 2 h at 30 °C and 40 °C within the pH range of 4.0–6.0.32 Similarly, Vaz et al. (2021) reported that the PG of A. terreus reaches its half-life after 2 h of incubation at 40 °C,24 mirroring the behaviour observed in the PG from A. japonicus at 50 °C.23 However, the mandatory transition to PG variants for industrial applications requires an improvement in thermostability. Conventional acidic mesothermal PGs are susceptible to thermal inactivation from 50 °C to 65 °C, with enzymatic activity decreasing within 10 to 60 min. This emphasises the need for enzymes with increased thermal resistance in industrial contexts. In particular, PG II isolated from A. tubingensis FAT 43 proves to be a promising candidate as it exhibits better thermostability compared to a broader range of characterised PG enzymes. These properties make PG II a potential asset for applications in industries where PGs with high thermostability requirements are essential.
The pH activity profile of PG varies greatly depending on the producing organism. The low pH optimum for Aspergillus PG activity was obtained in our findings. The maximum PG II A. tubingensis FAT43 activity towards polygalacturonic acid was noticed over a pH range of 3.5 to 4.5, as shown in Fig. 7a. A similarly broad pH optimum was found for PG from A. niger, which showed a pH optimum in the range of 4.3 to 4.9.33 This range is consistent with the pH conditions found in many plant tissues.
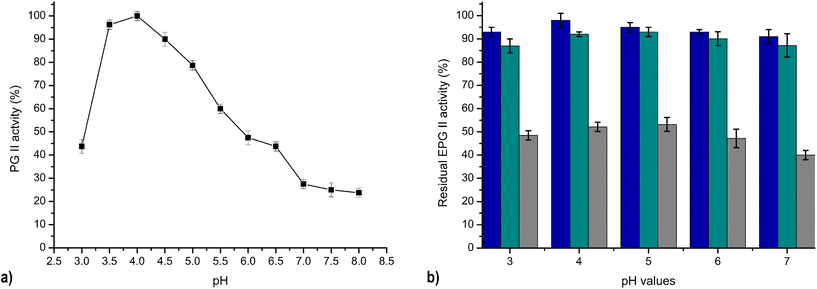 |
| Fig. 7 Effect of pH on PG II activity: pH optimum (a) and pH stability; blue – 24h, green – 48h, grey – 7 days (b). | |
The pH stability of PG II was measured over a broad pH range from 3 to 7, as shown in Fig. 7b. The results elucidate that PG II retains its full enzymatic activity at a pH of 4, and has 91–95% of its original activity at the other pH values after one day. Remarkably, the residual activity of PG II remained consistently high under the same conditions for all pH values tested, ranging between 87–93% and 40–53% of the original activity after 2 and 7 days, respectively (Fig. 7b). The significant lower pH stability of purified PG from A. niger NRC1ami was reported in the literature in the same pH range after 2 h of incubation,26 while polygalacturonase from A. japonicus showed high stability in pH range 3–6 during 24 h incubation at 4 °C.23 The sustained enzymatic activity across different pH values, coupled with robust stability, is of utmost importance for potential industrial applications. In juice processing, the intrinsic acidities of different fruit types lead to different initial pH values. The liquefaction step in this industrial process is usually limited to acidic pH conditions. Consequently, the subsequent processing steps, such as liquefaction and clarification of the juices, require pH adjustment, often to pH 5. Eliminating the pH adjustment step would greatly simplify the process and contribute significantly to economic efficiency, which represents a significant challenge for ongoing research. In this context, the pectinolytic enzyme PG II, characterised by its stability and activity at low pH values, appears promising for integration into industrial processes. Its compatibility with low pH conditions makes it possible to carry out all processing phases simultaneously and avoid additional pH adjustment steps. This emphasises the practical applicability of PG II and represents a potential opportunity to improve the efficiency and cost-effectiveness of industrial juice processing.
3.3 Hydrolysis of different pectins
The composition of pectin in fruit juices is influenced by various factors, such as the type of fruit, its degree of ripeness, and the method used for juice extraction. Pectin is a complex polysaccharide found in plant cell walls. There are different types of pectins, including homogalacturonans, rhamnogalacturonans and xylogalacturonans, each of which contributes to the unique character of fruit pectins. Homogalacturonans are the most abundant type. Polygalacturonate is a special form of pectin produced by the breakdown of homogalacturonan. The addition of methyl and acetyl groups to the galacturonic acid units in the pectin structure has a significant impact on the physicochemical properties of pectin, including solubility and gelation. These modifications lead to the intricate details of pectin in fruit juices. The hydrolysis products of these complex substrates can be analysed using TLC, a useful tool for studying the dynamic changes that occur during enzymatic hydrolysis processes. Purified PG II was used to perform enzymatic hydrolysis experiments with polygalacturonate, low-methylated pectin and high-methylated pectin. The resulting hydrolysis products are found to consist exclusively of mono- and digalacturonates (Fig. 8). It is worth noting that the highest hydrolysis specificity was observed with the non-methylated substrate, polygalacturonic acid. Accordingly, the specificity of hydrolysis decreased with increasing degree of methylation, which was reflected in significantly lower yields of hydrolysis products for both low- and high-methylated pectin. These results are consistent with previous studies indicating that an increase in the degree of methylation is associated with a decrease in the efficiency of substrate hydrolysis.34
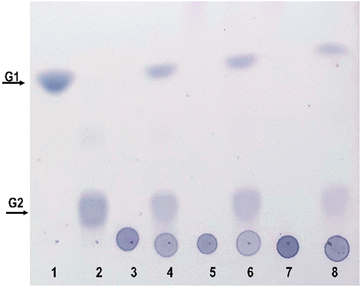 |
| Fig. 8 TLC analysis of hydrolytic products of PGII. Standard markers: G1-galacturonic acid (1) and G2-digalacturonic acid (2). Unhydrolysed and hydrolysed substrates: PGA, (3 and 4) LMP (5 and 6), and HMP (7 and 8). | |
3.4 Fruit processing with PG II
This study investigates the potential of the focus on optimising juice yield, improving clarity and investigating possible changes in antioxidant capacity. Experimental analyses were conducted on quince, apple, banana, strawberry, and orange to investigate the effects of enzyme on key aspects of fruit juice production. The pectin content of the individual fruits varies. Reported inconsistencies in measuring the pectin content of these fruits render categorization based solely on pectin content unreliable. These inconsistencies stem from variations in measurement methodologies; for instance, some studies measure peel pectin content, while others assess dry weight values or soluble pectins instead of total pectins. Moreover, pectin levels may fluctuate depending on the fruit's ripeness. Consequently, relying solely on pectin content for fruit categorization may not accurately depict their true composition.35–37
The liquefaction and clarification of fruit juices utilising pectinases depends on the fruit's various pectins and other polysaccharides that serve as substrates for the enzyme activity.38 Pectins are initially present in the cell wall of strawberries in a highly methylated form. They are then de-esterified by the activity of pectin methyl esterase, an enzyme found in strawberries, which increases the number of acidic pectins that are a suitable substrate for the activity PG II. The highest juice yield was achieved with strawberries. The yield of the treated juice increased by 25% compared to the untreated juice. Apples, oranges and bananas have highly methylated pectin (degree of methylation above 50%).39–41 The results of clarification of these juices are presented in Table 3 and Fig. 9.
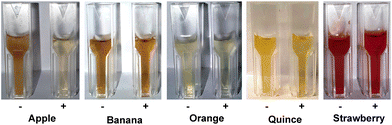 |
| Fig. 9 The appearance of fruit juices after enzyme treatment, (−) untreated and (+) treated (b). | |
Table 3 The improvement of juice clarification after their treatment with PG II
Pectinase, source |
Apple (%) |
Banana (%) |
Orange (%) |
Quince (%) |
Strawberry (%) |
Ref |
PGII, A. tubingensis FAT 43 |
90.0 |
19.0 |
5.8 |
11.2 |
51.4 |
This paper |
PGAj, A. japonicus |
9.4 |
10.8 |
|
|
|
23
|
exo-PG, A. niger MTCC 478 |
|
|
27.0 |
|
|
43
|
PG, A. awamori Nakazawa MTCC 6652 |
66.4 |
|
|
|
|
44
|
PGP2, A. niger |
|
|
42 |
|
|
45
|
Pectinase, A. niger LB-02-SF |
|
|
|
|
60 |
46
|
The treatment of orange juice resulted in a percentage clarification of 87.1% clarity and a yield increase of 12.5%. The yield of the treated apple juice increased by 15.83%, and the clarity of the juice was an excellent 95%. The yield of banana juice increased by 21%, and 76.1% was clarified. Quince has a significantly methylated pectin (degree of methylation >85%),42 which could be a sign of a lower efficiency of PG II in liquefying this juice (10% higher juice yield).
Antioxidants are crucial in slowing down, controlling, or preventing oxidation processes that can lead to the deterioration of food quality or trigger and spread inflammatory diseases.47 Various assays with different mechanisms can assess antioxidant activity. This study evaluated the antioxidant activities of untreated and enzyme-treated fruit juice by determining their DPPH radical scavenging activities and FRAP (Table 4). The statistically significant results for TPC, DPPH and FRAP before and after enzyme treatment were compared using the Student`s t-test. Based on the obtained p-values, they were classified into two groups.
Table 4 Antioxidant activities of untreated and PG II treated fruit juices
Fruit juice |
DPPH (mmol AAE) |
FRAP (mmol AAE) |
TPC (μmol GAE) |
Untreated |
Treated |
p-value |
Untreated |
Treated |
p-value |
Untreated |
Treated |
p-value |
Apple |
0.74 ± 0.02 |
0.89 ± 0.03 |
0.002 |
0.13 ± 0.01 |
0.16 ± 0.01 |
0.127 |
56 ± 2 |
65 ± 2 |
0.005 |
Banana |
1.16 ± 0.04 |
1.20 ± 0.05 |
0.340 |
1.00 ± 0.02 |
0.96 ± 0.03 |
0.178 |
958 ± 32 |
1002 ± 41 |
0.217 |
Orange |
2.75 ± 0.09 |
2.89 ± 0.10 |
0.146 |
6.32 ± 0.24 |
6.64 ± 0.24 |
0.148 |
885 ± 31 |
885 ± 24 |
1.000 |
Strawberry |
3.51 ± 0.12 |
3.69 ± 0.13 |
0.153 |
1.70 ± 0.28 |
1.51 ± 0.32 |
0.017 |
1500 ± 51 |
2027 ± 45 |
0.0002 |
Quince |
1.11 ± 0.04 |
1.63 ± 0.04 |
0.0001 |
1.99 ± 0.07 |
2.23 ± 0.08 |
0.127 |
479 ± 18 |
498 ± 15 |
0.233 |
A statistically significant difference, or an increase in the content of total phenols and/or antioxidative activity after enzyme treatment, was observed when the p-value < 0.05. In cases where the p-value > 0.05, there was no statistically significant increase in these values. The content of total phenols differs in the PG II-treated juices compared to the untreated juices, with the exception of orange juice, where there is no change in the phenolic composition of the juice. After enzymatic treatment, strawberry juice showed the greatest increase in phenolic components, consistent with the juice's strongest antioxidant potential, as demonstrated by DPPH and FRAP tests. The antioxidant activity directly depends on the concentration of POS and its composition.48,49 This result is in contrast with the findings of a study by Sandri and da Salveira 201846 according to which the addition of pectinases from A. niger to strawberry juice did not increase the antioxidant capacity of the juice. However, the most significant increase in antioxidant capacity was obtained in the treatment of quince (47%) and apple (20%) juice. Treatment of the other juices (orange and banana) also increased antioxidant activity. The increase in antioxidant activity of fruit juice could be due to improved extraction of antioxidants such as phenols and carotenoids from the cell cytoplasm.50 Pectinase plays a crucial role in the breakdown of the middle lamella of the cell and primary wall and facilitates the release of polyphenolic compounds and carotenoids from the cells. An improvement in the antioxidant potential of fruit juice after enzyme treatment has already been observed in apricot and raspberry juices.50,51 The increase in the antioxidant potential of pectin after pectinase treatment or the antioxidant activity of the field compared to the original pectin has been previously demonstrated in the literature.52 In addition, previous studies have shown that electron-donating groups such as carboxyl, hydroxyl, and methoxy groups can enhance the antioxidant properties of a sample.53 While the free radical scavenging activity of hydroxyl groups in polysaccharides is relatively low due to the lack of a phenolic structure, numerous other factors, including the presence of galacturonic acid and other chemical components in polysaccharides, are thought to play an important role in their antioxidant activities.54
4. Conclusion
The optimal temperature range (30–60 °C) for the activity of PG II, with a peak at 45 °C and remarkable thermal stability, makes it a versatile enzyme for industrial applications. The enzyme's pH activity profile, which shows a low optimum pH (3.5–4.5) and robust stability over a broad pH spectrum (3–7), emphasises its adaptability to different processing conditions. The high specificity of PG II for the hydrolysis of non-methylated substrates indicates its effectiveness in various enzymatic processes. In the processing of fruit juices, experiments with different fruit juices showed the effectiveness of PG II in liquefaction and clarification, resulting in increased yield and clarity. Strawberry juice, in particular, showed the greatest influence on the increase in yield. In addition, the application of PG II increased antioxidant activity in the treated juices, with significant improvements observed in strawberry, quince, and apple juices, suggesting potential health-promoting benefits. PG II derived from A. tubingensis FAT43-derived is a promising enzyme with favourable properties for industrial processes, especially fruit juice processing. Its versatility, improved thermal stability and adaptability to different pH conditions make PG II a valuable asset for potential applications in biotechnology. Further studies could explore its synergies with other enzymes and broader industrial applications.
Author contributions
Conceptualization: M. P. and M. Š. S.; formal analysis: M. P. and A. M.; funding acquisition: Z. V. and I. K.; investigation: M. P., M. Š. S. and A. L.; methodology: A. M., M. Š. S. and M. K.; supervision: Z. V. and I. K.; validation: A. M.; writing – original draft: M. P., M. Š. S. and A. M.; writing – review & editing: M. K. and A. L.
Conflicts of interest
There are no conflicts to declare.
Acknowledgements
The authors express their gratitude to Marina Ristović from the Institute of Chemistry, Technology and Metallurgy Department of Chemistry, Belgrade, Republic of Serbia, for their help in determining the antioxidant activity of juices. This work was financially supported by the Ministry of Science, Technological Development and Innovation of the Republic of Serbia Contract numbers: 451-03-47/2023-01/200026, 451-03-47/2023-01/200168, 451-03-47/2023-01/200177; and by the Slovenian Research and Innovation Agency grant P1-0207.
References
- S. Haile and A. Ayele, Pectinase from Microorganisms and Its Industrial Applications, Sci. World J., 2022, 2022, 1–15 Search PubMed.
-
W. Pilnik and A. G. J. Voragen, in Enzymes in Food Processing, Elsevier, 1993, pp. 363–399 Search PubMed.
- P. Blanco, Production of pectic enzymes in yeasts, FEMS Microbiol. Lett., 1999, 175, 1–9 CrossRef CAS PubMed.
- R. S. Jayani, S. Saxena and R. Gupta, Microbial pectinolytic enzymes: A review, Process Biochem., 2005, 40, 2931–2944 CrossRef CAS.
- M. Pinelo, B. Zeuner and A. S. Meyer, Juice clarification by protease and pectinase treatments indicates new roles of pectin and protein in cherry juice turbidity, Food Bioprod. Process., 2010, 88, 259–265 CrossRef CAS.
- M. Mutlu, K. Sarioǧlu, N. Demir, M. T. Ercan and J. Acar, The use of commercial pectinase in fruit juice industry. Part I: viscosimetric determination of enzyme activity, J. Food Eng., 1999, 41, 147–150 CrossRef.
- X. Lu, J. Lin, C. Wang, X. Du and J. Cai, Purification and characterization of exo-polygalacturonase from Zygoascus hellenicus, V25 and its potential application in fruit juice clarification, Food Sci. Biotechnol., 2016, 25, 1379–1385 CrossRef CAS PubMed.
- S. Shrestha, Md. S. Rahman and W. Qin, New insights in pectinase production development and industrial applications, Appl. Microbiol. Biotechnol., 2021, 105, 9069–9087 CrossRef CAS PubMed.
- H. P. Sharma, H. Patel and Sugandha, Enzymatic added extraction and clarification of fruit juices–A review, Crit. Rev. Food Sci. Nutr., 2017, 57, 1215–1227 CrossRef CAS PubMed.
- S. Liu, L. Tian, Y. Cong, Q. Shi, L. Wang, Y. Lu, L. Wang and G. Yang, Recent advances in polygalacturonase: Industrial applications and challenges, Carbohydr. Res., 2023, 528, 108816 CrossRef CAS PubMed.
- F. Amin, A. Mohsin, H. N. Bhatti and M. Bilal, Production, thermodynamic characterization, and fruit juice quality improvement characteristics of an Exo-polygalacturonase from Penicillium janczewskii, Biochim. Biophys. Acta, Proteins Proteomics, 2020, 1868, 140379 CrossRef CAS PubMed.
- M. Pavlović, M. Šokarda Slavić, M. Ristović, S. Stojanović, A. Margetić, M. Momčilović and Z. Vujčić, Optimization of solid-state fermentation for enhanced production of pectinolytic complex by Aspergillus tubingensis FAT43 and its application in fruit juice processing, Lett. Appl. Microbiol., 2023, 76, 1–10 CrossRef PubMed.
- S. Stojanović, M. Ristović, J. Stepanović, A. Margetić, B. Duduk, Z. Vujčić and B. Dojnov,
Aspergillus welwitschiae inulinase enzyme cocktails obtained on agro-material inducers for the purpose of fructooligosaccharides production, Food Res. Int., 2022, 160, 111755 CrossRef PubMed.
- S. Stojanović, J. Stepanović, B. Špirović Trifunović, N. Duduk, B. Dojnov, B. Duduk and Z. Vujčić, Selection of Non-Mycotoxigenic Inulinase Producers in the Group of Black Aspergilli for Use in Food Processing, Food Technol. Biotechnol., 2022, 60, 421–433 Search PubMed.
- M. M. Bradford, A rapid and sensitive method for the quantitation of microgram quantities of protein utilizing the principle of protein-dye binding, Anal. Biochem., 1976, 72, 248–254 CrossRef CAS PubMed.
- P. Bernfeld, Amylases α and β, Methods Enzymol., 1955, 1, 149–158 CAS.
- U. K. Laemmli, Cleavage of structural proteins during the assembly of the head of bacteriophage T4, Nature, 1970, 227, 680–685 CrossRef CAS PubMed.
- J. P. Day and R. C. Shattock, Aggressiveness and other factors relating to displacement of populations of Phytophthora infestans in England and Wales, Eur. J. Plant Pathol., 1997, 103, 379–391 CrossRef.
- R. L. de Oliveira, O. S. da Silva, A. Converti and T. S. Porto, Thermodynamic and kinetic studies on pectinase extracted from Aspergillus aculeatus: Free and immobilized enzyme entrapped in alginate beads, Int. J. Biol. Macromol., 2018, 115, 1088–1093 CrossRef PubMed.
- V. L. Singleton and J. A. Rossi, Colorimetry of total phenolics with phosphomolybdic-phosphotungstic acid reagent, Am. J. Enol. Vitic., 1965, 16, 144–158 CrossRef CAS.
- S. K. Niture, Comparative biochemical and structural characterizations of fungal polygalacturonases, Biologia, 2008, 63, 1–19 CrossRef CAS.
- A. R. Al-Najada, R. R. Al-Hindi and S. A. Mohamed, Characterization of polygalacturonases from fruit spoilage Fusarium oxysporum and Aspergillus tubingensis, Afr. J. Biotechnol., 2012, 11, 8527–8536 CAS.
- N. C. de A. Guimarães, N. N. Glienke, R. M. S. Galeano, R. Ruller, F. F. Zanoelo, D. C. Masui and G. C. Giannesi, Polygalacturonase from Aspergillus japonicus (PGAj): Enzyme production using low-cost carbon source, biochemical properties and application in clarification of fruit juices, Biocatal. Agric. Biotechnol., 2022, 39, 102233 CrossRef.
- R. P. Vaz, A. C. Vici, M. L. T. M. Polizeli, P. O. Magalhães and E. X. F. Filho, Immobilization studies of a pectinase produced by Aspergillus terreus, Biotechnol. Appl. Biochem., 2021, 68, 197–208 CrossRef CAS PubMed.
- H. J. D. Bussink, F. P. Buxton and J. Visser, Expression and sequence comparison of the Aspergillus niger and Aspergillus tubigensis genes encoding polygalacturonase II, Curr. Genet., 1991, 19, 467–474 CrossRef CAS PubMed.
- M. A. Esawy, A. A. Gamal and Z. Kamel, Optimization of Aspergillus niger NRC1ami Pectinase Using Citrus Peel Pectin, Purification, and Thermodynamic Characterization of the Free and Modified Enzyme, Waste Biomass Valorization, 2022, 13, 4823–4837 CrossRef CAS.
- P. M. Souza, B. Aliakbarian, E. X. F. Filho, P. O. Magalhães, A. P. Junior, A. Converti and P. Perego, Kinetic and thermodynamic studies of a novel acid protease from Aspergillus foetidus, Int. J. Biol. Macromol., 2015, 81, 17–21 CrossRef CAS PubMed.
- M. Melikoglu, C. S. K. Lin and C. Webb, Kinetic studies on the multi-enzyme solution produced via solid state fermentation of waste bread by Aspergillus awamori, Biochem. Eng. J., 2013, 80, 76–82 CrossRef CAS.
- N. Ortega, S. de Diego, M. Perez-Mateos and M. D. Busto, Kinetic properties and thermal behaviour of polygalacturonase used in fruit juice clarification, Food Chem., 2004, 88, 209–217 CrossRef CAS.
- T. Tu, H. Luo, K. Meng, Y. Cheng, R. Ma, P. Shi, H. Huang, Y. Bai, Y. Wang, L. Zhang and B. Yao, Improvement in Thermostability of an Achaetomium sp. Strain Xz8 Endopolygalacturonase via the Optimization of Charge-Charge Interactions, Appl. Environ. Microbiol., 2015, 81, 6938–6944 CrossRef CAS PubMed.
- S. Nerurkar, Biochemical and thermal stabilization parameters of polygalacturonase from Erwinia carotovora subsp. carotovora BR1, J. Mcrobiol. Biotechnol., 2010, 20, 1077–1085 CrossRef PubMed.
- J. C. Rocha, J. S. Araújo, W. K. V. de Paiva, E. S. S. Ribeiro, C. E. de A. Padilha, C. F. de Assis, E. S. dos Santos, G. R. de Macêdo and F. C. de S. Junior, Yellow mombin pulp residue valorization for pectinases production by Aspergillus niger IOC 4003 and its application in juice clarification, Biocatal. Agric. Biotechnol., 2020, 30, 101876 CrossRef.
- H. C. Kester and J. Visser, Purification and characterization of polygalacturonases produced by the hyphal fungus Aspergillus niger, Biotechnol. Appl. Biochem., 1990, 12, 150–160 CAS.
- M.-J. Hao, D. Wu, Y. Xu, X.-M. Tao, N. Li and X.-W. Yu, A Novel Endo-Polygalacturonase from Penicillium rolfsii with Prebiotics Production Potential: Cloning, Characterization and Application, Foods, 2022, 11, 3469 CrossRef CAS PubMed.
- R. A. Baker, Reassessment of Some Fruit and Vegetable Pectin Levels, J. Food Sci., 1997, 62, 225–229 CrossRef CAS.
- M. Thomas, F. Guillemin, F. Guillon and J.-F. Thibault, Pectins in the fruits of Japanese quince (Chaenomeles japonica), Carbohydr. Polym., 2003, 53, 361–372 CrossRef CAS.
- C. Lara-Espinoza, E. Carvajal-Millán, R. Balandrán-Quintana, Y. López-Franco and A. Rascón-Chu, Pectin and Pectin-Based Composite Materials: Beyond Food Texture, Molecules, 2018, 23, 942 CrossRef PubMed.
- F. Vaillant, P. Millan, G. O'Brien, M. Dornier, M. Decloux and M. Reynes, Crossflow microfiltration of passion fruit juice after partial enzymatic liquefaction, J. Food Eng., 1999, 42, 215–224 CrossRef.
- M. H. Canteri-Schemin, H. C. R. Fertonani, N. Waszczynskyj and G. Wosiacki, Extraction of pectin from apple pomace, Braz. Arch. Biol. Technol., 2005, 48, 259–266 CrossRef CAS.
- S. Singhal and N. R. S. Hulle, Citrus pectins: Structural properties, extraction methods, modifications and applications in food systems – A review, Appl. Food Res., 2022, 2, 100215 CrossRef CAS.
- N. S. Rajendran and B. S. Harikumaran Thampi, Pectin—Extraction from underground stem of banana and its structural, rheological, and textural analyses and grading, J. Food Process. Preserv., 2021, 45, e15332 CAS.
- N. El Fihry, K. El Mabrouk, M. Eeckhout, H. A. Schols and H. Hajjaj, Physicochemical, structural, and functional characterization of pectin extracted from quince and pomegranate peel: A comparative study, Int. J. Biol. Macromol., 2024, 256, 127957 CrossRef CAS PubMed.
- G. Anand, S. Yadav and D. Yadav, Production, purification and biochemical characterization of an exo-polygalacturonase from Aspergillus niger MTCC 478 suitable for clarification of orange juice, 3 Biotech, 2017, 7, 122 CrossRef PubMed.
- T. B. Dey, S. Adak, P. Bhattacharya and R. Banerjee, Purification of polygalacturonase from Aspergillus awamori Nakazawa MTCC 6652 and its application in apple juice clarification, LWT, 2014, 59, 591–595 CrossRef CAS.
- Y. Q. Almulaiky, Polygalacturonase by Aspergillus Niger Using Seaweed Waste Under Submerged Fermentation: Production, Purification and Characterization, Biomed. J. Sci. Tech. Res., 2020, 25, 19416–19422 Search PubMed.
- I. G. Sandri and M. M. Silveira, Production and Application of Pectinases from Aspergillus niger Obtained in Solid State Cultivation, Beverages, 2018, 4, 48 CrossRef CAS.
- F. Shahidi and Y. Zhong, Measurement of antioxidant activity, J. Funct. Foods, 2015, 18, 757–781 CrossRef CAS.
- H. Abari, H. Amini Rourani, S. M. Ghasemi, H. Kim and Y.-G. Kim, Investigation of antioxidant and anticancer activities of unsaturated oligo-galacturonic acids produced by pectinase of Streptomyces hydrogenans YAM1, Sci. Rep., 2021, 11, 8491 CrossRef PubMed.
- C. Sabater, A. Blanco-Doval, A. Montilla and N. Corzo, Optimisation of an enzymatic method to obtain modified artichoke pectin and pectic oligosaccharides using artificial neural network tools. In silico and in vitro assessment of the antioxidant activity, Food Hydrocolloids, 2021, 110, 106161 CrossRef CAS.
- O. Bashir, S. Z. Hussain, G. Gani, N. Jan, A. H. Rather, M. Reshi and T. Amin, Evaluating the physicochemical and antioxidant characteristics of apricot juice prepared through pectinase enzyme-assisted extraction from Halman variety, J. Food Meas. Charact., 2021, 15, 2645–2658 CrossRef.
- M. E. Z. Hansen and L. Laroze, Temperature effect on phenolic antioxidant extraction from raspberry wastes assisted by enzymes, N. Biotechnol., 2009, 25, S170 CrossRef.
- V. Chandel, D. Biswas, S. Roy, D. Vaidya, A. Verma and A. Gupta, Current Advancements in Pectin: Extraction, Properties and Multifunctional Applications, Foods, 2022, 11, 2683 CrossRef CAS PubMed.
- J. Chen, J. Yang, L. Ma, J. Li, N. Shahzad and C. K. Kim, Structure-antioxidant activity relationship of methoxy, phenolic hydroxyl, and carboxylic acid groups of phenolic acids, Sci. Rep., 2020, 10, 2611 CrossRef CAS PubMed.
- J. Wang, S. Hu, S. Nie, Q. Yu and M. Xie, Reviews on Mechanisms of In Vitro Antioxidant Activity of Polysaccharides, Oxid. Med. Cell. Longevity, 2016, 2016, 1–13 Search PubMed.
|
This journal is © The Royal Society of Chemistry 2024 |