DOI:
10.1039/D3FO04714H
(Paper)
Food Funct., 2024,
15, 1460-1475
Orally administered neohesperidin attenuates MPTP-induced neurodegeneration by inhibiting inflammatory responses and regulating intestinal flora in mice†
Received
1st November 2023
, Accepted 10th January 2024
First published on 13th January 2024
Abstract
Parkinson's disease (PD), a neurodegenerative disease, is the leading cause of movement disorders. Neuroinflammation plays a critical role in PD pathogenesis. Neohesperidin (Neo), a natural flavonoid extracted from citric fruits exhibits anti-inflammatory effects. However, the effect of Neo on PD progression is unclear. This study aimed to investigate the effects of Neo on 1-methyl-4-phenyl-1,2,3,6-tetrahydropyridine (MPTP)-induced PD in mice and its underlying mechanism. Our results indicated that Neo administration ameliorated motor impairment and neural damage in MPTP-injected mice, by inhibiting neuroinflammation and regulating gut microbial imbalance. Additionally, Neo administration reduced colonic inflammation and tissue damage. Mechanistic studies revealed that Neo suppressed the MPTP-induced inflammatory response by inhibiting excessive activation of NF-κB and MAPK pathways. In summary, the present study demonstrated that Neo administration attenuates neurodegeneration in MPTP-injected mice by inhibiting inflammatory responses and regulating the gut microbial composition. This study may provide the scientific basis for the use of Neo in the treatment of PD and other related diseases.
Introduction
Parkinson's disease (PD), the second most common neurodegenerative disease worldwide, mainly affects those middle-aged and older. Currently, the prevalence of PD in people over 65 years of age exceeds 1.5% and is increasing annually. Patients with PD exhibit pronounced motor dysfunction characterized by rigidity and bradykinesia, seriously diminishing their quality of life.1,2 The etiology of PD is unclear, but genetics, aging, and environmental factors are considered predisposing factors. These factors could lower immunity, promoting immune dysregulation, mitochondrial dysfunction, and oxidative stress. Subsequently, degeneration of nigrostriatal dopaminergic neurons occurs, and the release of striatal dopamine (DA) decreases, causing PD symptoms.3–5
Emerging research points to neuroinflammation as a key factor in PD progression. Microglia, the resident immune cells in the brain, are the main effector cells involved in neuroinflammation.6–8 In early PD, activated microglia amass at injury sites to mediate the inflammatory response and clear damaged cells or harmful substances, thus providing neuroprotection. However, as the disease advances, these activated microglia release excessive pro-inflammatory mediators such as interleukin (IL)-6 and tumor necrosis factor (TNF)-α, which ultimately damage neurons and exacerbate PD progression.9–11 Therefore, inhibition of microglial over-activation is important for PD treatment, especially in patients with mid to late-stage PD.
Gut microbiota, a community of bacteria that resides in the human intestine, has a close relationship with human health. Recent discoveries indicate that gut microbes may regulate the nervous system of the host through the gut-brain axis and that imbalances in these microbes are strong associated with numerous inflammation-related diseases.12–14 Clinical studies have shown that the composition of the gut flora differs in patients with PD compared to healthy individuals.15–17 1-methyl-4-phenyl-1,2,3,6-tetrahydropyridine (MPTP) is a dopaminergic neurotoxin that induces neurochemical, behavioral, and histopathological alterations in nonhuman primates similar to those seen in patients with PD. The study shows that the intestinal flora of mice injected with MPTP differs from that of control mice.12,18 Furthermore, one study reported that flora transplantation modifies the composition of intestinal flora, improving motor impairment and neuroinflammation in mice.19 Another study showed that Lactobacillus plantarum DP189 reduced MPTP-induced α-synuclein accumulation in mice by modulating oxidative damage, inflammation, and intestinal flora imbalances.20 Thus, neuroinflammation, gut microbial composition, and their interplay might play a role in PD development. Therefore, targeting neuroinflammation and gut microbiota might lead to the development of new neuroprotective drugs and provide a therapeutic approach for PD.
Current PD treatments primarily involve pharmacological control of the symptoms combined with exercise rehabilitation. However, the long-term use of existing drugs is associated with substantial side effects.21–23 Therefore, to identify new treatment candidates, the pathogenesis of PD should be further investigated. Recent studies indicate that many food components or extracts may inhibit the progression of neurodegenerative diseases.24–26 Neohesperidin (Neo), a natural flavonoid extracted from citrus fruits, exhibits potent bioactivity such as cough and phlegm relief, blood lipid reduction, and antioxidant, anti-aging, anti-cancer, anti-allergy, and anti-atherosclerosis properties, etc.27–29 It is also an important raw material for the synthesis of dihydrochalcone sweetener and has extensive uses in the food, chemical, and pharmaceutical industries. Neo protected against methotrexate-induced nephrotoxicity by inhibiting oxidative stress and inflammation in male rats.30 It also attenuated the pathological damage and immune imbalance in rats with myocardial ischemia-reperfusion injury by inactivating Jun N-terminal kinase (JNK) and nuclear factor kappa B (NF-κB) p65 signaling pathways.31 Additionally, Neo reduced high-fat diet-induced obesity in rats and exerted a beneficial role in inflammation-related diseases by altering the composition of the gut microbiota.32 However, whether Neo can positively impact PD by modulating neuroinflammation and gut microbial composition is still unclear. Herein, we aimed to clarify the effect of Neo on neuroinflammation and gut microbial composition in MPTP-injected mice, hoping to identify a potentially effective drug for the treatment of PD.
Materials and methods
Reagents
MPTP (purity ≥ 98%) and dimethyl sulfoxide (DMSO) were obtained from Sigma-Aldrich (Louis, MO). Neo (purity ≥ 98%) was purchased from Chengdu Ruifensi Biotechnology (Chengdu, China). NP-40, antibiotics (vancomycin, neomycin, metronidazole, and penicillin) and PMSF solution were obtained from (Beyotime Biotech, Beijing, China).
Animals and treatment
Male mice (C57BL/6j, ten-week-old) were purchased from Liaoning Chang Sheng (Liaoning, China). Animals were allowed to drink and eat freely in a 12 h light 12 h dark environment. All animal experiments complied with Jilin University's Animal Care and Use Committee regulations (approval number: SY202302005).
In the first experiments, thirty mice were randomly divided into 5 groups (n = 6): saline group (saline, orally), Neo group (Neo 50 mg kg−1 orally), MPTP group (MPTP 25 mg kg−1, dissolved in saline, intraperitoneally), N25+M group (MPTP 25 mg kg−1 intraperitoneally+Neo 25 mg kg−1 orally), and N50+M group (MPTP 25 mg kg−1 intraperitoneally+Neo 50 mg kg−1 orally). Neo was dissolved in saline and administered by gavage once daily for 24 days. On the fourth day, MPTP was injected intraperitoneally once daily for 7 d. After 24 days of Neo treatment, all mice underwent a week of daily acclimation and behavioral training in the test environment, before proceeding with further experiments.
In the second batch of experiments, thirty six mice were randomly divided into donor and recipient mice. The three groups of donor mice (saline, MPTP, N+M) were treated as above. Three groups of recipient mice (FMT-saline, FMT-MPTP, FMT-N+M) were transplanted daily with feces from the corresponding recipient group for 4 weeks and injected MPTP (25 mg kg−1, intraperitoneally) at second week. After that, all recipient mice were executed for further experiments.
Behavioral testing
Once behavioral training concluded, mice underwent behavioral tests (open field, pole climbing, and rotarod experiments) to assess their motor performance (Fig. 1A).
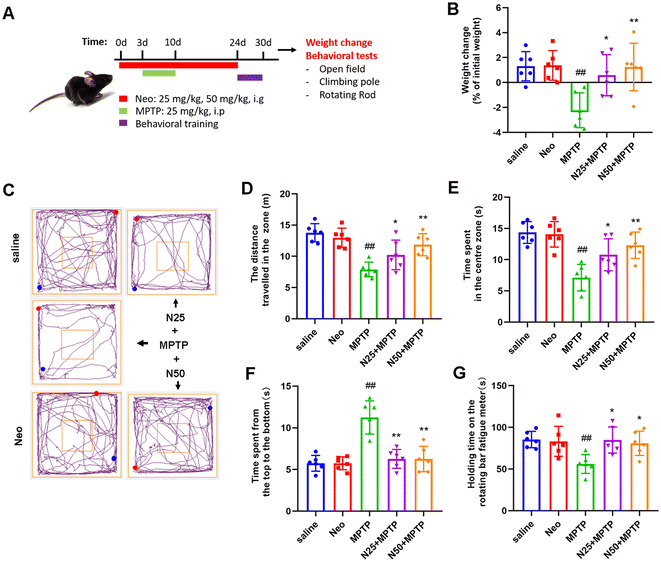 |
| Fig. 1 Impact of neohesperidin (Neo) on 1-methyl-4-phenyl-1,2,3,6-tetrahydropyridine (MPTP)-induced weight loss and locomotor behavior in mice. (A) Schematic of the experimental design (B) Mouse body weight variations (C) Travel patterns of mice in the open field (D) Total distance traversed by mice within the zone for 5 min. (E) Duration spent by mice in the central zone for 5 min. (F) Time taken by mice to descend from the top to the bottom of the pole. (G) Duration mice were able to remain on the rotarod. Data are presented as mean ± SEM (n = 6). ##P < 0.01 vs. saline group. *P < 0.05, **P < 0.01 vs. MPTP-treated group. | |
Open field test.
It was used to assess the motor activity and exploratory behavior of mice. The animals were free to move within a 50 cm × 50 cm × 30 cm open field. A computerized tracking system recorded their movements over five minutes, with total travel distance and time spent in the central area being analyzed using behavioral analysis software (Stoelting Co, US). All mice were allowed to adapt to the environment for 3 d before the test. The open field was cleaned after the test.
Pole climbing test.
This test evaluated the balance and limb coordination of mice. They were placed atop a 50 cm long and 1 cm diameter rod and allowed to climb down freely. The time taken from top to bottom was recorded. Mice were given time to acclimatize to the environment for 3 d before the test.
Rotarod test.
It was used to measure fatigue tolerance. Mice were positioned on a rod rotating (ZS-RDM, Zongshen Technology Co., Ltd, Beijing) at a constant speed of 40 rpm. The length of time they maintained balance without falling was recorded with the Super Maze software system. Mice were allowed to acclimatize to the environment for 3 d before the test. The apparatus was cleaned up after the test.
Immunohistochemical (IHC) assay
After testing, mice were euthanized, and midbrain tissues were collected to assess nerve damage (Fig. 2A). Neuron damage and microglia activation in the substantia nigra (SN) were assessed using IHC. The midbrain tissue was fixed in 4% paraformaldehyde for 24 h, dehydrated using gradient alcohol (70%, 80%, 90%, 95%, and 100% alcohol for one hour each), and cleared in xylene (40 min). The tissue was embedded in paraffin and cut into 5 μm slices. Dopaminergic neurons in the SN were labeled with rabbit anti-tyrosine hydroxylase (TH) antibodies (Abcam, ab137869, Cambridge, UK), and microglia with rabbit anti-ionized calcium binding adapter molecule 1 (IBA-1) antibodies (Abcam, ab178846, Cambridge, UK), using the Ultrasensitive TMS-P kit (Boster Biological Technology Co., Ltd Wuhan, China) according to the manufacturer's instructions. Finally, the number of TH and IBA-1 positive cells was quantified using Image J software.
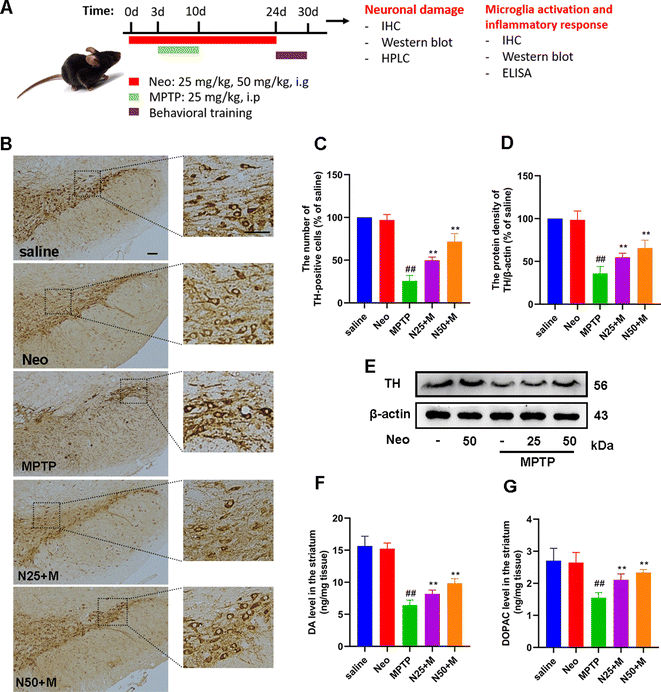 |
| Fig. 2 Effect of Neo administration on MPTP-induced neuronal damage in mice. (A) Schematic of the experimental design (B and C) Tyrosine hydroxylase (TH)-positive cells in the substantia nigra (SN) were measured using immunohistochemistry (IHC) (scale bar = 50 μm) (D and E) The expression of TH at protein level measured in mouse midbrain using western blot (F and G) The content of dopamine (DA) and its metabolite 3,4-dihydroxyphenylacetic acid (DOPAC) in mouse striatum assessed using high-performance liquid chromatography (HPLC). Results are presented as mean ± SEM (n = 3). ##P < 0.01 vs. saline group. **P < 0.01 vs. MPTP-treated group. | |
Western blot
Protein levels and inflammatory pathways in tissues were analyzed via western blot. First, tissues were lysed using NP-40 reagent, and total protein concentration was measured using a bicinchoninic acid protein assay kit according to the manufacturer's instructions (Beyotime Biotech, Beijing, China). Afterward, 40 μg of protein underwent electrophoresis at 100 V for 2 h. After completion of electrophoresis, proteins were transferred to polyvinylidene fluoride membranes (PVDF) (Millipore, Billerica, MA, USA) at 60 V for 2 h. Subsequently, the protein-containing PVDF membrane was closed using 5% skim milk powder for 3 h. Upon completion of the closure, the membranes were incubated with primary antibodies against β-actin (1
:
10
000), OX42 (1
:
500), TH (1
:
1000), NF-κB p65 (1
:
2000), p-NF-κB p65 (1
:
1000), ERK (1
:
2000), p-ERK (1
:
1000), JNK (1
:
2000), p-JNK (1
:
1000), p38 (1
:
2000), p-p38 (1
:
1000), followed by binding to secondary antibodies sheep anti-rabbit (1
:
5000). All antibodies were purchased from Proteintech (Wuhan, China). Finally, we detected the protein using ECL western blotting Detection Reagents (Millipore, MA, US). The results were analyzed using Image J software.
High-performance liquid chromatography (HPLC) assay
HPLC was used to measure the content of DA and its metabolite 3,4-dihydroxyphenylacetic acid (DOPAC) in mouse striatum. Briefly, 0.2 g striatum was dissolved in 1 mL perchloric acid 0.1 M and centrifuged at 12
000 rpm for 10 min, and the supernatant was collected. An equal volume of potassium dihydrogen phosphate solution (300 mM) was added to the supernatant. The mix was centrifuged again at 12
000 rpm for 10 min. The supernatant was collected and filtered through a 0.22 μm filter. A 20 μL sample of this solution was loaded into an HPLC system for analysis.
Enzyme-linked immunosorbent assay (ELISA)
Brain and colon tissues were ground using PMSF-containing PBS at a ratio of 1 mLPBS per 200 mg of tissue. The levels of pro-inflammatory factors (IL-6, TNF-α, and IL-1β) in the brain, colon, and serum and the levels of LPS in the serum were detected using commercially available ELISA kits (Biolegend, San Diego, CA) according to the manufacturer's instructions.
Gut microbiota analysis
Mice feces were collected for gut microbial composition analysis using 16S rRNA sequencing (Shanghai Personal Biotechnology, China). DNA was extracted from fecal samples, isolated, quality-checked, and further used for high-throughput sequencing. The resulting sequences were filtered and trimmed to obtain high-quality sequences. They were clustered into operational taxonomic units (OTUs) based on a similarity threshold set at 97%. Following cluster analysis, OTUs were annotated to obtain species-specific taxa, which facilitated further analysis such as OTU phylogenetic diversity studies. The alpha (α) diversity of gut microbiota was evaluated using the Chao, Faith, and Observed species indexes. The beta (β) diversity was evaluated using the principal coordinate analysis (PCoA) and non-metric multidimensional scaling (NMDS). Furthermore, differences in bacterial species abundance between groups were analyzed using the linear discriminant analysis (LDA) effect size (LEfSe) algorithm.
Hematoxylin–eosin (H&E) staining
Colonic tissue damage was assessed using H&E staining. Fresh colonic tissue samples were fixed in 4% paraformaldehyde for 24 h, dehydrated through a gradient alcohol series (70%, 80%, 90%, 95%, and 100% alcohol for one hour each), and cleared with xylene for 40 min, before paraffin embedding. Embedded tissue was sectioned into 5 μm slices using a microtome. Subsequently, these sections were deparaffinized in xylene (for 30 min), rehydrated through a reverse alcohol gradient (100%, 95%, 90%, 80%, and 70% alcohol for 5 min each), and stained sequentially with hematoxylin (for 3 min) and eosin (for 3 min). After staining, sections were dehydrated, cleared, mounted, and finally visualized under a microscope.
Fecal microbiota transplantation (FMT)
Recipient mice were treated with antibiotics by gavage daily for 7 days prior to performing fecal microbiota transplants. Antibiotics included vancomycin (100 mg kg−1), neomycin (200 mg kg−1), metronidazole (200 mg kg−1), and penicillin (200 mg kg−1). During fecal transplantation, 200 mg of feces from donor rats was dissolved in sterile water. After sufficient shaking and centrifugation, the supernatant was gavaged to recipient rats approximately 200 μL each. Feces of donor mice were obtained freshly every day. The fecal microbiota transplantation procedure was performed in the evening of each day for 4 weeks.
Statistical analysis
Data were presented as mean ± standard error of the mean (SEM). Statistical analysis was performed, and figures were generated using Graph Pad Prism 8. Shapiro–Wilk test was used to test the normality of data distribution. Brown-Forsythe test for homoscedasticity of variance was used. The differences among multiple groups were analyzed using one-way analysis of variance (ANOVA) combined with multiple comparisons and followed by Tukey's post hoc test. A p-value < 0.05 was considered statistically significant.
Results
Neo prevents MPTP-induced weight loss and behavioral deficits in mice
In patients, PD primarily presents as mental depression and loss of motor skills. To investigate the potential therapeutic effect of Neo on PD, we induced PD symptoms in mice by intraperitoneal injection of MPTP (25 mg kg−1 d−1, 7d), and subsequently administered Neo by gavage (25 mg kg−1, 50 mg kg−1). Initially, the analysis focused on the impact of Neo on weight loss in MPTP-injected mice, revealing that Neo significantly mitigated MPTP-induced body weight loss (Fig. 1B).
Subsequently, we examined the effects of Neo on the locomotor behavior of MPTP-injected mice using open field, pole climbing, and rotarod tests. In the open-field test, Neo significantly enhanced the locomotor and exploratory abilities of MPTP-injected mice (Fig. 1C–E). The pole climbing test revealed that Neo improved balance and coordination in MPTP-injected mice (Fig. 1F). The rotarod test demonstrated that Neo administration increased fatigue tolerance in MPTP-injected mice (Fig. 1G). These results demonstrate that Neo alleviates both MPTP injection-induced weight loss and behavioral deficits in mice.
Neo ameliorates MPTP-induced nigrostriatal dopaminergic neuronal damage in mice
The main pathological feature of PD is damage to the nigrostriatal dopaminergic neurons. To explore the effect of Neo on this phenomenon, we used IHC staining methods to assess the number of TH-positive cells in the SN. Compared to the MPTP group, the Neo group presented a significantly higher number of TH-positive cells (Fig. 2B and C). Further supporting this, a western blot assay revealed that Neo administration significantly increased the expression of TH at the protein level in the midbrain of mice compared to MPTP alone (Fig. 2D and E). The health of midbrain dopaminergic neurons is pivotal as their damage affects the levels of striatal DA and its metabolites, leading to motor impairment. Therefore, we evaluated the content of DA and its metabolite DOPAC in mouse striatum using HPLC. The results showed that compared to the MPTP group, Neo group presented higher levels of striatal DA and DOPAC (Fig. 2F and G).
These results demonstrate that Neo mitigates the damage of nigrostriatal dopaminergic neurons induced by MPTP in mice.
Neo attenuates the MPTP-induced hyper-activation of nigrostriatal microglia in mice
In PD, microglial hyper-activation contributes to the inflammatory response and neuronal damage. To explore the effect of Neo on this phenomenon, we examined the number of IBA-1-positive cells in the SN using IHC. MPTP group presented a significantly higher number of IBA-1-positive cells compared to the control group, while Neo administration mitigated this effect (Fig. 3A and B). Subsequently, we used western blot to assess the expression of OX42 in the midbrain of mice. The results indicated that Neo also reduced the expression of OX42 at the protein level in the midbrain of MPTP-injected mice (Fig. 3C and D). Subsequently, we examined the expression of pro-inflammatory mediators (IL-6, IL-1β, and TNF-α) in the mouse midbrain using an ELISA assay. Neo treatment reduced the level of expression of pro-inflammatory mediators in the midbrain of MPTP-injected mice (Fig. 3E–G).
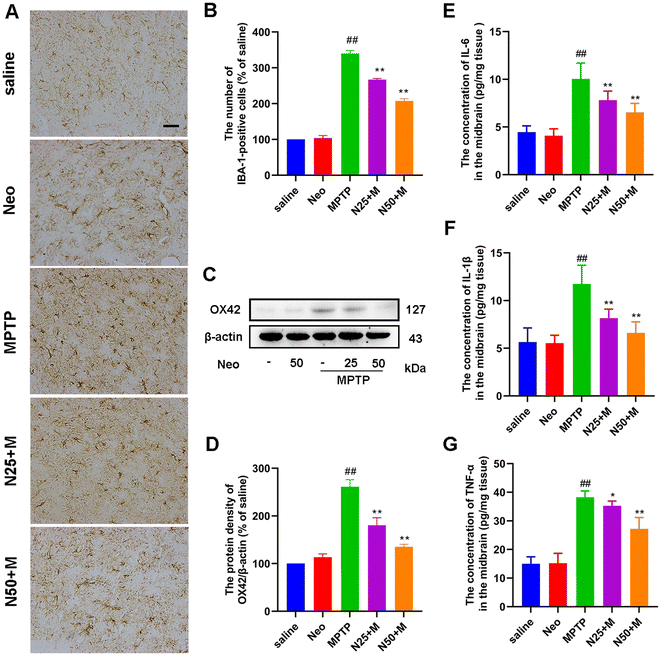 |
| Fig. 3 Effect of Neo administration on MPTP-induced microglial activation in mice. (A and B) The ionized calcium-binding adapter molecule 1 (IBA-1)-positive cells in the substantia nigra measured using immunohistochemistry (scale bar = 20 μm) (C and D) Expression of OX42 at protein level in mouse midbrain measured using western blot (E-G) The expression of pro-inflammatory factors IL-6, IL-1β, TNF-α in the mouse midbrain measured using ELISA. Results are given as mean ± SEM (n = 3). ##P < 0.01 vs. saline group. **P < 0.01 vs. MPTP-treated group. | |
These results demonstrate that Neo reduces the hyper-activation of microglia and neuroinflammation in MPTP-injected mice.
Neo alters gut microbiota composition in MPTP-treated mice
Numerous studies have established an important association between gut microbial composition and neuroinflammation, as well as inflammation-related neurodegenerative diseases. To gain further insight into the effect of Neo on this relationship, we analyzed the gut microbiota composition of mice using 16S rRNA sequencing. As the number of samples increased, the rarefaction curve representing operational taxonomic unit (OUT) levels gradually reached saturation, indicating that the sequencing depth was sufficient (Fig. 4A and B). Additionally, the Venn diagram revealed 4042, 4749, and 5764 independent OTUs detected in the saline, MPTP, and NM (Neo+MPTP) groups, respectively (Fig. 4C).
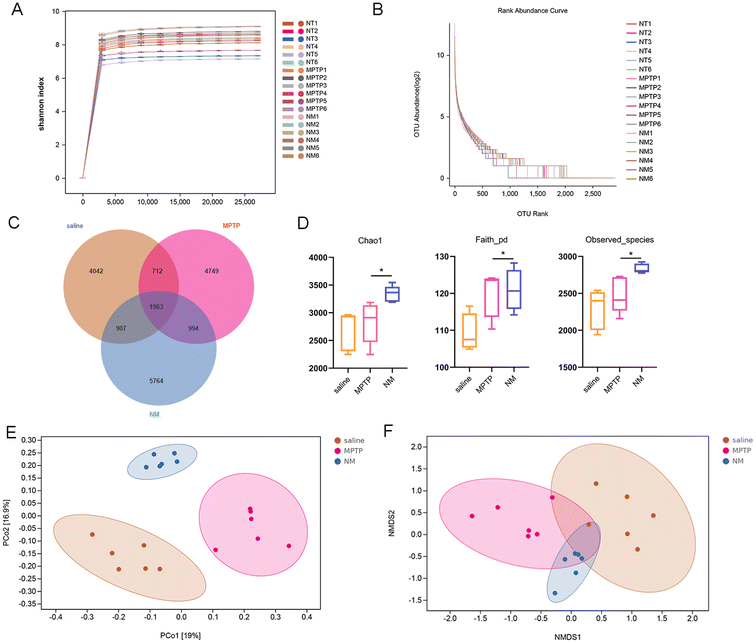 |
| Fig. 4 Effect of Neo on the diversity of mouse intestinal flora. (A and B) Rarefaction curve based on operational taxonomic unit (OTU) levels of intestinal flora (C) The Venn diagram representing OTUs across groups (D) α diversity evaluation – Chao1, Faith, and the observed species indexes (E and F) β diversity evaluation using principal coordinates analysis and nonmetric multidimensional scaling. Results are presented as mean ± SEM (n = 6). *P < 0.05. | |
Subsequent α diversity analysis showed an increase in the Chao1, Faith, and observed species indexes in the Neo group compared to the MPTP group, suggesting that Neo treatment augmented the α diversity of the gut microbiota in mice (Fig. 4D). Additionally, β diversity analysis revealed that compared to the MPTP group, the gut microbial composition of the NM group was more similar to that of the control group (Fig. 4E and F). These results demonstrate that Neo alters the gut microbiota composition in MPTP-injected mice.
Neo attenuates the MPTP-induced imbalance in the gut microbiota of mice
To further delineate the effect of Neo on gut microbial composition, we used LEfSe to identify statistically significant biomarkers across different treatment groups. Compared to the saline group, the MPTP group presented higher levels of Allobaculum, Psychrobacter, Corynebacterium, while the Neo+MPTP group presented higher levels of Prevotella and Bacteroides (Fig. 5A and B).
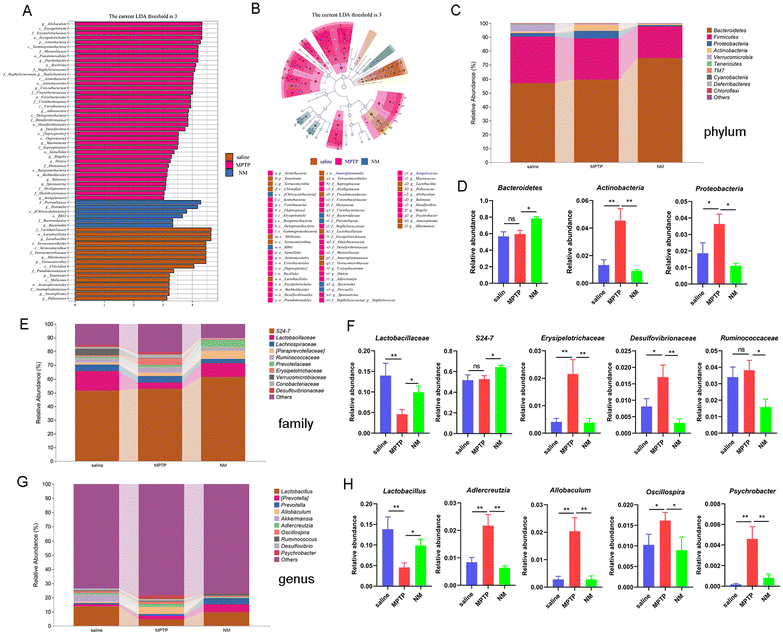 |
| Fig. 5 Impact of Neo on gut microbial composition in MPTP-injected mice. (A and B) Statistically significant biomarkers across different treatment groups revealed by linear discriminant analysis effect size analysis (linear discriminant analysis cut-off score > 3, P < 0.05) (C and D) Relative abundance differences of gut microbes at the phylum level (E and F) Relative abundance differences of gut microbes at the family level (G and H) Relative abundance differences of gut microbes at the genus level. Results are presented as mean ± SEM (n = 6). *P < 0.05, **P < 0.01. | |
Next, we analyzed the effect of Neo on the abundance of gut microbiota species at the phylum, family, and genus levels (Fig. 5C, E and G). At the phylum level, Neo treatment significantly increased the abundance of Bacteroidetes and decreased the abundance of Actinobacteria and Proteobacteria (Fig. 5C and D) in MPTP-injected mice. At the family level, Neo treatment significantly increased the abundance of Lactobacillaceae and S24-7 while decreasing that of Erysipelotrichaceae, Desulfovibrionaceae, and Ruminococcaceae (Fig. 5E and F) in MPTP-injected mice. At the genus level, Neo treatment significantly increased the abundance of Lactobacillus and decreased that of Adlercreutzia, Allobaculum, Oscillospira, and Psychrobacter (Fig. 5G and H) in MPTP-injected mice.
These results demonstrate that Neo mitigates MPTP-induced gut microbiota imbalance in mice.
Neo mitigates MPTP-induced colonic damage and inflammatory responses in mice
Several studies have demonstrated a correlation between gut flora imbalance and colonic injury. We further investigated the effects of Neo on colonic tissue damage. Our results revealed that Neo administration increases the integrity of colonic tissue and reduces inflammatory cell infiltration in MPTP-injected mice (Fig. 6B). Subsequently, we examined the expression of barrier proteins (ZO-1, claudin-3) in colonic tissue. Neo administration elevated the expression of ZO-1 and claudin-3 compared to MPTP alone (Fig. 6C–E), indicating Neo inhibited MPTP-induced colonic tissue damage in mice.
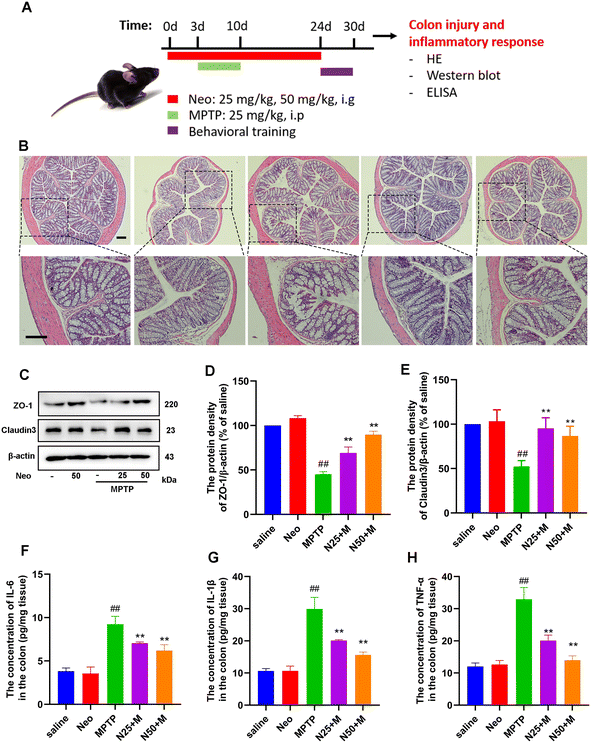 |
| Fig. 6 Impact of Neo on MPTP-induced colonic tissue damage and inflammatory response in mice. (A) Schematic of the experimental design (B) Colonic damage detected using hematoxylin–eosin staining (scale bar = 250 μm) (C–E) The level of expression of barrier proteins ZO-1 and claudin-3 was detected using western blot (F–H) The expression of pro-inflammatory IL-6, IL-1β, and factor of TNF-α in the mouse colonic tissue measured using ELISA assay. Results are presented as mean ± SEM (n = 6). ##P < 0.01 vs. saline group. **P < 0.01 vs. MPTP-treated group. | |
Additionally, we quantified pro-inflammatory mediators in colonic tissues. Neo administration significantly reduced the expression of IL-6, IL-1β, and TNF-α in colonic tissues (Fig. 6F–H).
These results demonstrate that Neo mitigates colonic damage and inhibits inflammatory responses in MPTP-injected mice.
Neo attenuates MPTP-induced activation of NF-κB and mitogen-activated protein kinase (MAPK) pathways in mice
The expression of inflammatory mediators is regulated by specific inflammatory pathways. We examined the effect of Neo on key inflammatory pathways (NF-κB and MAPK) in the midbrain and colonic tissue of MPTP-injected mice. We initially observed significantly higher phosphorylation levels of NF-κB p65 (Fig. 7A and B), ERK (Fig. 7A and C), JNK (Fig. 7A and D), and p38 (Fig. 7A and E) in the midbrain of MPTP-injected mice compared to saline-treated mice. This effect was inhibited by Neo. Similar results were obtained for the colonic tissue (Fig. 7F–J). These results demonstrate that Neo reduces MPTP-induced activation of the NF-κB and MAPK signaling pathways in mice.
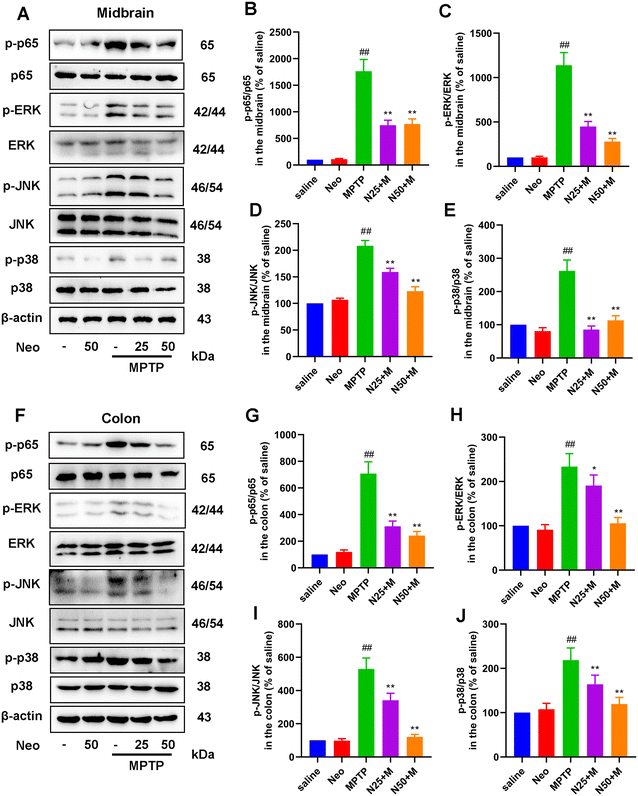 |
| Fig. 7 Impact of Neo administration on the nuclear factor (NF)-κB and mitogen-activated protein kinase (MAPK) pathways in MPTP-injected mice. (A) The protein levels of phosphate (p)-Jun N-terminal kinase (JNK), JNK, p-p38, p38, p-p65, p65, p-extracellular signal-regulated kinase (ERK), and ERK in the mouse midbrain measured by western blot (B–E) Gray-scale analysis results of phosphorylated protein relative to total protein content (F) The protein levels of p-JNK, JNK, p-p38, p38, p-p65, p65, p-ERK, and ERK in the mouse colonic tissues measured by western blot (G–J) Gray-scale analysis results of phosphorylated protein relative to total protein content. Results are presented as mean ± SEM (n = 6). ##P < 0.01 vs. saline group. *P < 0.05, **P < 0.01 vs. MPTP-treated group. | |
Lipopolysaccharide (LPS) and inflammatory cytokines mediate the association between PD-like nerve injury, colitis, and gut microbiota
To investigate the potential association between neuroinflammation and colonic inflammation, we evaluated the serum levels of LPS and pro-inflammatory mediators. Our results revealed that serum levels of LPS and pro-inflammatory cytokines (IL-6, IL-1β, and TNF-α) were significantly higher in the MPTP group than in the saline group, while Neo treatment reduced the MPTP-induced serum elevation of LPS (Fig. 8A) in mice (Fig. 8B–D).
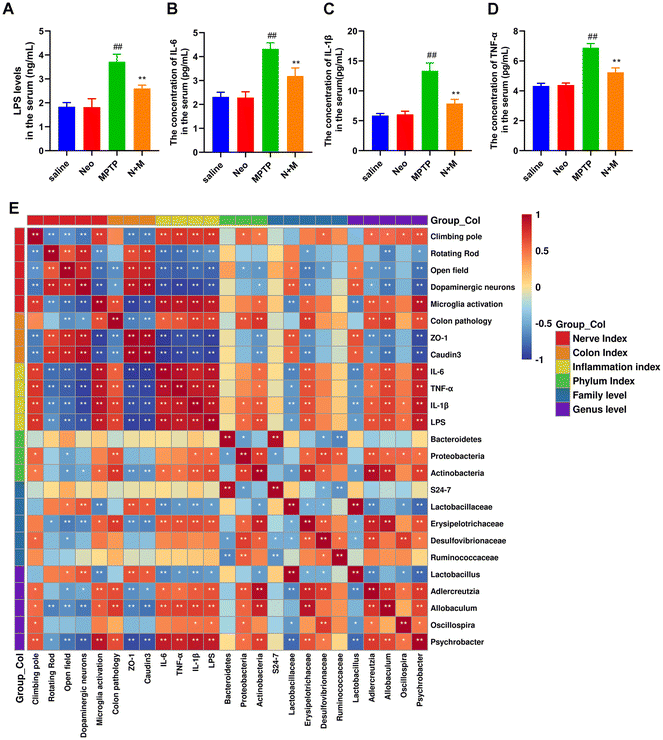 |
| Fig. 8 Lipopolysaccharide (LPS) and inflammatory cytokines mediate the association between PD-like neural damage, colonic injury, and gut microbiota. (A) Serum levels of LPS measured using ELISA assay (B–D) Serum levels of pro-inflammatory cytokines IL-6, IL-1β, and TNF-α measured using ELISA (E) The Spearman's rank correlation between LPS, inflammatory cytokines, PD-like neural damage, colonic injury, and gut microbiota. Results are presented as mean ± SEM (n = 6). ##P < 0.01 vs. saline group. *P < 0.05, **P < 0.01 vs. MPTP-treated group. | |
Additionally, we analyzed the correlation between neural damage, colonic damage, serum levels of pro-inflammatory factors, and gut microbiota using Spearman's rank correlation analysis (Fig. 8E). We found positive correlations among neural damage, colonic injury, and serum levels of LPS and pro-inflammatory factors. At the phylum level, Proteobacteria and Actinobacteria were positively correlated, while Bacteroidetes were negatively correlated with serum inflammation levels. At the family level, Erysipelotrichaceae, Desulfovibrionaceae, and Ruminococcaceae were positively correlated, while S24-7 and Lactobacillaceae were negatively correlated with serum inflammation levels. At the genus level, Adlercreutzia, Allobaculum, Oscillospira, and Psychrobacter were positively correlated, while Lactobacillus were negatively correlated with serum inflammation levels.
In summary, these results suggest that gut microbiota are involved in Neo's mitigation of neural damage and colonic injury and that LPS and pro-inflammatory factors might be crucial in this process.
FMT alleviates motor symptoms and neurodegeneration in MPTP-intoxicated PD mice
To further elucidate the role of gut microbes in Neo exerting neuroprotection, we performed fecal microbial transplantation experiments (Fig. 9A). First we evaluated the effect of FMT on nerve injury and microglia activation in MPTP-injected mice by IHC experiments. The results showed that after MPTP injection, mice transplanted with feces from the MPTP group showed a significant decrease in dopaminergic neurons and an increase in microglia activation compared to mice transplanted with saline. In contrast, mice that inhibited feces from the N+M group showed reduced neuronal damage and decreased microglia activation (Fig. 9B–D). Similarly, protein assays for neuronal markers and microglia activation markers presented the same results (Fig. 9E–G). These results suggest that FMT alleviated neurodegeneration in MPTP-intoxicated PD mice.
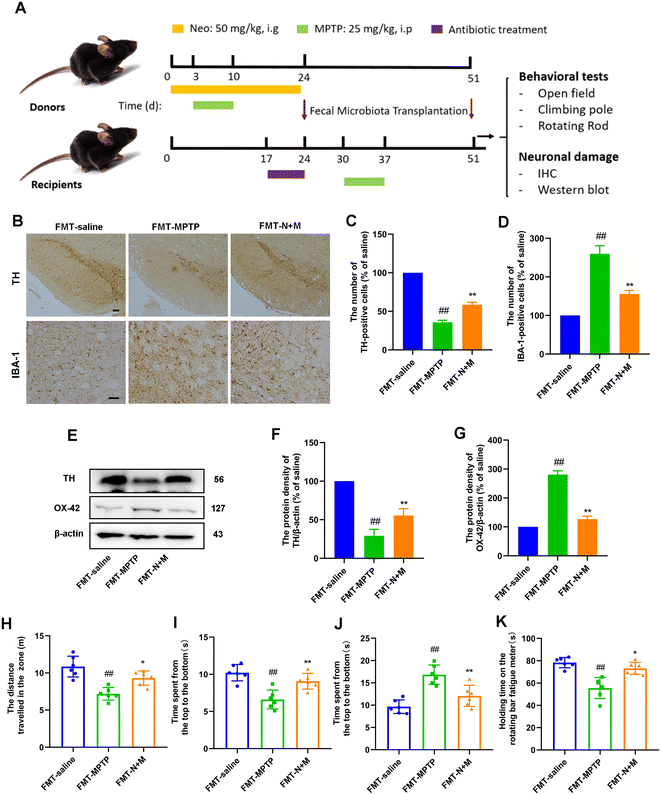 |
| Fig. 9 Effect of FMT on MPTP-induced neuronal damage and motor symptoms in mice. (A) Schematic of the experimental design (B and C) TH-positive cells in the SN were measured using IHC (scale bar = 50 μm). (B and D) IBA-1-positive cells in the SN were measured using IHC (scale bar = 20 μm) (E–G) The expression of TH and OX-42 at protein level measured in mouse midbrain using western blot. Results are presented as mean ± SEM (n = 3). (H) Total distance traversed by mice within the zone for 5 min. (I) Duration spent by mice in the central zone for 5 min. (J) Time taken by mice to descend from the top to the bottom of the pole. (K) Duration mice were able to remain on the rotarod. Data are presented as mean ± SEM (n = 6). #P < 0.05, ##P < 0.01 vs. FMT-saline group. *P < 0.05, **P < 0.01 vs. FMT-MPTP-treated group. | |
Subsequently, we evaluated the effect of FMT on motor symptoms in MPTP-injected mice by behavioral experiments. The results of the open-field experiment showed that mice in the FMT-N+M group exhibited greater locomotor activity and exploratory ability compared to the FMT-MPTP group (Fig. 9H and I). The results of pole-climbing experiments showed that FMT ameliorated the decrease in balance caused by MPTP-injected mice (Fig. 9J). The results of the rod-turning experiment showed that FMT ameliorated the decrease in fatigue tolerance caused by MPTP-injected mice (Fig. 9K). These results suggest that FMT alleviated motor symptoms in MPTP-intoxicated PD mice.
Discussion
PD, the second most prevalent neurodegenerative disease worldwide, is typically characterized by motor dysfunction such as bradykinesia and rigidity.1,33 To investigate the potential therapeutic role of Neo, we developed a mouse model of PD induced by intraperitoneal injection of MPTP. In this study, we evaluated the effects of Neo on MPTP-induced inflammation and neurodegeneration in mice. Our results revealed that Neo not only ameliorated MPTP-induced motor impairment and neuronal damage but also inhibited neuroinflammation and modulated gut microbiota imbalance. Additionally, Neo mitigated MPTP-induced colonic inflammation and tissue damage. Mechanistic studies revealed that Neo suppressed the inflammatory response by inhibiting MPTP-induced excessive activation of the NF-κB and MAPK pathways. These results collectively demonstrate that in MPTP-injected mice, Neo administration attenuates neurodegeneration by suppressing inflammation and regulating the composition of gut microbiota (Fig. 10).
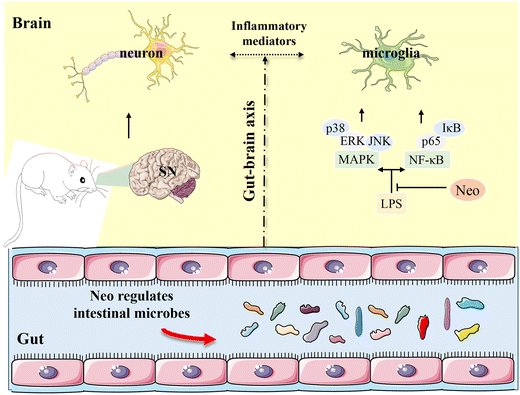 |
| Fig. 10 Neohesperidin attenuates MPTP-induced neurodegeneration by inhibiting inflammatory responses and regulating intestinal flora in mice. | |
The main pathological change in PD is the degeneration of nigrostriatal dopaminergic neurons, resulting in reduced striatal DA levels.4,34 Therefore, we investigated the effects of Neo on MPTP-induced damage of the nigrostriatal dopaminergic neurons and the striatal DA metabolism. Neo administration significantly mitigated the damage of nigrostriatal dopaminergic neurons and increased the levels of striatal DA and its metabolite DOPAC in MPTP-injected mice. These results demonstrate Neo ameliorates PD symptoms in MPTP-injected mice, revealing its possible beneficial role in PD treatment.
Although the exact etiology of PD remains unclear, inflammation has been involved in its pathogenesis. Pro-inflammatory mediators released by over-activated microglia induce neuronal damage and exacerbate the progression of PD.7,9,35 Neo has been reported to inhibit inflammation in peripheral tissues. Herein, we demonstrated that Neo administration significantly inhibited the hyper-activation of microglia and the release of pro-inflammatory mediators in MPTP-injected mice, suggesting that Neo may exert neuroprotective effects by suppressing neuroinflammation. However, the exact underlying mechanism remains to be clarified.
Gut microbes regulate the nervous system of the host via the gut-brain axis. Imbalances in the gut flora are associated with several inflammation-related diseases. Furthermore, an imbalance in intestinal flora is also an important trigger of neuroinflammation, and regulation of gut microbial composition mitigates neuroinflammation.12,36,37 In our experiments, we first found that administration of Neo ameliorated PD-associated symptoms such as MPTP-induced dyskinesia and neurological damage in mice. Further studies, we also found that Neo administration inhibited neuroinflammation in MPTP-injected mice. To further explore the role of neohesperidin, we assessed the gut microbial composition of mice by 16S sequencing. The results showed that Neo administration was able to modulate the intestinal microbial imbalance in MPTP-injected mice, as evidenced by an increase in the abundance of beneficial bacteria and a decrease in the abundance of harmful bacteria. Studies have shown that some harmful bacteria may cause inflammation in the body by releasing harmful factors such as LPS that flow through the circulatory system.38,39 In this experiment, we examined serum levels of LPS and pro-inflammatory mediators. It was found that the levels of LPS and pro-inflammatory mediators in the serum of mice in the Neo-administered group were significantly lower compared with those in the MPTP group, which further suggests that Neo administration may regulate neuroinflammation by modulating the microbiota composition of the intestinal tract. In this study, we demonstrated that Neo administration ameliorated colonic tissue damage and reduced the levels of expression of pro-inflammatory mediators in the colon of MPTP-injected mice. These results indicate that Neo has beneficial effects in MPTP-injected mice by modulating intestinal microbial composition and preventing colonic tissue damage. Also, our results lay the foundation for targeting gut microbes to further investigate the role of Neo in neurodegenerative diseases.
NF-κB is a critical regulator of cellular responses, with its activation often triggered by various bacterial metabolites and surface receptors. This activation regulates the transcription of pro-inflammatory mediators, such as IL-6, TNF-α, and IL-1β.40,41 The MAPK pathway, another important cellular signal transmitter, has been associated with inflammation upon activation of its subunits, including ERK, JNK, and p38.42,43 Studies have shown that neuroinflammation plays an important role in the pathogenesis of PD. In this experiment, we found that Neo inhibited neuroinflammation in MPTP-injected mice. And further studies have found that gut microbes play a role in this. It has also been reported that gut microbes may regulate inflammatory responses by releasing factors such as LPS that modulate the activation of NF-κB and MAPK pathways.44,45 It has been shown that LPS mediates inflammatory responses by activating NF-κB and MAPK pathways through TLR4 receptors on the cell surface.46,47 To clarify the mechanism by which Neo acts, we explored the effects of Neo on NF-κB and MAPK pathways in MPTP-injected mice. The results showed that Neo administration improved the activation of NF-κB and MAPK pathways in MPTP-injected mice.
LPS, the main component of the cell wall of Gram-negative bacteria, often induces NF-κB and MAPK pathway activation.48,49 In this study, we found that Neo administration significantly reduced the serum levels of LPS and pro-inflammatory mediators in MPTP-injected mice. Additionally, Spearman's rank correlation analysis revealed that the levels of LPS and pro-inflammatory mediators were positively associated with neuronal damage and colonic injury and correlated with gut microbial abundance at different levels. These results suggest that LPS and pro-inflammatory mediators may mediate the association between gut microbiota, inflammation, and nerve injury. To further demonstrate the role played by gut microorganisms in the amelioration of PD symptoms in mice by Neo, we performed a flora transplantation experiment. We found that behavioral and neurological impairments were significantly improved in mice with Neo+MPTP group feces compared to mice with MPTP group feces. However, mice with Neo+MPTP group feces still had motor behavioral deficits and neurological impairments compared to mice with control group feces by gavage. These results suggest that gut microbes play a role in Neo ameliorating dyskinesia and neurological damage in MPTP-injected mice, but Neo does not only act through this one pathway.
In summary, this study provides the first evidence that Neo attenuates MPTP-induced neurodegeneration in mice and exerts neuroprotective effects by inhibiting the excessive activation of NF-κB and MAPK signaling pathways. These effects were associated with the ability of Neo to increase intestinal flora diversity and significantly alter its composition, further lead to an increase in the abundance of beneficial bacteria and a decrease in that of harmful bacteria. Combined with the fact that it comes from fruit and has properties such as non-toxicity and easy access, Neo may offer therapeutic benefits in PD by targeting neuroinflammation and the composition of gut microbiota. However, its specific role and its application in PD need to be further investigated.
Abbreviations
DA | Dopamine |
DOPAC | 3,4-dihydroxyphenylacetic acid |
FMT | Fecal microbiota transplantation |
IBA-1 | Ionized calcium-binding adapter molecule 1; |
IHC | Immunohistochemical |
IL-1β | Interleukin IL-1β |
IL-6 | Interleukin IL-6 |
MAPK | Mitogen-activated protein kinase |
MPTP | 1-Methyl-4-phenyl-1,2,3,6-tetrahydropyridine |
Neo | Neohesperidine |
NF-κB | Nuclear factor (NF)-κB |
PD | Parkinson's disease |
SN | Substantia nigra |
TH | tyrosine hydroxylase |
TNF-α | Tumor necrosis factor-α. |
Author contributions
Dewei He and Xiyu Gao accomplished most of the experiments, analyzed the results and wrote the manuscript. Juxiong Liu and Dianfeng Liu designed this study. Jingru Wen, Yiming Zhang, Shuo Yang, Xiaojia Sun, Mingchi Cui, Zhe Li, Shoupeng Fu took part in the study. All authors read and approved the final manuscript.
Conflicts of interest
The authors declare no competing financial interest.
Acknowledgements
This work was funded by Natural Science Foundation of Chongqing (Project No. CSTB2022NSCQ-MSX0330), Jilin Scientific and Technological Development Program (Project No.20230505038ZP), and Graduate Innovation Fund of Jilin University (Project No. 202310183330, S202310183663).
References
- L. Klingelhoefer and H. Reichmann, Parkinson's disease as a multisystem disorder, J. Neural Transm., 2017, 124, 709–713 CrossRef CAS PubMed.
- A. Samii, J. G. Nutt and B. R. Ransom, Parkinson's disease, Lancet, 2004, 363, 1783–1793 CrossRef CAS PubMed.
- J. Pagonabarraga, J. Kulisevsky, A. P. Strafella and P. Krack, Apathy in Parkinson's disease: clinical features, neural substrates, diagnosis, and treatment, Lancet Neurol., 2015, 14, 518–531 CrossRef PubMed.
- D. K. Simon, C. M. Tanner and P. Brundin, Parkinson Disease Epidemiology, Pathology, Genetics, and Pathophysiology, Clin. Geriatr. Med., 2020, 36, 1–12 CrossRef PubMed.
- M. Picillo, A. Nicoletti, V. Fetoni, B. Garavaglia, P. Barone and M. T. Pellecchia, The relevance of gender in Parkinson's disease: a review, J. Neurol., 2017, 264, 1583–1607 CrossRef PubMed.
- W. W. Liu, S. Z. Wei, G. D. Huang, L. B. Liu, C. Gu, Y. Shen, X. H. Wang, S. T. Xia, A. M. Xie, L. F. Hu, F. Wang and C. F. Liu, BMAL1 regulation of microglia-mediated neuroinflammation in MPTP-induced Parkinson's disease mouse model, FASEB J., 2020, 34, 6570–6581 CrossRef CAS PubMed.
- A. Heidari, N. Yazdanpanah and N. Rezaei, The role of Toll-like receptors and neuroinflammation in Parkinson's disease, J. Neuroinflammation, 2022, 19, 135 CrossRef CAS PubMed.
- S. Sarkar, H. M. Nguyen, E. Malovic, J. Luo, M. Langley, B. N. Palanisamy, N. Singh, S. Manne, M. Neal, M. Gabrielle, A. Abdalla, P. Anantharam, D. Rokad, N. Panicker, V. Singh, M. Ay, A. Charli, D. Harischandra, L. W. Jin, H. Jin, S. Rangaraju, V. Anantharam, H. Wulff and A. G. Kanthasamy, Kv1.3 modulates neuroinflammation and neurodegeneration in Parkinson's disease, J. Clin. Invest., 2020, 130, 4195–4212 CAS.
- E. Lazdon, N. Stolero and D. Frenkel, Microglia and Parkinson's disease: footprints to pathology, J. Neural Transm., 2020, 127, 149–158 CrossRef PubMed.
- S. George, N. L. Rey, T. Tyson, C. Esquibel, L. Meyerdirk, E. Schulz, S. Pierce, A. R. Burmeister, Z. Madaj, J. A. Steiner, M. L. Escobar Galvis, L. Brundin and P. Brundin, Microglia affect alpha-synuclein cell-to-cell transfer in a mouse model of Parkinson's disease, Mol. Neurodegener., 2019, 14, 34 CrossRef PubMed.
- S. Smajic, C. A. Prada-Medina, Z. Landoulsi, J. Ghelfi, S. Delcambre, C. Dietrich, J. Jarazo, J. Henck, S. Balachandran, S. Pachchek, C. M. Morris, P. Antony, B. Timmermann, S. Sauer, S. L. Pereira, J. C. Schwamborn, P. May, A. Grunewald and M. Spielmann, Single-cell sequencing of human midbrain reveals glial activation and a Parkinson-specific neuronal state, Brain, 2022, 145, 964–978 CrossRef PubMed.
- T. R. Sampson, J. W. Debelius, T. Thron, S. Janssen, G. G. Shastri, Z. E. Ilhan, C. Challis, C. E. Schretter, S. Rocha, V. Gradinaru, M. F. Chesselet, A. Keshavarzian, K. M. Shannon, R. Krajmalnik-Brown, P. Wittung-Stafshede, R. Knight and S. K. Mazmanian, Gut Microbiota Regulate Motor Deficits and Neuroinflammation in a Model of Parkinson's Disease, Cell, 2016, 167, 1469–1480 CrossRef CAS PubMed.
- C. Chen, J. Liao, Y. Xia, X. Liu, R. Jones, J. Haran, B. McCormick, T. R. Sampson, A. Alam and K. Ye, Gut microbiota regulate Alzheimer's disease pathologies and cognitive disorders via PUFA-associated neuroinflammation, Gut, 2022, 71, 2233–2252 CrossRef PubMed.
- J. M. Pickard, M. Y. Zeng, R. Caruso and G. Nunez, Gut microbiota: Role in pathogen colonization, immune responses, and inflammatory disease, Immunol. Rev., 2017, 279, 70–89 CrossRef CAS PubMed.
- M. F. Sun and Y. Q. Shen, Dysbiosis of gut microbiota and microbial metabolites in Parkinson's Disease, Ageing Res. Rev., 2018, 45, 53–61 CrossRef CAS PubMed.
- Q. Wang, Y. Luo, K. Ray Chaudhuri, R. Reynolds, E. K. Tan and S. Pettersson, The role of gut dysbiosis in Parkinson's disease: mechanistic insights and therapeutic options, Brain, 2021, 144, 2571–2593 CrossRef PubMed.
- A. Pant, K. S. Bisht, S. Aggarwal and T. K. Maiti, Human gut microbiota and Parkinson's disease, Prog. Mol. Biol. Transl. Sci., 2022, 192, 281–307 CAS.
- M. F. Sun, Y. L. Zhu, Z. L. Zhou, X. B. Jia, Y. D. Xu, Q. Yang, C. Cui and Y. Q. Shen, Neuroprotective effects of fecal microbiota transplantation on MPTP-induced Parkinson's disease mice: Gut microbiota, glial reaction and TLR4/TNF-alpha signaling pathway, Brain, Behav., Immun., 2018, 70, 48–60 CrossRef CAS PubMed.
- Z. Zhao, J. Ning, X. Q. Bao, M. Shang, J. Ma, G. Li and D. Zhang, Fecal microbiota transplantation protects rotenone-induced Parkinson's disease mice via suppressing inflammation mediated by the lipopolysaccharide-TLR4 signaling pathway through the microbiota-gut-brain axis, Microbiome, 2021, 9, 226 CrossRef CAS PubMed.
- L. Wang, Z. Zhao, L. Zhao, Y. Zhao, G. Yang, C. Wang, L. Gao, C. Niu and S. Li, Lactobacillus plantarum DP189 Reduces alpha-SYN Aggravation in MPTP-Induced Parkinson's Disease Mice via Regulating Oxidative Damage, Inflammation, and Gut Microbiota Disorder, J. Agric. Food Chem., 2022, 70, 1163–1173 CrossRef CAS PubMed.
- M. J. Armstrong and M. S. Okun, Diagnosis and Treatment of Parkinson Disease: A Review, J. Am. Med. Assoc., 2020, 323, 548–560 CrossRef PubMed.
- M. J. Armstrong and M. S. Okun, Choosing a Parkinson Disease Treatment, J. Am. Med. Assoc., 2020, 323, 1420 CrossRef PubMed.
- M. K. Mak, I. S. Wong-Yu, X. Shen and C. L. Chung, Long-term effects of exercise and physical therapy in people with Parkinson disease, Nat. Rev. Neurol., 2017, 13, 689–703 CrossRef PubMed.
- A. Kozlowska and D. Szostak-Wegierek, Flavonoids–food sources and health benefits, Rocz. Panstw. Zakl. Hig., 2014, 65, 79–85 Search PubMed.
- A. Nakajima and Y. Ohizumi, Potential Benefits of Nobiletin, A Citrus Flavonoid, against Alzheimer’s Disease and Parkinson’s Disease, Int. J. Mol. Sci., 2019, 20, 3380 CrossRef CAS PubMed.
- Q. Liu, Y. Xi, Q. Wang, J. Liu, P. Li, X. Meng, K. Liu, W. Chen, X. Liu and Z. Liu, Mannan oligosaccharide attenuates cognitive and behavioral disorders in the 5xFAD Alzheimer's disease mouse model via regulating the gut microbiota-brain axis, Brain, Behav., Immun., 2021, 95, 330–343 CrossRef CAS PubMed.
- Y. Xiao, D. Su, X. Hu, G. Yang and Y. Shan, Neohesperidin Dihydrochalcone Ameliorates High-Fat Diet-Induced Glycolipid Metabolism Disorder in Rats, J. Agric. Food Chem., 2022, 70, 9421–9431 CrossRef CAS PubMed.
- S. Yuan, C. Zhang and B. Wang, Neohesperidin promotes the proliferation and osteogenic differentiation of BMSCs via BMP2-Wnt/beta-catenin pathway, Cell Cycle, 2022, 21, 187–201 CrossRef CAS PubMed.
- L. Cheng, Y. Ren, D. Lin, S. Peng, B. Zhong and Z. Ma, The Anti-Inflammatory Properties of Citrus wilsonii Tanaka Extract in LPS-Induced RAW 264.7 and Primary Mouse Bone Marrow-Derived Dendritic Cells, Molecules, 2017, 22, 1213 CrossRef PubMed.
- A. T. Osman, S. M. Z. Sharkawi, M. I. A. Hassan, A. M. Abo-Youssef and R. A. M. Hemeida, Empagli fl ozin and neohesperidin protect against methotrexate-induced renal toxicity via suppression of oxidative stress and inflammation in male rats, Food Chem. Toxicol., 2021, 155, 112406 CrossRef CAS PubMed.
- A. Li, X. Zhang and Q. Luo, Neohesperidin alleviated pathological damage and immunological imbalance in rat myocardial ischemia-reperfusion injury via inactivation of JNK and NF-kappaB p65, Biosci., Biotechnol., Biochem., 2021, 85, 251–261 CrossRef PubMed.
- J. F. Lu, M. Q. Zhu, H. Zhang, H. Liu, B. Xia, Y. L. Wang, X. Shi, L. Peng and J. W. Wu, Neohesperidin attenuates obesity by altering the composition of the gut microbiota in high-fat diet-fed mice, FASEB J., 2020, 34, 12053–12071 CrossRef CAS PubMed.
- J. Opara, A. Malecki, E. Malecka and T. Socha, Motor assessment in Parkinson;s disease, Ann. Agric. Environ. Med., 2017, 24, 411–415 CrossRef PubMed.
- Z. Chen, G. Li and J. Liu, Autonomic dysfunction in Parkinson's disease: Implications for pathophysiology, diagnosis, and treatment, Neurobiol. Dis., 2020, 134, 104700 CrossRef PubMed.
- D. He, S. Fu, B. Ye, H. Wang, Y. He, Z. Li, J. Li, X. Gao and D. Liu, Activation of HCA2 regulates microglial responses to alleviate neurodegeneration in LPS-induced in vivo and in vitro models, J. Neuroinflammation, 2023, 20, 86 CrossRef CAS PubMed.
- A. Rutsch, J. B. Kantsjo and F. Ronchi, The Gut-Brain Axis: How Microbiota and Host Inflammasome Influence Brain Physiology and Pathology, Front. Immunol., 2020, 11, 604179 CrossRef CAS PubMed.
- F. Li, Y. Wang and K. Zheng, Microglial mitophagy integrates the microbiota-gut-brain axis to restrain neuroinflammation during neurotropic herpesvirus infection, Autophagy, 2023, 19, 734–736 CrossRef CAS PubMed.
- A. Larabi, N. Barnich and H. T. T. Nguyen, New insights into the interplay between autophagy, gut microbiota and inflammatory responses in IBD, Autophagy, 2020, 16, 38–51 CrossRef CAS PubMed.
- M. Stephens and P. Y. von der Weid, Lipopolysaccharides modulate intestinal epithelial permeability and inflammation in a species-specific manner, Gut Microbes, 2020, 11, 421–432 CrossRef PubMed.
- H. Liang, X. Yang, C. Liu, Z. Sun and X. Wang, Effect of NF-kB signaling pathway on the expression of MIF, TNF-alpha, IL-6 in the regulation of intervertebral disc degeneration, J. Musculoskeletal Neuronal Interact., 2018, 18, 551–556 CAS.
- A. S. Baldwin Jr., The NF-kappa B and I kappa B proteins: new discoveries and insights, Annu. Rev. Immunol., 1996, 14, 649–683 CrossRef CAS PubMed.
- Y. Zhang, X. Gu, D. Li, L. Cai and Q. Xu, METTL3 Regulates Osteoblast Differentiation and Inflammatory Response via Smad Signaling and MAPK Signaling, Int. J. Mol. Sci., 2019, 21, 199 CrossRef PubMed.
- J. S. Arthur and S. C. Ley, Mitogen-activated protein kinases in innate immunity, Nat. Rev. Immunol., 2013, 13, 679–692 CrossRef CAS PubMed.
- Y. Ji, X. Fan, Y. Zhang, J. Li, Z. Dai and Z. Wu, Glycine regulates mucosal immunity and the intestinal microbial composition in weaned piglets, Amino Acids, 2022, 54, 385–398 CrossRef CAS PubMed.
- Z. Liu, M. Liu, H. Wang, P. Qin, Y. Di, S. Jiang, Y. Li, L. Huang, N. Jiao and W. Yang, Glutamine attenuates bisphenol A-induced intestinal inflammation by regulating gut microbiota and TLR4-p38/MAPK-NF-kappaB pathway in piglets, Ecotoxicol. Environ. Saf., 2023, 270, 115836 CrossRef PubMed.
- L. Liu, H. Guo, A. Song, J. Huang, Y. Zhang, S. Jin, S. Li, L. Zhang, C. Yang and P. Yang, Progranulin inhibits LPS-induced macrophage M1 polarization via NF-small ka, CyrillicB and MAPK pathways, BMC Immunol., 2020, 21, 32 CrossRef CAS PubMed.
- W. Zhao, L. Ma, C. Cai and X. Gong, Caffeine Inhibits NLRP3 Inflammasome Activation by Suppressing MAPK/NF-kappaB and A2aR Signaling in LPS-Induced THP-1 Macrophages, Int. J. Biol. Sci., 2019, 15, 1571–1581 CrossRef CAS PubMed.
- N. Dong, X. Li, C. Xue, L. Zhang, C. Wang, X. Xu and A. Shan, Astragalus polysaccharides alleviates LPS-induced inflammation via the NF-kappaB/MAPK signaling pathway, J. Cell Physiol., 2020, 235, 5525–5540 CrossRef CAS PubMed.
- H. N. Kim, G. H. Park, S. B. Park, J. D. Kim, H. J. Eo, H. J. Son, J. H. Song and J. B. Jeong, Sageretia thea Inhibits Inflammation through Suppression of NF- kappa B and MAPK and Activation of Nrf2/HO-1 Signaling Pathways in RAW264.7 Cells, Am. J. Chin. Med., 2019, 47, 385–403 CrossRef CAS PubMed.
|
This journal is © The Royal Society of Chemistry 2024 |
Click here to see how this site uses Cookies. View our privacy policy here.