DOI:
10.1039/D3FO03814A
(Paper)
Food Funct., 2024,
15, 125-138
Preparation of a novel expandable konjac fiber at different freezing temperatures and exploration of its digestion regulation functions†
Received
8th September 2023
, Accepted 13th November 2023
First published on 16th November 2023
Abstract
A new form of konjac fiber was successfully prepared, and it could instantaneously expand when in contact with the digestive fluid. The expanded konjac fiber could inhibit the digestion of the ingested food by competing with the substrate for digestive enzymes and space. The konjac fiber with desirable physical properties was obtained at 4 different freezing temperatures (−20 °C, −40 °C, −80 °C, and −196 °C), and the digestion regulation mechanisms of these fibers were systematically explored. The results showed that the konjac fiber prepared at −20 °C displayed an outstanding performance in delaying gastric emptying and preventing intestinal starch hydrolysis, while the fiber prepared under liquid nitrogen conditions (−196 °C) showed the weakest digestion regulation ability. However, the digestion regulation ability of this novel fiber was highly related to the food rheological property, and it exhibited a stronger interference effect on high-viscosity food. Our novel konjac fibers exhibited a great digestion regulation potential. Our findings provide valuable references for the development of dietary fiber-based satiety-enhancing functional foods.
1. Introduction
Obesity is a global pandemic chronic disease, which is associated with multiple metabolic syndromes that threaten human health.1,2 The increasing trend of obesity is unlikely to be reversed due to the occurrence of sedentary lifestyles and the preference for high-sugar and high-fat recipes, refined grains, and animal-based foods.3 Non-lifestyle interventions such as surgery and drug implementation do not seem to be the most suitable approaches, since they often require participants to have strong tolerance of the side effects.4 However, the development of satiety-enhancing functional foods, as one of the strategies of lifestyle intervention, is advisable in terms of accessibility and universality.5 Among a variety of alternatives, dietary fiber exhibits multiple advantages such as the low energy density, bulk effect, and fermentation function in the digestive tract.6–10
Both isolated dietary fiber and intact fiber in the cell wall can regulate gastric digestion, and this regulation highly depends on the rheological properties of food.11 Numerous studies have indicated that the viscosity of dietary fiber plays a central role in regulating nutrition absorption. In addition, some researchers have reported that the regulation of digestion by dietary fiber is closely related to its hydration and fermentative properties.12,13 Water-soluble dietary fibers, including pectin, guar gum, psyllium polysaccharides, and konjac glucomannan, could increase the viscosity of meals, thus slowing down gastric emptying and the transition of digesta through the intestine.14–16 Apart from viscosity properties, some types of dietary fibers cause gastric distention and slow down the digestion of nutrients by forming a bulking gel.17,18 Short-chain fatty acids produced by the anaerobic fermentation of fiber also contribute to its satiety-enhancing properties (Wanders et al., 2011).49 However, food supplemented with a large amount of dietary fiber tends to have unpleasant mouthfeel and poor acceptance. To avoid premature hydration, low-viscosity recipes have been developed to enable sufficient gelation in the gastric antrum,19 thus enhancing satiety.20 Alginate beads with a strong architecture can enhance satiety through the distension of the digest cavity.21 Therefore, developing novel forms of dietary fiber with satiety-enhancement function is essential.
Among a variety of dietary fibers, konjac glucomannan (KGM) shows very high viscosity in solution, conferring satiety, and thus it draws enormous attention.13,22 Noodles containing a larger amount of KGM have been reported to decrease the cumulative energy intake due to the KGM-induced satiety.23 Juices enriched with KGM and xanthan gum significantly decreased the appetite score, compared to the guar gum with its xanthan gum counterpart.24 With the increased viscosity of degraded konjac glucomannan, the levels of GLP-1, PYY3–36, and CCK-8 biomarkers were elevated, thereby enhancing satiety.25 However, it is not easy to handle the ultra-high viscosity of konjac glucomannan during food processing, and thus limiting the hydration process of fiber before ingestion seems to be an appealing strategy. The addition of incompletely hydrated KGM into cheese pies enables stepwise hydration in the digestive tract by forming solid-like structures.26 Emulsions encapsulated with KGM powder enable gradual hydration under gastric conditions, eventually generating high-viscosity clumps during digestion.27 Based on these findings, we hypothesized that limiting the hydration degree of konjac glucomannan-based foods might effectively weaken hunger.
A cryogenic treatment especially freeze–thaw is widely used to regulate the physical, chemical, structural, and mechanical properties of konjac foods because of its generic applicability and simple operation.28–30 Freeze–thaw treatment adjusts the pore size of the konjac gel.31,32 In addition, freeze–thawing alters the rheological response of the composite gel, increasing the G′ and hardness and reducing the elasticity values of the konjac gel. The underlying mechanism lies in the morphology and quantity of ice crystals formed during the freezing process, which are the replica gel pores that are closely related to the physicochemical properties of konjac foods. The size and distribution of ice crystals depend on the freezing rate, while the number is related to the freezing temperature.33 In short, freeze–thaw treatment has personalized konjac-based gel foods with different physicochemical properties.
Existing studies mostly focus on the digestion efficacy of soluble KGM in the form of an aqueous sol or an unstable gel-like substance, but the research on the insoluble or stable konjac glucomannan fiber is still lacking. It has been reported that konjac tofu and noodles or konnyaku konjac-based foods are almost completely hydrated before ingestion and disrupted under fluid invasion. This paper was aimed at preparing a novel form of konjac glucomannan unhydrated before ingestion. Once the dietary fiber comes in contact with the digestive juice, it will expand, but it will not be distorted in the later stage, and this space-occupying effect contributes to satiety. The fiber with various physical properties was obtained by adjusting the freezing temperature, and its critical properties were investigated. In addition, the mechanism by which konjac fiber regulated digestion through interference with hydrolytic enzymes was explored. The present work provides a simple and efficient method for preparing the novel form of konjac glucomannan fiber with a robust digestion regulation effect, which will promote the development and application of satiety-oriented functional food containing dietary fiber.
2. Materials and methods
2.1 Materials
Konjac glucomannan was purchased from the Hubei Konson Konjac Technology Co., Ltd (Wuhan, China). Japonica rice, indica rice (Simiao), and waxy rice were purchased from a local supermarket in Wuhan. These materials were subjected to chemical composition analyses (ESI 2.1†). Aspergillus niger amyloglucosidase (A7095, 260 U mL−1), porcine pancreas α-amylase (type VI-B, 14 U mg−1), and porcine gastric mucosa pepsin (P7125, 400 U mg−1 solid) were purchased from Sigma Chemical Co. (St. Louis, MO, USA). All the chemical reagents were of analytical grade.
2.2 Preparation of easily expanding konjac fiber
A 2% (w/w) konjac glucomannan powder was dispersed in sodium carbonate (0.24% w/w) and sodium bicarbonate (0.5% w/w) solution under constant stirring at 300 rpm for 2 min. After 4-hour standing, the resultant solution was heated at 90 °C for 1 h, and maintained at 37 °C for 1 h. Then, the gel was placed in the refrigerator at different temperatures (−20 °C, −40 °C, and −80 °C) for 24 h. The −196 °C KGM was obtained through 45-second fast-freezing in liquid nitrogen. Finally, the konjac glucomannan fiber was obtained by lyophilization and compression.
2.3 Konjac fiber physical properties
2.3.1 Hydration properties of konjac fiber.
The swelling ratio of konjac fiber was determined according to a previously reported method.34 The water retention capacity was estimated according to the method of centrifugation35 with slight modifications (ESI 2.2†).
2.3.2 Water migration in konjac fiber.
A low-field nuclear magnetic resonance analyzer (Suzhou Niumag Electric Corporation, Shanghai, China) was used to monitor the water distribution (ESI 2.3†).
2.3.3 Texture measurements.
The texture properties of konjac fiber were evaluated using a texture analyzer36 (ESI 2.4†).
2.3.4 Morphology observation.
The morphology of the konjac fiber was observed using scanning electron microscopy (JSM-6390LV, JEOL Ltd, Japan) with an acceleration voltage of 5 kV.
2.3.5 Volume of konjac fiber before and after gastric digestion.
The volume of konjac dietary fiber before and after gastric digestion was measured by the exclusion method (ESI 2.5†).
2.4 Konjac fiber digestion regulation
2.4.1
In vitro oral and gastric digestion.
In vitro digestion was conducted according to a previously reported method.16,37 To determine the effect of expanded konjac fiber on digestion with different substrates, three kinds of rice varieties were used to make a comparison.
Rice cooking.
To 200 g rice grains, 400 mL distilled water was added and cooked with a rice cooker (Midea Group Co., Ltd, Guangdong, China). Japonica rice and indica rice were cooked for 15 min, while the waxy rice was cooked for 25 min to ensure its palatability. All cooked rice was then stood at room temperature for 1 h before subsequent digestion analyses.
Simulated digestion fluid preparation.
The simulated saliva fluid (SSF) consisted of 1.1 mg mL−1 KCl, 0.5 mg mL−1 KH2PO4, 1.1 mg mL−1 NaHCO3, 0.03 mg mL−1 MgCl2(H2O)6, 0.2 mg mL−1 CaCl2(H2O)2, and 150 U mL−1 α-amylase. The SSF was adjusted to pH 7.0 ± 0.1 with 1 mol L−1 NaOH under magnetic stirring (150 rpm, 5 min).38
The simulated gastric fluid (SGF) was composed of 0.5 mg mL−1 KCl, 0.1 mg mL−1 KH2PO4, 2.1 mg mL−1 NaHCO3, 2.5 mg mL−1 NaCl, 0.02 mg mL−1 MaCl2(H2O)6, 0.02 mg mL−1 CaCl2(H2O)2, and 4000 U mL−1 pepsin. The SGF was adjusted to pH 3.0 ± 0.1 with 6 mol L−1 HCl under magnetic stirring (150 rpm, 10 min).38
In vitro gastric digestion protocol.
To mimic the oral digestion process, 150 g cooked rice and 120 mL SSF were mixed for 1 min and broken down in a food processor into less than 1–2 mm particles. The 1.5 g konjac fiber was loaded into the gastric compartment of a bionic human digestion system through the bionic esophagus without contact with saliva, and then the chyme was ingested into this system through a funnel within 5 min. The gastric digestion profile was analyzed using a near-real dynamic in vitro human stomach (DHS-IV) instrument (Xiao Dong Pro-health Instrumentation Co., Ltd, Suzhou, China) with excellent performance in mimicking gastric emptying. The instrument parameters were set as follows. The gastric juice secretion rate controlled by a peristaltic pump was set as 3.0 mL min−1. The pylorus opening and closing (controlled by the pylorus extrusion plate) was set at 3 cpm at a speed of 36 mm min−1. An auxiliary emptying instrument of the stomach model was used to simplify the digestion procedures: at 0° for 30 min, 0.2° counterclockwise rotation per minute for 60 min, and 0.5° counterclockwise rotation per minute for 30 min, respectively. At the end of the gastric digestion, the model exhibited an angle of 27°. The digesta was collected at 15 min intervals and weighed. The chyme was centrifugated at 4000g for 5 min to separate the supernatant and sediment. The supernatant volume was recorded and the sediment was freeze-dried using a freeze dryer.
2.4.2
In vitro small intestine digestion.
Small intestine digestion was performed according to the previously reported methods with slight modifications.39 The simulated intestinal fluid (SIF) consisted of 0.5 mg mL−1 KCl, 0.1 mg mL−1, KH2PO4, 7.1 mg mL−1 NaHCO3, 2.2 mg mL−1 NaCl, 0.07 mg mL−1 MaCl2(H2O)6, 3 mg mL−1 CaCl2(H2O)2, 42 U mL−1 α-amylase and 42 U mL−1 amyloglucosidase. The SIF was adjusted to pH 6.0 ± 0.1 with 6 mol L−1 HCl under magnetic stirring (150 rpm, 10 min).38,39
The 0.04 g konjac fiber after gastric digestion and the freeze-dried chyme after gastric emptying (equivalent to 100 mg dry starch) were dispersed in the SIF and placed in a 37 °C water bath for 2 h with magnetic stirring at 130 rpm. At the indicated time, an aliquot (0.1 mL) of the hydrolyzed substrate was withdrawn and 0.9 mL 95% ethanol was added to stop the digestion reaction. After centrifugation at 13
000 rpm for 3 min, the amount of glucose in the supernatant was determined using a glucose oxidase reagent kit. The percentage of starch hydrolysis was calculated as the glucose content multiplied by 0.9. The starch fraction contents were calculated according to the following equations:
| 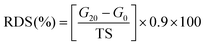 | (1) |
| 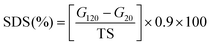 | (2) |
| 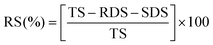 | (3) |
where RDS, SDS, and RS refer to rapidly digestible starch, slowly digestible starch, and resistant starch, respectively; TS represents the total starch content in the test system;
G0,
G20, and
G120 correspond to the glucose content released from the digesta after hydrolysis for 0, 20, and 120 min respectively; and 0.9 refers to the conversion coefficient from glucose to starch. Moreover, the digestibility rate curve (the logarithm of the slope (LOS)
vs. various time points) was plotted according to the method from a previous report.
40–42
2.5 Konjac fiber digestion regulation mechanism
2.5.1 Effect of konjac fiber on the absorption of hydrolytic enzymes.
10 mg mL−1 konjac fiber was incubated in 0.2 M acetate sodium buffer (pH 6.0) containing 3 mg mL−1 α-amylase and 162 μL amyloglucosidase at 37 °C for 2 h. The expanded konjac fiber was withdrawn, and the remaining system was supplemented with acetate sodium buffer to a volume of 5 mL.38 The amount of protein in this supplemented system was determined by using Bradford enzyme kits. The enzyme absorption was calculated according to the following formula.
2.5.2 Confocal laser scanning microscopy observation.
The cryo-sectioning technique (Leica CM 1950) was employed to slice the konjac fiber after intestinal digestion with a slice thickness of 80 μm. Calcofluor white (1 mg mL−1) and Nile red (1 mg mL−1) were used to dye the polysaccharide and enzyme, respectively. The confocal image of the konjac fiber was obtained using microscopy (model IX3-CBH, Olympus) at a 405 nm emission wavelength for Calcofluor white and 488 nm for Nile red.
2.5.3 Fluorescence spectra.
The fluorescence spectra were analyzed according to a previously reported method.38 Specifically, 10 mg mL−1 konjac fiber was mixed with 0.5 mg mL−1 α-amylase in acetate sodium buffer (0.2 M, pH 6), and then the fluorescence spectra of the mixture system were measured at a 295 nm excitation wavelength and 300–400 nm emission wavelength, respectively, with a slit width of 5 nm.
2.5.4 Michaelis–Menten kinetics of hydrolysis reaction.
The kinetics assay was conducted according to a previously reported method with slight modifications.43 Specifically, starch powder (20 mg, 25 mg, 40 mg, 60 mg, 80 mg, and 100 mg) was dispersed in 4 mL acetate sodium buffer (0.2 M, pH 6.0) containing 2 U mL−1 fresh prepared enzyme solution. The konjac fiber after gastric digestion was added into the reaction tubes (10 mg mL−1) and placed in a water bath at 37 °C with magnetic stirring. The 200 μL digesta was withdrawn at 4 min, 8 min, and 12 min post-digestion and mixed with 200 μL sodium carbonate solution (0.5 M) to terminate the reaction. The mixture solution was centrifuged at 13
000g for 3 min. After centrifugation, 50 μL supernatant was mixed with 1.5 mL PAHBAH solution and heated for 3 min. Subsequently, the reducing sugar content of the mixture solution was measured at 410 nm absorbance. The reaction velocity was calculated according to the Michaelis–Menton equation: |  | (4) |
where v refers to the reaction velocity (mg mL−1 min−1); Vmax is the maximum enzyme reaction velocity (mg mL−1 min−1); [S] represents the substrate concentration (mg mL−1); and Km is the Michaelis constant (mg mL−1).
2.6 Statistics
The data were expressed as mean ± SD of three replicates. ANOVA and Duncan (P < 0.05) test were performed to determine the significant differences using SPSS 23.0 software. The Pearson correlation analysis was conducted using Origin 2021 software (OriginLab Inc., Massachusetts, USA).
3. Results
3.1 Hydration properties of konjac fiber
3.1.1 Swelling rate and water-retention capacity.
All the konjac fibers prepared at different temperatures could absorb the solution within less than 1 minute. Compared with −80 °C, the higher KGM preparation temperatures (−20 °C and −40 °C) could promote the formation of an easily absorbable architecture in the early stage. After liquid nitrogen freezing, the konjac fiber showed a steady low gastric liquid absorption value at different measurement time points, indicating that liquid nitrogen freezing restricted gastric liquid absorption by the fiber (Fig. 1A). The physical properties of the konjac fiber mostly rely on the ice crystal growth condition. Smaller ice particles endow the fiber with a more uniform rigid architecture, thus negatively affecting the swelling capacity of the fiber.44
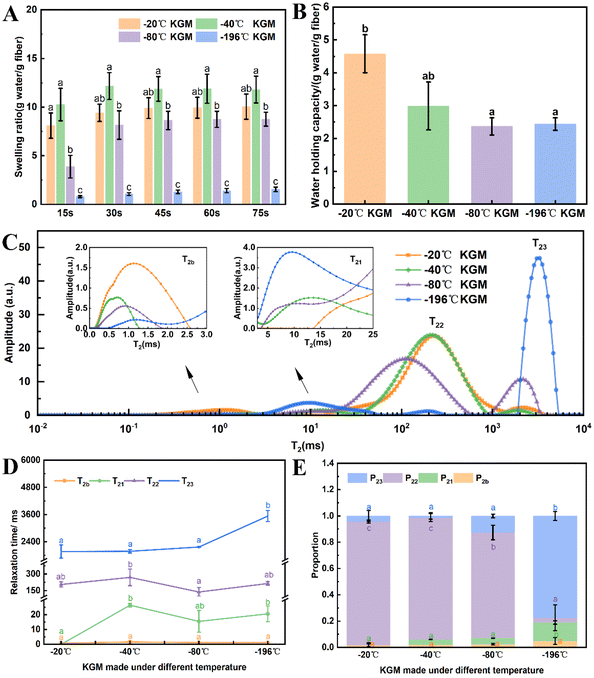 |
| Fig. 1 Hydration properties of konjac fiber. (A) The swelling rate of konjac fiber prepared at different freezing temperatures. (B) The water-holding capacity of konjac fiber prepared at different freezing temperatures. (C) Distributed T2 relaxation times of konjac fiber (T2b and T21 refer to bound water, T22 refers to immobilized water, and T23 refers to free water; the inset figure represents the enlarged plot of T2b and T21 populations, respectively). (D) T2 relaxation time and (E) the proportion of water distribution of konjac fiber. Different lower-case letters represent significant difference at p < 0.05. | |
With the decreasing freezing temperature, the konjac fiber showed a gradually weakened water-retention capacity. The −20 °C KGM (prepared at −20 °C) showed the best water-holding performance, followed by −40 °C KGM, and −80 °C KGM and −196 °C KGM presented poor holding performance (Fig. 1B). Besides, the water-holding ability tests indicated that this novel form of fiber was subjected to repeated hydration and re-expansion after gastric digestion (Fig. 2 and ESI Video S1†). Moreover, the different water-holding capacities of konjac fiber were in line with its swelling rate, both of which depended highly on the freezing parameters. When the ice crystals grow at low temperatures, the fiber exhibits more dendritic structures, which may prevent liquid absorption by the fiber.45
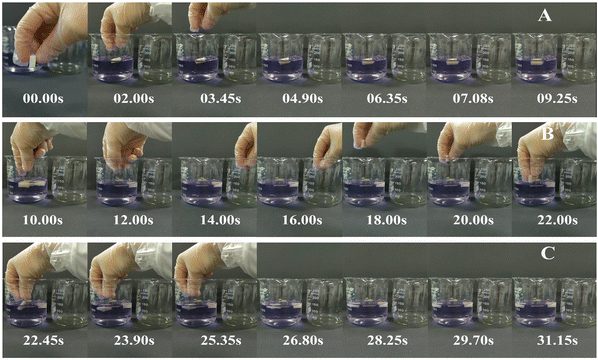 |
| Fig. 2 The swelling and re-expanding features of konjac fiber in an aqueous solution. (A) The swelling process of original konjac fiber in an aqueous phase. (B) The squeezing process of konjac fiber. (C) The re-expanding process of konjac fiber. The purple solution was 0.001 mg mL−1 aqueous crystal violet. | |
3.1.2
T
2 relaxation time and water distribution.
The tested konjac fiber exhibited 4 water populations with different T2 relaxation times (Fig. 1C). With the decreasing konjac fiber preparation temperature, the profile of the sample shifted to the right, indicating the increased number of free water protons. The proton pool T2b of −20 °C KGM was larger than that of −196 °C KGM while its proton pool T23 was smaller than that of −196 °C KGM, implying that low-temperature preparation of samples led to a worse water absorption. As shown in Fig. 1D, the relaxation time of bound water of −20 °C KGM was significantly lower than that of −196 °C KGM (T21). Although T22 corresponding to immobilized water exhibited no significant difference among different KGM samples, the T23 value of −196 °C KGM was much higher than those of other KGM samples (prepared at −20 °C, −40 °C, and −80 °C). Overall, the lower the fiber preparation temperature, the higher the unbound water content in the test system (Fig. 1E). That is, the KGM fiber prepared at gradually decreasing temperatures displayed a progressively worse water hydration performance.
3.2 Texture analysis
Texture analysis can provide a comprehensive evaluation of the physical properties of the konjac fiber, and these physical properties may affect consumers’ acceptance and preference.29 As shown in Fig. 3A, the hardness of −20 °C KGM was the lowest (only 194.03 N); −40 °C KGM and −80 °C KGM displayed a similar hardness, which was 429.72 N and 466.62 N, respectively; and −196 °C KGM exhibited the highest hardness (3495.68 N). The chewiness value showed the same pattern with the highest value (2592.95 N) observed in −196 °C KGM and the lowest value (155.32 N) in −20 °C KGM. With the decrease in the preparation temperature, springiness, cohesiveness, and resilience showed a trend of decline first and then an increase (Fig. 3B). The marked change of texture parameters was attributed to the physical effects of the formed ice crystals under different conditions. Under fast freezing rates, the fiber produced finer microstructures, which was also confirmed by previous research.46
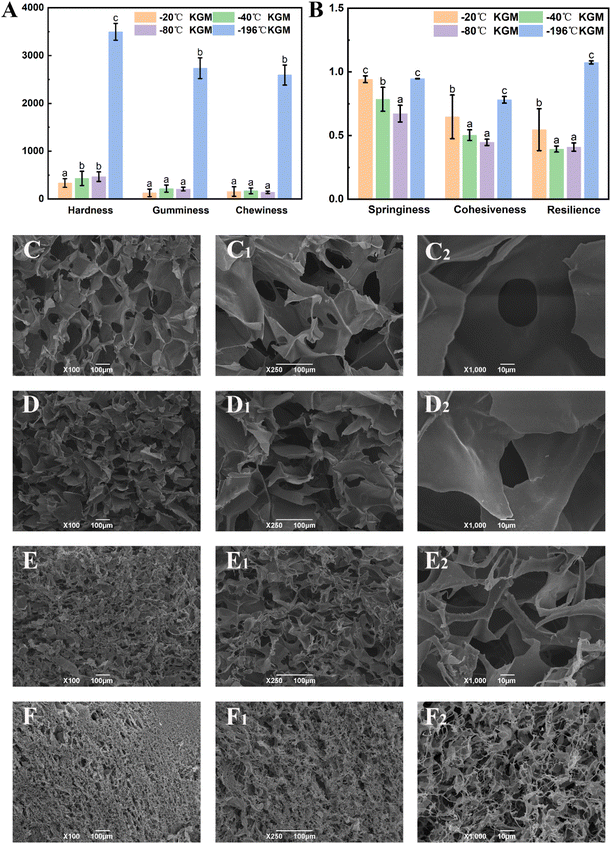 |
| Fig. 3 Structural properties of konjac fiber. Textural properties such as hardness, gumminess, and chewiness (A) and springiness, cohesiveness, and resilience (B) of konjac fiber. The SEM images of konjac fiber (C: −20 °C KGM, D: −40 °C KGM, E: −80 °C KGM, and F: −196 °C KGM) under 100× (C–F), 250× (C1–F1), and 1000× magnification (C2–F2). The scale bar is 100 μm and 10 μm, respectively. | |
3.3 Morphological observation
Undoubtedly, the texture and hydration properties of konjac fiber are related to its microscopic structural features. Considering this, the morphological differences among samples were investigated by scanning electron microscopy (SEM). The results showed that the size of the pores of −20 °C KGM was the largest, which was nearly 100–200 μm. When the temperature was lower than −20 °C, a more compact structure with decreasing pore size was observed (Fig. 3C–F). The −80 °C KGM exhibited a significantly increased pore number and a mutually interconnected pore morphology. Furthermore, liquid nitrogen treatment (−196 °C) caused a tightly stacked structure (Fig. 3F–F2), accompanied by a small pore size and an increased pore number. High magnification observation (1000×) showed filamentous fibers and dense pores (Fig. 3F2). Therefore, different freezing temperatures resulted in differences in the pore size and number.
This new form of konjac fiber was prepared by employing the ice-templating technique, and the pore morphology of the obtained fiber depended highly on the solidification conditions.45 The architecture of the konjac fiber is closely associated with the ice crystal formation process. At a high freezing temperature (−20 °C), the ice crystal growth rate was quicker than the nucleation rate, resulting in large-sized ice crystal formation. Once the crystal growth rate slowed down (−40 °C), the rapid heat transfer favored the small-sized ice crystal formation. With the decreasing temperature (−196 °C), freezing rates increased, and konjac fiber presented a finer microstructure.46
3.4 Retention of gastric digesta
Specific food structures affect appetite by enhancing gastric digesta retention and reducing the gastric emptying rate.47 The konjac fiber is characterized by the expansion in the digestive tract, thereby occupying space and delaying gastric emptying action.
The fiber delayed gastric emptying of japonica rice chyme especially in the early stage (at 15 min post-digestion) by reducing the discharge of both the supernatant and dry mass in the stomach (Fig. 4A–A2). With the prolongation of digestion, the difference in gastric emptying delay between the experimental and control groups diminished. The −20 °C KGM fiber exhibited optimal gastric digesta retention by simultaneously postponing the discharge of aqueous and solid mass chyme from the stomach, followed by −40 °C KGM or −80 °C KGM. The −196 °C KGM fiber showed the poorest gastric retention of japonica rice. Indica rice has the highest starch content, exhibiting easier gastric digestion than japonica rice (Table S1†). After coming in contact with salivary amylase, the indica rice chyme becomes semi-fluid, thus easily passing through the pyloric sphincter. The konjac fiber ingested into the antrum hindered the rice contact with the fluid, hence impairing gastric digestion and blocking the discharge of the chyme (Fig. 4B), except the −196 °C KGM which had no significant effect on gastric digesta retention. At 15 min post-digestion, −20 °C KGM exhibited the largest gastric retention amount of indica rice chyme, while −196 °C KGM displayed the smallest retention amount, which was associated with the sharply decreased volume of −196 °C KGM (Fig. 5), and its reduced water-holding capacity after gastric digestion (Fig. 1B). Waxy rice was more easily digested than the other two varieties (Fig. 4C), since waxy rice contained a large amount of amylopectin, resulting in an easier conversion from a viscous bolus to a fluid after coming in contact with amylase (ESI Fig. S1 and S2†). Konjac fiber displayed the lowest gastric retention of waxy rice chyme (ranging from 38.36% to 46.30%) compared with the other two varieties (Fig. 4C). Although −20 °C KGM presented a slightly enhanced gastric retention of waxy rice, this retention effect was gradually weakened with the extension of digestion time.
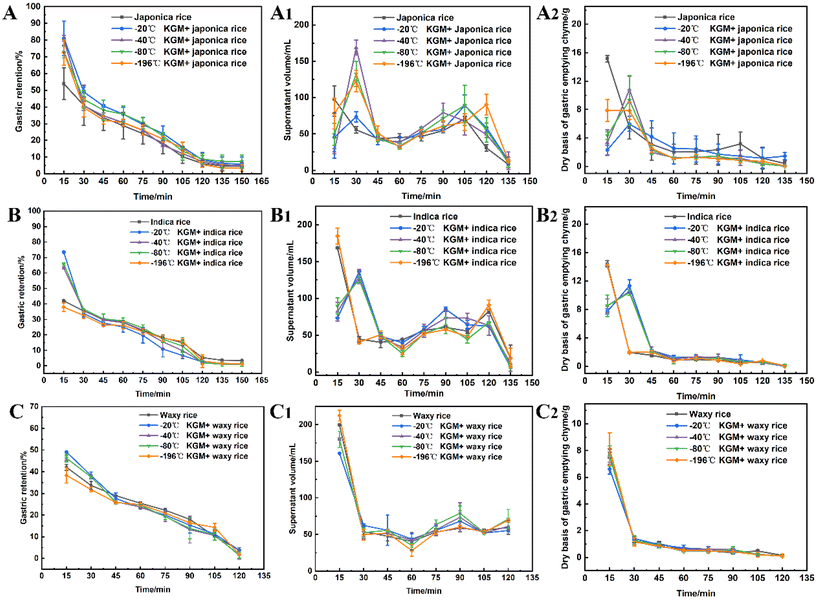 |
| Fig. 4 Gastric retention of japonica rice chyme (A), indica rice chyme (B), and waxy rice chyme (C) with (or no) konjac fiber explored with a DHS-IV instrument. The supernatant volume of the gastric emptying digesta of the japonica rice (A1), indica rice (B1), and waxy rice (C1) with konjac fiber. The dry basis content of gastric emptying chyme of the japonica rice (A2), indica rice (B2), and waxy rice (C2) with konjac fiber. | |
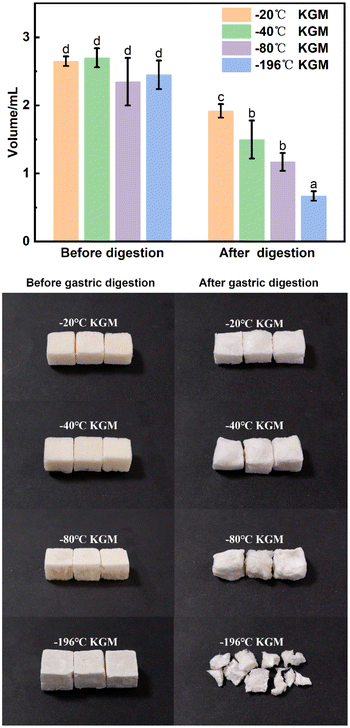 |
| Fig. 5 Volume and morphology of konjac fiber after gastric digestion. | |
Taken together, konjac fiber delayed the gastric emptying of chyme to various extents, which was associated with the rheological properties of the substrates (ESI Fig. S2 & S3†). Waxy rice showed a significantly lower viscosity after coming in contact with amylase than japonica rice and indica rice. These results suggested that the novel form of konjac fiber could exert more prominent satiety-enhancement effects when dealing with viscous, less fluid substrates.
3.5 Volume of konjac fiber after gastric digestion
The konjac fiber has an expansion structure which is retained even under the large action force from the scroll wheel to mimic the actual movement during stomach digestion. The −20 °C KGM retained the most intact morphology, while the morphology of −196 °C KGM was damaged most severely. The −40 °C KGM and −80 °C KGM displayed slight deformation (Fig. 5). The deformation is reliant on the preparation temperature, since the pores are the replica of the ice crystals, and the pore morphology is controlled by the freezing kinetics.44,45 The initial planar ice front could trap the polymer molecules, resulting in the formation of a dense layer of the sample (ESI Fig. S5†). With the decreasing freezing rate, the ice front movement velocity was decreased, thus leading to the formation of a columnar microstructure, followed by the conversion of the columnar microstructure into a lamellar microstructure.48 These hierarchical structures were subjected to different deformations under gastric stress (Fig. 5). After liquid nitrogen fast freezing, some cracks occurred on the surface of the konjac fiber, making the fiber liable to collapse. With the decrease in the freezing temperatures, the deformation rate of the fiber gradually increased, and fiber volume was significantly decreased.
3.6
In vitro small intestine digestion
Fig. 6 shows the effect of konjac fiber on the small intestine digestion of three types of cooked rice chyme. Both japonica and indica rice showed a rapid digestion in the initial 40 min hydrolysis, followed by a slow digestion, while waxy rice was hydrolyzed rapidly at an initial 20 min, and then digested slowly. All konjac fiber inhibited starch hydrolysis. The −20 °C KGM exhibited the most significant starch hydrolysis inhibition effect on different rice varieties, while −196 °C KGM displayed the weakest inhibition effect (Fig. 6B and C), and −40 °C and −80 °C KGM showed a moderate inhibition effect. In addition, our results showed that konjac fiber reduced the rapidly digestible starch content significantly, but increased the resistant starch content (Fig. 6D–F). Fiber with different physical properties (such as texture) showed no differences in perturbing rapidly digestible starch content and resistant starch content. To investigate the effects of the konjac fiber on rice digesta hydrolysis, the LOS kinetics parameters were estimated. All the tested fibers exhibited a decreased hydrolysis reaction rate (k value). To be more specific, −20 °C KGM exhibited an evident inhibitory effect on the reaction rate constant of japonica rice and indica rice. However, the presence of konjac fiber (−20 °C) had no significant effect on the k value of waxy rice. Indica rice with −20 °C KGM fiber added showed a flat LOS curve, indicating that the −20 °C KGM fiber significantly inhibited the hydrolysis of indica rice, while indica rice with other KGM fibers added displayed a gradually steep LOS curve, suggesting the weak hydrolysis inhibition by other KGM fibers (especially −196 °C KGM fiber). The konjac fiber with different physical properties showed no significant regularity in the interference with the hydrolysis rate of the other two varieties of rice digesta (Fig. 6A1, C1, and ESI Fig. S4, and Table S2†).
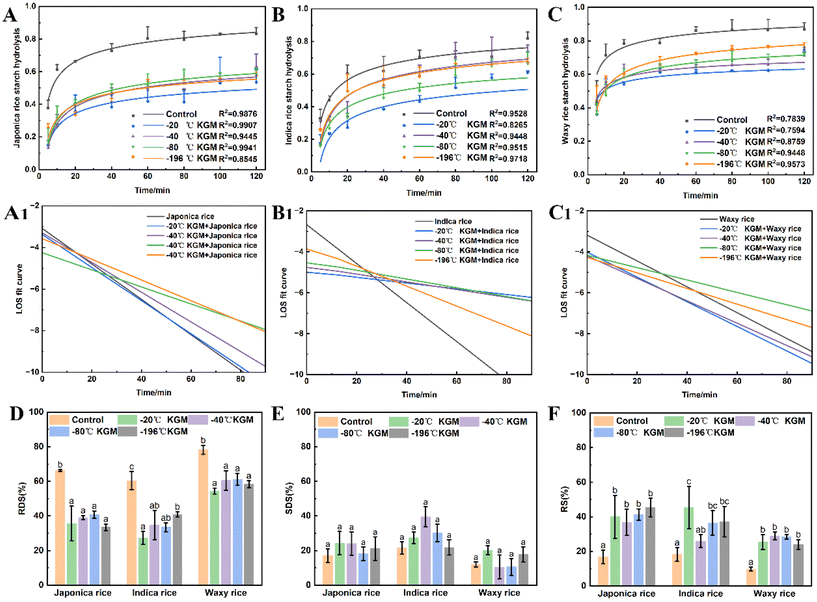 |
| Fig. 6 Starch hydrolysis diagram of japonica rice chyme (A), indica rice chyme (B), and waxy rice chyme (C) and first-order kinetics curve of starch. Kinetic constants of japonica rice chyme (A1), indica rice chyme (B1), and waxy rice chyme (C1). Starch fraction of japonica rice, indica rice, and waxy rice chyme. Rapid digestion starch (D), slow digestion starch (E) and resistant starch fractions (F). | |
3.7 Mechanism by which konjac fiber regulates digestion
As mentioned above, the novel form of konjac fiber inhibited chyme digestion. Hence, the interference effect of the fiber on enzymes was evaluated to reveal the underlying mechanism. The −20 °C KGM and −40 °C KGM fibers exhibited the highest absorption capacity towards hydrolytic enzymes, while −80 °C and −196 °C fibers showed poor absorption capacity (Fig. 7A). The absorption capacity of the fiber is associated with its honeycomb-like micro-network structure, which endows the fiber with loose and easy-expansion features. With the decreasing freezing temperature, the rigid structure of the −196 °C fiber made it difficult to absorb the hydrolytic enzymes, thus weakening its digestion regulation capacity. Another distinctive feature of the konjac fiber is that in the case of being squeezed or crushed, konjac fiber can retain its original morphology once it is in contact with the aqueous solution. This feature was confirmed by the microscopy observation of the fibers after gastric digestion (Fig. 7C–F). In addition, after digestion, the −20 °C and −40 °C fibers retained a normal structure, while the −80 °C konjac fiber was distorted with twisted and deformed pores. The laser confocal image showed that the −196 °C konjac fiber had a densely stacked architecture, which was consistent with SEM observation (Fig. 3D). The −20 °C KGM exhibited a better enzyme absorption capacity (Fig. 7C1 and C2), leading to a significant inhibitory effect on starch hydrolysis.
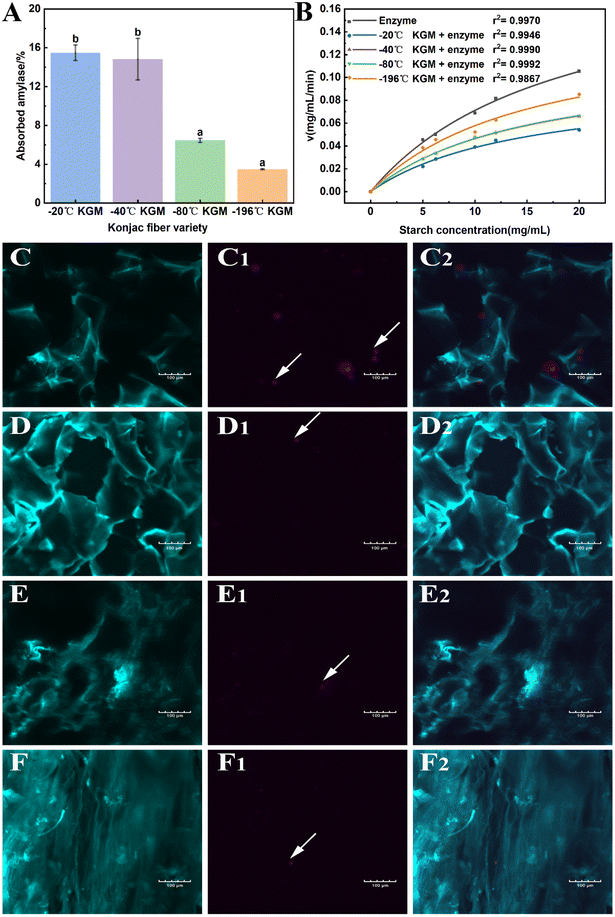 |
| Fig. 7 (A) Konjac fiber absorption of hydrolytic enzymes. (B) Konjac fiber hindered α-amylase-catalyzed kinetic reaction. Microstructure of digested konjac fiber: (C–F) calcofluor white dyes the fiber, (C1–F1) Nile red dyes the enzyme, and (C2–F2) merged images. The scale bar was 100 μm. | |
Furthermore, the Michaelis–Menten reaction parameters were evaluated. Our prepared konjac fiber, especially −20 °C KGM, acted as an amylase inhibitor, exhibiting a lower reaction rate and a lower Km value than the control without fiber addition (Fig. 7B and ESI Table S3†). The −196 °C KGM fiber showed the weakest affinity towards enzymes, and the Vmax and Km were the closest to those of the control. Therefore, the novel form of konjac fiber interacted with the enzyme, thus modifying the kinetics of the enzymatic reaction, eventually decelerating the hydrolysis of the substrates.
3.8 Correlation analysis
Pearson correlation analysis was performed to reveal the relationship between the physical properties of konjac fibers and digestion regulation capacity. The physical properties of the fibers include absorption, water-holding capacity, textural properties (such as hardness and chewiness), and morphological characteristics.
Significant correlation was observed among the internal physical properties of fibers (Fig. 8). After digestion, the fiber volume was positively correlated with water absorption (r2 = 0.860, p < 0.001) and water-holding capacity (r2 = 0.866, p < 0.001), but it was negatively correlated with hardness (r2 = −0.830, p < 0.001) and chewiness (r2 = −0.806, p < 0.001). Since the rigid architecture of the fiber restricted water accessibility, the fiber volume became smaller after digestion. Therefore, the volume of the fiber was positively correlated with water-holding capacity.
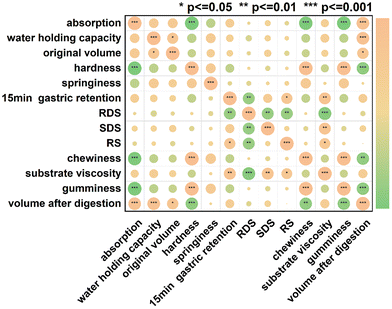 |
| Fig. 8 Pearson analysis between physical properties and functional parameters. | |
Our data showed that the delay of gastric emptying by the konjac fiber was positively correlated with the substrate viscosity (r2 = 0.763, p < 0.001). Additionally, the starch types showed a significant correlation with viscosity. Rapidly digestible starch (RDS) content exhibited a negative correlation with the viscosity (r2 = −0.897, p < 0.001), while slowly digestible starch (SDS) and resistant starch (RS) contents displayed a positive correlation with viscosity (r2 = 0.741, p < 0.01; r2 = 0.616, p < 0.05). Gastric emptying influenced the RDS and RS content. The enhanced chyme gastric retention resulted in a decrease in RDS content, but an increase in RS content.
4. Discussion
This study prepared a new type of konjac fiber, which could enhance satiety and promote health with desirable digestive regulatory capabilities. This konjac fiber preparation strategy has never been reported. By regulating the freezing conditions, we obtained konjac fibers with different physical properties (swelling rate, water holding capacity, texture, and pore size) and digestion regulation functions.
Since the pores in the konjac fiber are replicas of the ice crystals, the shape and number of ice crystals during the freezing process determine the products with different physicochemical properties. When the freezing temperature is low, the freezing rate is fast, and the ice crystal nucleation rate is faster than the ice crystal growth rate, thus producing small and large numbers of crystal grains in the konjac gel, eventually resulting in the formation of densely arranged pores and a relatively rigid structure of the konjac fiber after freeze-drying. It is this relatively rigid structure that limits the water absorption and water-holding capacity of konjac fiber, resulting in a small volume after hydration and enhancement in hardness and chewiness. In contrast, at a freezing temperature of −20 °C, the growth of ice crystals is in a dominant position, and relatively large ice crystals are formed in the system. The sublimation of large ice crystals enlarges the pore size of the konjac fiber and loosens its structure. The porous network of konjac fiber provides favorable conditions for its hydration, thus significantly enhancing the water absorption and water holding capacities, ultimately reducing the hardness of the konjac fiber.
The interference effect of konjac fiber on substrate digestion mainly lies in the absorption of hydrolase, thus regulating the utilization rate of enzymes in the reaction system and interfering with the reaction kinetics of enzymes, ultimately hindering chyme digestion. When the hydration capacity of konjac fiber is poor, the absorption of hydrolytic enzymes during digestion is limited. When the konjac fiber prepared at −196 °C (low-temperature conditions) was added to the reaction system, the enzyme hydrolysis reaction rate was less affected (indica rice chyme system), and interference with chyme digestion was the weakest. In contrast, konjac fiber with strong hydration capacity exhibited high amylase absorption ability in the reaction system, thus reducing the enzyme reaction kinetics. When the konjac fiber prepared at −20 °C was added to the reaction system, the strongest inhibitory effect on chyme digestion was observed (especially indica rice system), which was conducive to maintaining blood sugar stability and promoting health.
Furthermore, our results indicate that substrate viscosity plays a dominant role in the regulation of fiber digestion. Substrate viscosity was negatively correlated with enhanced gastric retention and starch hydrolysis. This is consistent with previous reports that sticky food in the antrum may prevent premature gastric emptying and contribute to the secretion of hormones associated with satiety.47 However, viscosity may not be the only factor influencing appetite and blood sugar responses, and this still deserves further investigation.
In summary, this study prepared a new type of konjac fiber that could absorb liquid and swell in the digestive tract to exert a space-occupying effect. In addition, this konjac fiber has strong digestion regulatory potential, and it can act directly on hydrolytic enzymes to interfere with the digestion rate and degree of hydrolysis of substrates, ultimately enhancing satiety and promoting health. Our findings provide a valuable reference for the development of dietary fiber functional foods.
Conflicts of interest
The authors declare no competing financial interest.
Acknowledgements
This work was financially supported by the National Natural Science Foundation of China (Grant No. 32172200).
References
- L. M. Jaacks, S. Vandevijvere, A. Pan, C. J. McGowan, C. Wallace, F. Imamura, D. Mozaffarian, B. Swinburn and M. Ezzati, The obesity transition: stages of the global epidemic, Lancet Diabetes Endocrinol., 2019, 7, 231–240 CrossRef PubMed.
- K. Chen, Z. W. Shen, W. J. Gu, Z. H. Lyu, X. Qi, Y. Mu and Y. Ning, Prevalence of obesity and associated complications in China: A nationwide, cross-sectional, observational, real-world study in 15.8 million adults, Diabetes, Obes. Metab., 2023, 1–10, DOI:10.1111/dom.15238.
- Q. Zeng, N. Li, X. F. Pan, L. Chen and A. Pan, Clinical management and treatment of obesity in China, Lancet Diabetes Endocrinol., 2021, 9, 393–405 CrossRef.
- H. Yang, H. Yang, C. Zhu, D. Fan and J. Deng, Highly expandable edible hydrogels for the prevention and treatment of obesity through dietary intervention, Food Hydrocolloids, 2023, 144, 108946 CrossRef CAS.
- P. E. S. Munekata, J. Á. Pérez-Álvarez, M. Pateiro, M. Viuda-Matos, J. Fernández-López and J. M. Lorenzo, Satiety from healthier and functional foods, Trends Food Sci. Technol., 2021, 113, 397–410 CrossRef CAS.
- Y. Zhang, Q. Xie, L. You, P. C. K. Cheung and Z. Zhao, Behavior of non-digestible polysaccharides in gastrointestinal tract: A mechanistic review of its anti-obesity effect, eFood, 2021, 2, 59–72 CrossRef.
- H. D. Goff, N. Repin, H. Fabek, D. E. Khoury and M. J. Gidley, Dietary fibre for glycaemia control: Towards a mechanistic understanding, Bioact. Carbohydr. Diet. Fibre, 2018, 14, 39–53 CrossRef CAS.
- M. Akhlaghi, The role of dietary fibers in regulating appetite, an overview of mechanisms and weight consequences, Crit. Rev. Food Sci. Nutr., 2022, 1–12 CrossRef PubMed.
- S. Sutehall, S. D. R. Galloway, A. Bosch and Y. Pitsiladis, Addition of an alginate hydrogel to a carbohydrate beverage enhances gastric emptying, Med. Sci. Sports Exerc., 2020, 52, 1785–1792 CrossRef CAS.
- M. Wang, S. Wichienchot, X. He, X. Fu, Q. Huang and B. Zhang, In vitro colonic fermentation of dietary fibers: Fermentation rate, short-chain fatty acid production and changes in microbiota, Trends Food Sci. Technol., 2019, 88, 1–9 CrossRef CAS.
- D. Y. Low, A. M. Pluschke, B. Flanagan, F. Sonni, L. J. Grant, B. A. Williams and M. J. Gidley, Isolated pectin (apple) and fruit pulp (mango) impact gastric emptying, passage rate and short chain fatty acid (SCFA) production differently along the pig gastrointestinal tract, Food Hydrocolloids, 2021, 118, 106723 CrossRef CAS.
- A. D. Blackwood, J. Salter, P. W. Dettmar and M. F. Chaplin, Dietary fibre, physicochemical properties and their relationship to health, J. R. Soc. Promot. Health, 2000, 120, 242–252 CrossRef CAS PubMed.
- L. Guo, W. Yokoyama, M. Chen and F. Zhong, Konjac glucomannan molecular and rheological properties that delay gastric emptying and improve the regulation of appetite, Food Hydrocolloids, 2021, 120, 106894 CrossRef CAS.
- T. M. S. Wolever, S. M. Tosh, S. E. Spruill, A. L. Jenkins, A. Ezatagha, R. Duss, J. Johnson, Y. Chu and R. E. Steinert, Increasing oat beta-glucan viscosity in a breakfast meal slows gastric emptying and reduces glycemic and insulinemic responses but has no effect on appetite, food intake, or plasma ghrelin and PYY responses in healthy humans: a randomized, placebo-controlled, crossover trial, Am. J. Clin. Nutr., 2020, 111, 319–328 CrossRef.
- A. Tamargo, C. Cueva, M. D. Alvarez, B. Herranz, M. V. Moreno-Arribas and L. Laguna, Physical effects of dietary fibre on simulated luminal flow, studied by in vitro dynamic gastrointestinal digestion and fermentation, Food Funct., 2019, 10, 3452–3465 RSC.
- L. C. Shang, Y. Wang, Y. Ren, T. Ai, P. Zhou, L. Hu, L. Wang, J. Li and B. Li, In vitro gastric emptying characteristics of konjac glucomannan with different viscosity and its effects on appetite regulation, Food Funct., 2020, 11, 7596–7610 RSC.
- C. L. Hoad, P. Rayment, R. C. Spiller, L. Marciani, B. d. C. Alonso, C. Traynor, D. J. Mela, H. P. F. Peters and P. A. Gowland, In vivo imaging of intragastric gelation and its effect on satiety in human, J. Nutr., 2004, 134, 2293–2300 CrossRef CAS.
- H. P. Peters, R. J. Koppert, H. M. Boers, A. Strom, S. M. Melnikov, E. Haddeman, E. A. Schuring, D. J. Mela and S. A. Wiseman, Dose-dependent suppression of hunger by a specific alginate in a low-viscosity drink formulation, Obesity, 2011, 19, 1171–1176 CrossRef CAS.
- A. Ström, H. M. Boers, R. Koppert, S. M. Melnikov, S. Wiseman and H. P. F. Peters, Physico-chemical properties of hydrocolloids determine their appetite effects, Gums Stab. Food Ind., 2009, 15, 341–356 Search PubMed.
- S. Fiszman and P. Varela, The role of gums in satiety/satiation. A review, Food Hydrocolloids, 2013, 32, 147–154 CrossRef CAS.
- C. Hoad, P. Rayment, E. Cox, P. Wright, M. Butler, R. Spiller and P. Gowland, Investigation of alginate beads for gastro-intestinal functionality, Part 2: In vivo characterisation, Food Hydrocolloids, 2009, 23, 833–839 CrossRef CAS.
- L. P. Guo, W. Yokoyama, L. Chen, F. Liu, M. Chen and F. Zhong, Characterization and physicochemical properties analysis of konjac glucomannan: Implications for structure-properties relationships, Food Hydrocolloids, 2021, 120, 106818 CrossRef CAS.
- F. Au-Yeung, E. Jovanovski, A. L. Jenkins, A. Zurbau, H. V. T. Ho and V. Vuksan, The effects of gelled konjac glucomannan fibre on appetite and energy intake in healthy individuals: a randomised cross-over trial, Br. J. Nutr., 2018, 119, 109–116 CrossRef CAS.
- É. Paquet, A. Bédard, S. Lemieux and S. L. Turgeon, Effects of apple juice-based beverages enriched with dietary fibres and xanthan gum on the glycemic response and appetite sensations in healthy men, Bioact. Carbohydr. Diet. Fibre, 2014, 4, 39–47 CrossRef.
- L. C. Shang, T. Ai, J. Li and B. Li, Correlations between sol viscosity of the partially degraded konjac glucomannan and appetite response of rats, Food Hydrocolloids Health, 2021, 1, 100026 CrossRef CAS.
- J. Marcano, I. Hernando and S. Fiszman, In vitro measurements of intragastric rheological properties and their relationships with the potential satiating capacity of cheese pies with konjac glucomannan, Food Hydrocolloids, 2015, 51, 16–22 CrossRef CAS.
- J. Wu and Q. Zhong, Encapsulation of konjac glucomannan in oil droplets to reduce viscosity of aqueous suspensions and gradually increase viscosity during simulated gastric digestion, J. Food Eng., 2016, 175, 104–107 CrossRef.
- R. Lai, J. Liu and Y. Liu, Effects of pH and incubation temperature on properties of konjac glucomannan and zein composites with or without freeze-thaw treatment, Food Hydrocolloids, 2023, 134, 108098 CrossRef.
- L. Shang, C. Wu, S. Wang, X. Wei, B. Li and J. Li, The influence of amylose and amylopectin on water retention capacity and texture properties of frozen-thawed konjac glucomannan gel, Food Hydrocolloids, 2021, 113, 106521 CrossRef CAS.
- X. Ni, F. Ke, M. Xiao, K. Wu, Y. Kuang, H. Corke and F. Jiang, The control of ice crystal growth and effect on porous structure of konjac glucomannan-based aerogels, Int. J. Biol. Macromol., 2016, 92, 1130–1135 CrossRef CAS PubMed.
- R. Lai, Y. Liu and J. Liu, Properties of the konjac glucomannan and zein composite gel with or without freeze-thaw treatment, Food
Hydrocolloids, 2021, 117, 106700 CrossRef CAS.
- S. Charoenrein, O. Tatirat, K. Rengsutthi and M. Thongngam, Effect of konjac glucomannan on syneresis, textural properties and the microstructure of frozen rice starch gels, Carbohydr. Polym., 2011, 83, 291–296 CrossRef CAS.
- S. Zhu, J. Yu, X. Chen, Q. Zhang, X. Cai, Y. Ding, X. Zhou and S. Wang, Dual cryoprotective strategies for ice-binding and stabilizing of frozen seafood: A review, Trends Food Sci. Technol., 2021, 111, 223–232 CrossRef CAS.
- H. M. Li, S. Shen, K. J. Yu, H. B. Wang and J. Fu, Construction of porous structure-based carboxymethyl chitosan sodium alginate tea polyphenols for wound dressing, Int. J. Biol. Macromol., 2023, 233, 123404 CrossRef CAS.
- S. Jiang, L. C. Shang, H. S. Liang, B. Li and J. Li, Preparation of konjac glucomannan/xanthan gum/sodium alginate composite gel by freezing combining moisture regulation, Food Hydrocolloids, 2022, 127, 107449 Search PubMed.
- Z. Li, L. Zhang, C. Mao, Z. Song, X. Li and C. Liu, Preparation and characterization of konjac glucomannan and gum arabic composite gel, Int. J. Biol. Macromol., 2021, 183, 2121–2130 CrossRef CAS PubMed.
- A. Brodkorb, L. Egger, M. Alminger, P. Alvito, R. Assuncao, S. Ballance, T. Bohn, C. Bourlieu-Lacanal, R. Boutrou, F. Carriere, A. Clemente, M. Corredig, D. Dupont, C. Dufour, C. Edwards, M. Golding, S. Karakaya, B. Kirkhus, S. Le Feunteun, U. Lesmes, A. Macierzanka, A. R. Mackie, C. Martins, S. Marze, D. J. McClements, O. Menard, M. Minekus, R. Portmann, C. N. Santos, I. Souchon, R. P. Singh, G. E. Vegarud, M. S. J. Wickham, W. Weitschies and I. Recio, INFOGEST static in vitro simulation of gastrointestinal food digestion, Nat. Protoc., 2019, 14, 991–1014 CrossRef CAS.
- L. Chen, R. Deng, W. Yokoyama and F. Zhong, Investigation of the effect of nanocellulose on delaying the in vitro digestion of protein, lipid, and starch, Food Hydrocolloids Health, 2022, 2 DOI:10.1016/j.fhfh.2022.100098.
- S. Wang, S. Wang, L. Liu, S. Wang and L. Copeland, Structural orders of wheat starch do not determine the in vitro enzymatic digestibility, J. Agric. Food Chem., 2017, 65, 1697–1706 CrossRef CAS.
- D. Li, Z. Wu, S. Zhao, L. Wang, D. Qiao, L. Zhang and B. Zhang, Cold-chain cooked rice with different water contents: Retarded starch digestion by refrigeration, Int. J. Biol. Macromol., 2022, 199, 10–16 CrossRef CAS PubMed.
- D. Qiao, F. Xie, B. Zhang, W. Zou, S. Zhao, M. Niu, R. Lv, Q. Cheng, F. Jiang and J. Zhu, A further understanding of the multi-scale supramolecular structure and digestion rate of waxy starch, Food Hydrocolloids, 2017, 65, 24–34 CrossRef CAS.
- H. R. Kim, S. J. Choi, C. S. Park and T. W. Moon, Kinetic studies of in vitro digestion of amylosucrase-modified waxy corn starches based on branch chain length distributions, Food Hydrocolloids, 2017, 65, 46–56 CrossRef CAS.
- L. Li, H. Xu, J. Zhou, J. Yu, L. Copeland and S. Wang, Mechanisms underlying the effect of tea extracts on in vitro digestion of wheat starch, J. Agric. Food Chem., 2021, 69, 8227–8235 CrossRef CAS PubMed.
- G. M. Genevro, M. A. de Moraes and M. M. Beppu, Freezing
influence on physical properties of glucomannan hydrogels, Int. J. Biol. Macromol., 2019, 128, 401–405 CrossRef CAS.
- M. C. Gutiérrez, M. L. Ferrer and F. D. Monte, Ice-templated materials sophisticated structures exhibiting enhanced functionalities obtained after unidirectional freezing and ice-segregation-induced self-assembly, Chem. Mater., 2008, 20, 634–648 CrossRef.
- T. Köhnke, A. Lin, T. Elder, H. Theliander and A. J. Ragauskas, Nanoreinforced xylan–cellulose composite foams by freeze-casting, Green Chem., 2012, 14, 1864–1869 RSC.
- A. R. Mackie, H. Rafiee, P. Malcolm, L. Salt and G. van Aken, Specific food structures supress appetite through reduced gastric emptying rate, Am. J. Physiol.: Gastrointest. Liver Physiol., 2013, 304, 1038–1043 CrossRef PubMed.
- S. Deville, E. Saiz and A. P. Tomsia, Ice-templated porous alumina structures, Acta Mater., 2007, 55, 1965–1974 CrossRef CAS.
- A. J. Wanders, J. J. G. C. van den Borne, C. de Graaf, T. Hulshof, M. C. Jonathan, M. Kristensen, M. Mars, H. A. Schols and E. J. Feskens, Effects of dietary fibre on subjective appetite, energy intake and body weight: a systematic review of randomized controlled trials, Obes. Rev., 2011, 12, 724–739 CrossRef CAS.
|
This journal is © The Royal Society of Chemistry 2024 |