DOI:
10.1039/D3FO03506A
(Paper)
Food Funct., 2024,
15, 208-222
Tibetan tea consumption prevents obesity by modulating the cellular composition and metabolic reprogramming of white adipose tissue†
Received
22nd August 2023
, Accepted 15th November 2023
First published on 16th November 2023
Abstract
Obesity, a global health concern, is linked with numerous metabolic and inflammatory disorders. Tibetan tea, a traditional Chinese beverage rich in theabrownin, is investigated in this study for its potential anti-obesity effects. Our work demonstrates that Tibetan tea consumption in C57BL/6J mice significantly mitigates obesity-related phenotypic changes without altering energy intake. Computational prediction revealed that Tibetan tea consumption reconstructs gene expression in white adipose tissue (WAT), promoting lipid catabolism and thereby increasing energy expenditure. We also note that Tibetan tea suppresses inflammation in WAT, reducing adipocyte hyperplasia and immune cell infiltration. Furthermore, Tibetan tea induces profound metabolic reprogramming, influencing amino acid metabolic pathways, specifically enhancing glutamine synthesis, which in turn suppresses pro-inflammatory chemokine production. These findings highlight Tibetan tea as a potential candidate in obesity prevention, providing a nuanced understanding of its capacity to modulate the cellular composition and metabolic landscape of WAT.
1 Introduction
Obesity is a widespread global health concern, impacting individuals across all age groups and both genders.1,2 This health issue arises from a myriad of factors, encompassing dietary habits, lifestyle choices, genetic predisposition, and environmental influences. A primary driver behind the surge in obesity is a disruption of energy homeostasis. This is marked by a rise in energy consumption and a decrease in energy expenditure, culminating in an undue accumulation of fat. The primary metabolic organ in animals for storing energy is the WAT. In conditions of excess dietary intake, adipocytes safeguard energy as triacyl glycerides, which later serve as fuel for peripheral organs during calorie deficiency via the release of fatty acids.3 However, persistent calorie overload prompts WAT expansion, attributed to adipocyte hyperplasia and hypertrophy, leading to increased body weight and manifesting as obesity.3 As there is a ceiling to the amount of fat and cell size adipocytes can manage, prolonged caloric excess can induce chronic stress responses and adipocyte apoptosis. This in turn instigates inflammation within the WAT, setting the stage for a systemic low-grade inflammatory condition, which is linked to ailments like cardiovascular disease and type 2 diabetes.3,4 Therefore, devising effective strategies to curb and address obesity is paramount.
While diet modifications and regular physical activity are the go-to approaches to tackle obesity, sustaining such a lifestyle over extended periods remains a challenge for many. The FDA has given the green light to a range of anti-obesity medications, including phentermine and orlistat, but their usage is not devoid of health-related side effects.5 Phentermine, an oral noradrenergic agonist, works by dampening one's appetite; however, it is frequently linked to side effects like dry mouth and insomnia.6 Orlistat, on the other hand, acts by inhibiting gastrointestinal and pancreatic lipases, curtailing fatty acid absorption.7 Yet, its use can lead to steatorrhea and digestive issues. Interestingly, numerous studies suggest the potential of certain natural products in aiding weight loss, with the advantage of minimal to no side effects.8–11
Tea, a traditional Chinese beverage, has been highlighted for its beneficial impact on obesity and metabolic disorders. Previous studies have shown that the consumption of tea, particularly rich in polyphenols like epigallocatechin gallate (EGCG), modulates energy balance, body weight, and glucose metabolism.12 However, high doses of EGCG, an isolated substance found in tea, have been reported to exhibit cytotoxic and hepatotoxic effects in rodents.13,14 This raises the question of whether crude extracts from tea, abundant in bioactive compounds, might represent a more effective and safer anti-obesity strategy.
Tibetan tea, a traditional Chinese beverage consumed for over a millennium, has been regarded as safe and valued for its potential obesity-preventive properties. Rich in theabrownin, one of the most active pigments in Tibetan tea, it is a promising candidate for obesity management.15–17 However, the exact anti-obesity mechanisms of Tibetan tea remain unclear, necessitating detailed investigation into its potential effects on obesity-related phenotypes, cellular composition, and the metabolic landscape of WAT.
In this study, we use a combination of newly generated and published omics data to illuminate the potential anti-obesity effects of Tibetan tea. This work not only fills the knowledge gap concerning the molecular mechanisms underlying Tibetan tea's anti-obesity effects but also provides a novel perspective on the role of dietary factors in obesity prevention and management. In light of current obesity trends and their associated health consequences, our findings could have significant implications for the development of effective and safe anti-obesity strategies, contributing to public health initiatives aimed at reducing obesity and its related disorders worldwide.
2 Materials and methods
2.1 Tibetan tea water extract
In the present study, premium-grade Tibetan tea, characterized by its stability, was procured from Sichuan Yingtai Tea Industry Co., Ltd. The tea, prepared in October 2021, was initially subjected to an extraction process.18 This process involved steeping the tea in ultrapure water (1
:
15, w/v) at a temperature of 75 °C for a duration of 45 minutes. The resultant infusion was subsequently filtered, and ultrapure water was incorporated once again (1
:
10, w/v). This mixture was subjected to a second extraction phase at 75 °C for a period of 30 minutes. The extracts from both phases were combined, subjected to lyophilization, and securely stored at −80 °C for future use.
2.2 Detection of phytochemical composition
Detection was performed using our previously established method.16 Specifically, polyphenolic compounds in an aqueous extract of Tibetan tea were measured using the Folin–Ciocalteu colorimetric approach, with gallic acid as the comparison standard.19 The aluminum trichloride–sodium nitrite colorimetric assay, with rutin as the comparative standard, was applied to determine the total flavonoid content.20 The phenol–sulfuric acid technique, referenced against glucose, was used to measure soluble sugar levels, while the Coomassie-Brilliant Blue protein assay kit, calibrated with bovine serum albumin, was utilized to examine the soluble protein content.21,22 Total free amino acids were determined using the ninhydrin colorimetric assay, using glutamic acid as the reference standard. The total pigment content, which includes theaflavins (TFs), thearubigins (TRs), and theabrownins (TBs), was analyzed using a system analysis approach.23,24
2.3 Measurement of catechins, caffeine, and gallic acid
An Agilent HPLC system, with an attached ZORBAX SB-C18 column (250 mm × 4.6 mm, 5 μm), was used for quantification using high-performance liquid chromatography with diode array detection (HPLC-DAD) under the specified chromatographic conditions.16 Mobile phase A was made up of 0.2% (v/v) acetic acid in water, while mobile phase B included methanol. The gradient elution was carried out in a specific sequence, and the flow rate was set at 0.9 mL min−1. The column temperature and detection wavelength were kept constant at 35 °C and 278 nm respectively, and the injection volume was fixed at 10 μL. The identification of chemical compounds was based on matching the peak retention times to those of the standards in the tea samples.
2.4 Experimental design
Male C57BL/6 J mice, aged 6 weeks, were obtained from Beijing Vital River Laboratory Animal Technology Co., Ltd. These mice were accommodated in a specific pathogen-free (SPF) setting with unfettered access to food and water, under a 12-hour light–dark cycle. Post a two-week acclimatization phase, the mice were randomly allocated to four groups: the CW group (chow diet and drinking water), the CT group chow diet with drinking 0.25% (w/v) Tibetan tea (an equivalent dose to human consumption of 7 g/60 kg day−1, a concentration based on previous crude extracts), the HW group (high-fat diet with drinking water) and the HT group (high-fat diet with drinking Tibetan tea). After 10 weeks, mice were anesthetized and euthanized by CO2 inhalation in cohorts. An immediate collection of samples was undertaken for subsequent analysis. All experiments were conducted in strict accordance with the guidelines established by the Experimental Animal Ethical Review Committee and the Welfare Committee of Sichuan Agricultural University and received their approval (approval no.: 20220205). Study participants were fully informed regarding the purposes of the study and consent was obtained.
2.6 Histological analysis
The hematoxylin and eosin (H&E) staining process was carried out on 5 mm thick tissue sections prepared from paraffin-embedded samples.25 These sections were dried at 56 °C for a day, and then stored at room temperature. They were deparaffinized in xylene, rehydrated using a series of alcohol baths, and rinsed in distilled water before being stained with hematoxylin. After washing and treating with 1% acid alcohol, they were counterstained with eosin, dehydrated, cleared with alcohols and xylene, and mounted. Images were acquired with a microscope, and the diameters of WAT were measured using ImageJ software (v 1.53t).
2.7 Untargeted metabolomics
Samples conserved at −80 °C were thawed on ice and homogenized using a 30 Hz grinder for 20 seconds. An internal standard-containing solution of 400 μL (methanol
:
water = 7
:
3, v/v) was added to the 20 mg ground sample, followed by shaking at 1500 rpm for 5 minutes. The sample was then placed on ice for 15 minutes and centrifuged at 12
000 rpm for 10 minutes (4 °C). A 300 μL supernatant was isolated and frozen at −20 °C for 30 minutes, followed by centrifugation at 12
000 rpm for 3 minutes (4 °C). Aliquots of 200 μL supernatant were transferred for LC-MS analysis, executed by the LC-MS system guidelines. The analytical conditions included: UPLC with a Waters ACQUITY UPLC HSS T3 C18 column (1.8 μm, 2.1 mm × 100 mm); a column temperature of 40 °C; a flow rate of 0.4 mL min−1; a 2 μL injection volume; and a solvent system of water (0.1% formic acid)
:
acetonitrile (0.1% formic acid). The column was initially eluted with 5% mobile phase B (0.1% formic acid in acetonitrile), and then increased to 90% mobile phase B over 11 minutes, held for 1 minute, returned to 5% mobile phase B within 0.1 minutes, and held for 1.9 minutes.
2.8 RNA extraction
Total RNA was isolated from the tissues utilizing TRIzol® Reagent, adhering to the manufacturer's guidelines. The quality and quantity of the extracted RNA were determined using the 5300 Bioanalyser (Agilent) and an ND-2000 spectrophotometer (NanoDrop Technologies), respectively. Only RNA samples of high quality (OD260/280 = 1.8–2.2, OD260/230 ≥ 2.0, RIN ≥ 6.5, 28S:18S ≥ 1.0, >1μg) were selected for further sequencing library construction.
2.9 Library preparation and sequencing
Library preparation and sequencing.
All procedures related to RNA purification, reverse transcription, library construction, and sequencing were conducted at Shanghai Majorbio Bio-pharm Biotechnology Co., Ltd (Shanghai, China), in compliance with the manufacturer's instructions (Illumina, San Diego, CA). The WAT RNA-seq transcriptome library was constructed utilizing the Illumina® Stranded mRNA Prep, Ligation kit (Illumina, San Diego, CA), employing 1 μg of total RNA. Briefly, messenger RNA was initially isolated using an oligo(dT) bead-based polyA selection method, followed by fragmentation using a fragmentation buffer. Subsequently, a SuperScript double-stranded cDNA synthesis kit (Invitrogen, CA) and random hexamer primers (Illumina) were utilized to generate double-stranded cDNA. This synthesized cDNA underwent end-repair, phosphorylation, and ‘A’ base addition according to the protocol outlined by Illumina for library construction. Libraries were size selected for 300 bp cDNA target fragments using 2% Low Range Ultra Agarose, followed by PCR amplification with Phusion DNA polymerase (NEB) for 15 cycles. Upon quantification with Qubit 4.0, the resulting paired-end RNA-seq sequencing library was sequenced on the NovaSeq 6000 platform, with a read length of 2 × 150 bp.
2.10 RNA-Seq data processing
The raw sequence data underwent quality control via fastp (v0.23.3).26 Subsequent alignment of reads to the GENCODE GRCm39 reference genome (vM32) was performed by HISAT2 (v2.2.1).27 Gene counts were analyzed using featureCounts (v2.0.6),28 while the differential gene expression was detected using DESeq2 (v1.40.1).29 The differentially expressed genes were characterized by a threshold set at adjusted P-value ≤ 0.05 and |log2 FoldChange| ≥ 0.5. The RNA-seq sequencing data generated in this study are accessible under the GEO: accession number GSE235473. The clusterProfiler R package (version 4.8.1)30 was utilized for the GO biological process and pathway enrichment analyses.
2.11 Analysis of the transcription factor–target gene network
Gene regulatory networks were inferred from gene expression matrices in WAT and differentially expressed genes (DEGs) in obesity or Tibetan tea consumption using the GENIE3 R package (version 1.22.0).31 The RcisTarget R package (version 1.20.0), based on the mm9 RcisTarget database, was utilized to identify enriched transcription factor-binding motifs and to predict potential target genes (regulons). Finally, Cytoscape software (version 3.10.0) was used to visualize the transcription factor–target gene network with high-confidence annotations.32
2.12 scRNA-seq data processing
For the integration of bulk RNA-seq and scRNA-seq data, we sourced scRNA-seq data of mouse WAT from the Gene Expression Omnibus (GSE176171) and retrieved inguinal adipose tissue data aligning with our bulk RNA-seq for further analysis. Nuclei exhibiting over 7000 genes or a mitochondrial genes ratio greater than 1% were excluded from the analysis. Normalization, integration, dimensionality reduction, clustering, and differential gene expression analysis followed the pipeline outlined by the Seurat R package (version 4.3.0.1).33
2.13 Phenotype-associated subpopulation identification
We used SCISSOR to associate phenotypic data from our bulk RNA-seq experiments with public scRNA-seq data.34 SCISSOR was performed on both HW vs. CW and HT vs. HW, conducting a grid search for the α parameter with 2−i/2 (where i ∈ [24, 23, 22, …, 2]) and a cutoff threshold of 0.3. For each scRNA-seq sample, we computed the fraction of Scissor+ cells (positively associated with obesity or Tibetan tea consumption), Scissor− cells (negatively associated), and background cells.
2.14 Deconvolution using CIBERSORTx
With public scRNA-seq from mouse WAT,35 we established gene expression signatures for each unique cell type. We identified top-ranking overexpressed genes (adjusted P-value ≤0.05, average log2 fold change ≥ 0.5) using Seurat's FindMarkers function for each cell type. These genes were then used in CIBERSORTx to calculate the cellular composition of bulk RNA-seq samples.36
2.15 Gene set variation analysis (GSVA) of KEGG metabolic pathways
Pathway analyses focused on KEGG pathways described in the molecular signature database. Metabolic pathway activities were also evaluated using a curated dataset. The GSVA was then applied to assign pathway activity estimates to individual samples, employing standard settings via the GSVA package (version 1.22.4).37
2.16 Statistical analyses
All statistical analyses were executed in R 4.3.1 software. Data are represented as mean ± SEM unless otherwise specified. Differences between treatment and control groups were evaluated using a two-tailed Student's t-test, assuming an equal variance. *, **, and *** represent P ≤ 0.05, P ≤ 0.01, and P ≤ 0.001, respectively, which represent statistically significant values, while NS represents insignificant values.
3 Results
3.1 Main chemical compounds in the aqueous extract from Tibetan tea
In the aqueous extract of Tibetan tea powders, we identified the main chemical compounds (Fig. 1a). Tea polyphenols emerged as the most abundant component, accounting for 34.3%, trailed by total flavonoids (23.7%), soluble sugars (18.3%), theabrownin (6.46%), soluble proteins (6.27%), total free amino acids (6.18%), thearubigins (3.14%), and theaflavins (1.68%). Delving deeper into the tea polyphenols, the major compounds were CAF (32.0%), followed by GA (22.7%), GCG (17.5%), EGC (10.1%), EGCG (5.82%), ECG (5.64%), EC (4.84%), and C (1.44%).
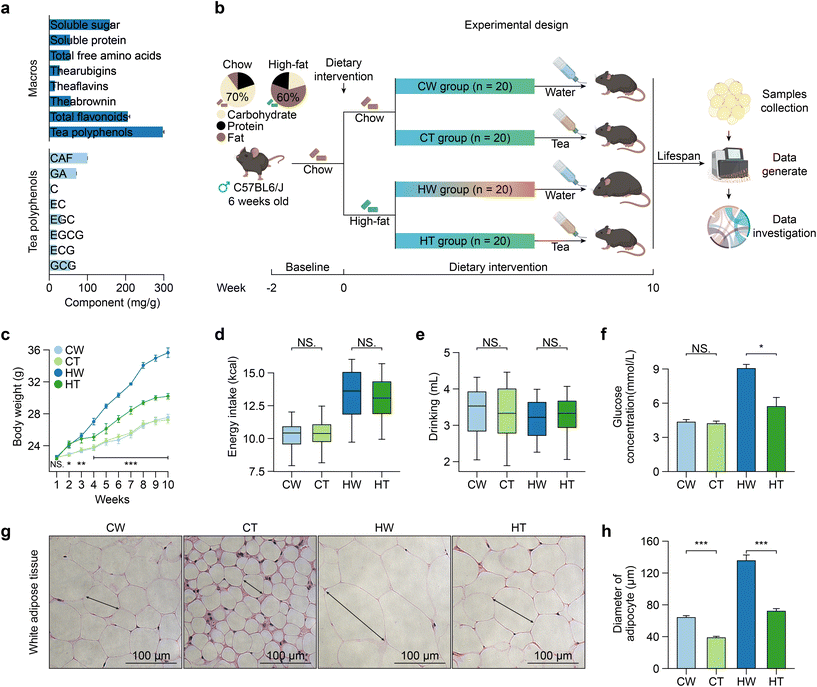 |
| Fig. 1 Tibetan tea consumption influences the body weight gain and exerted effects on obesity-associated morphological changes. (a) Profiles of chemical components in Tibetan tea water extraction. (b) A schematic diagram of mice subjected to a dietary intervention for 10 weeks starting at the age of 8 weeks. CW, chow diet with drinking water; CT, chow diet with drinking tea; HW, high-fat diet with drinking water; HT, high-fat diet with drinking tea. (c) Average body weight (n = 20 mice). (d and e) Average daily energy and water intake (n = 20 over 10 weeks). (f) Quantification of the blood glucose concentration (n = 20). (g) H&E staining of WAT. Scale bars, 100 mm. (h) Quantification of the diameter of the adipocytes (n = 10). All data are presented as mean ± SEM. A two-tailed Student's t-test was used, where * indicates P ≤ 0.05, ** signifies P ≤ 0.01, and *** represents P ≤ 0.001. | |
3.2 Tibetan tea consumption attenuates the body weight gain and alleviates obesity-related phenotypic changes
To determine whether Tibetan tea consumption can prevent obesity, we designed an animal experiment (Fig. 1b). As anticipated, mice in the HT group exhibited a significantly lower weight gain compared to the HW group, while no significant differences in weight were observed between the CW and CT groups (Fig. 1c). Furthermore, the energy intake and drinking of the mice were found to be independent of Tibetan tea consumption (Fig. 1d and e). Notably, while Tibetan tea consumption did not significantly affect the blood glucose levels under a regular diet, it did lower the levels in the context of a high-fat diet (Fig. 1f). This aligns with previous reports that dark tea can stabilize the blood glucose levels.38 Moreover, Tibetan tea consumption had profound impacts on obesity-associated tissue morphology changes. Dissection of tissues revealed decreased adipose tissue mass in HT mice, with no changes in liver mass (Fig. S1a†). It notably reduced the size of adipocytes in WAT (Fig. 1g and h). Accumulation of white fat was found in brown adipose tissue (BAT) of HW mice, and these changes were reversed after Tibetan tea consumption. Simultaneously, Tibetan tea consumption also reduced the accumulation of lipids in the liver of mice (Fig. S1b†). Our results suggest that Tibetan tea delays the onset of obesity-associated phenotypes.
3.3 Tibetan tea consumption reverses obesity-related gene expression alterations
To understand the effects of obesity and Tibetan tea on the transcriptional profiles of WAT, we generated RNA-seq atlases for CW, CT, HW, and HT mice (five replicates in each group). Following the quality control process, we secured a total of 18 satisfactory samples for the ensuing analyses. Then, we performed principal component analysis (PCA), revealing the greatest separation between the HW and other groups, moderate separation between HT and CW, and little separation between CT and CW (Fig. 2a). Next, we performed differentially expressed gene (DEG) analysis, and as a result, we identified thousands of DEGs, among which the DEGs of HW vs. CW were referred to as “Obesity DEGs”, and the DEGs of HT vs. HW and CT vs. CW were referred to “Tibetan tea consumption DEGs” (Fig. 2b and Table S1†). Specifically, obesity DEGs include a series of obesity-related genes such as Dbp, Hmga2, and Oxtr.39–41 For example, the DBP gene encodes the D site of albumin promoter (albumin D-box) binding protein. It has a role in circadian rhythm regulation, and disruptions in circadian rhythms have been implicated in metabolic disorders including obesity.
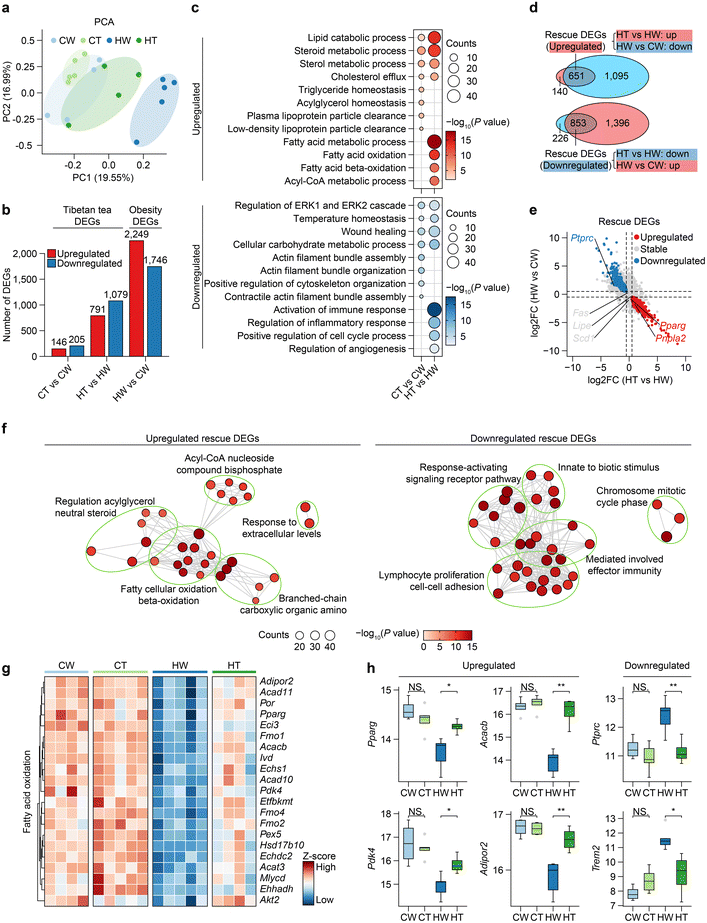 |
| Fig. 2 Impact of Tibetan tea consumption on obesity-induced alterations in WAT transcriptome. (a) Principal component analysis (PCA) of transcriptomes for WAT. (b) Bar plot indicating the number of Tibetan tea and obesity DEGs in WAT (|log2FC| ≥ 0.5, adjusted P-value ≤ 0.05). (c) Selected gene ontology terms and enriched pathways in Tibetan tea DEGs based on functional enrichment analysis. (d) Venn diagrams presenting the count of obesity, Tibetan tea, and rescue DEGs. The overlapping areas show the count of upregulated (top) and downregulated (bottom) rescue DEGs. (e) The four-quadrant plot shows the log2FC of rescue DEGs, where the marked genes are based on previously reported. (f) Gene Ontology of the upregulated or downregulated rescue DEGs. (g) Heatmap of representative fatty acid oxidation genes. (h) Boxplots of the representative gene expression. All data are presented as mean ± SEM. A two-tailed Student's t-test was used, where * indicates P ≤ 0.05, ** signifies P ≤ 0.01, and *** represents P ≤ 0.001. | |
Strikingly, HW and CW had the largest number of DEGs. HT and HW showed an intermediate effect. The number of DEGs in CT vs. CW was the lowest, indicating that Tibetan tea had little effect on gene expression in WAT under normal physiological conditions. Next, we delved into the biological consequences of obesity and DEGs related to Tibetan tea consumption, employing Gene Ontology (GO) and pathway analyses (Fig. 2c). The commonly upregulated genes in CT and HT were enriched in the lipid catabolic process, steroid metabolic process, and sterol metabolic process, which is consistent with the previously reviewed dark tea that can improve lipid metabolism.38 Notably, the triglyceride homeostasis and acylglycerol homeostasis pathway was specifically upregulated in CT, implying that Tibetan tea consumption can make the metabolic system of WAT more stable. The fatty acid oxidation pathway was specifically upregulated in HT, suggesting that it may significantly contribute to energy dissipation and a lean phenotype in mice.42 As previously reported, mice following a high-fat diet elicited an inflammatory response in WAT.43
Through an integrative comparative analysis of these DEGs, we identified certain obesity DEGs that Tibetan tea partially mitigated, hence termed “rescue DEGs” (Fig. 2d). Interestingly, the genes related to the inhibition of lipid synthesis (Fas, Scd1) were not affected by Tibetan tea consumption, but the genes related to lipolysis (Pnpla2) and fatty acid oxidation (Pparg) were upregulated in rescue DEGs (Fig. 2e), suggesting that Tibetan tea consumption may preferentially increase lipid catabolism in WAT rather than inhibit lipid synthesis to increase energy expenditure.44 Notably, the enrichment of rescue DEGs in the acyl-CoA nucleoside compound bisphosphate pathway suggests a boost in acyl-CoA degradation, a process central to mitochondrial fatty acid oxidation (Fig. 2f). Moreover, downregulated rescue DEGs were mostly associated with lymphocyte proliferation cell–cell adhesion and the response-activating signaling receptor pathway, suggesting that Tibetan tea consumption might also suppress immune cell infiltration and inflammatory signaling in WAT (Fig. 2f).
We further observed the changes in fatty acid oxidation-related genes during obesity and Tibetan tea consumption, and the results showed that fatty acid oxidation-related genes were generally downregulated during obesity and restored by Tibetan tea consumption (Fig. 2g). To be specific, Pparg plays a key role in regulating genes involved in lipid metabolism and fatty acid oxidation. Pdk4 is involved in the regulation of glucose metabolism and switches the energy source of the cell from glucose to fatty acids.45Acacb is a crucial enzyme in fatty acid synthesis, and its inhibition can increase fatty acid oxidation.46 Lastly, Adipor2 is a receptor for adiponectin, a hormone released from adipose tissue that increases fatty acid oxidation47 (Fig. 2h).
3.4 Tibetan tea consumption reconstruct transcriptional regulatory networks
To delineate the transcriptional regulatory networks implicated in obesity and Tibetan tea consumption, we predicted core transcription factors that regulate obesity and Tibetan tea consumption DEGs in WAT. Transcription factors that were dysregulated during obesity and then normalized by Tibetan tea consumption were classified as rescue transcription factors (Fig. 3a and Table S2†). Notably, Pparg, a member of the peroxisome proliferator-activated receptor (PPAR) subfamily of nuclear receptors known to be a browning indicator in mature white adipocytes and a regulator of adipogenesis-related genes,48 was downregulated during obesity and upregulated by Tibetan tea consumption (Fig. 3b). Furthermore, Spi1, a member of the E26 transformation-specific family of transcription factors involved in macrophage migration and infiltration,49 was upregulated during obesity and downregulated by Tibetan tea consumption (Fig. 3c).
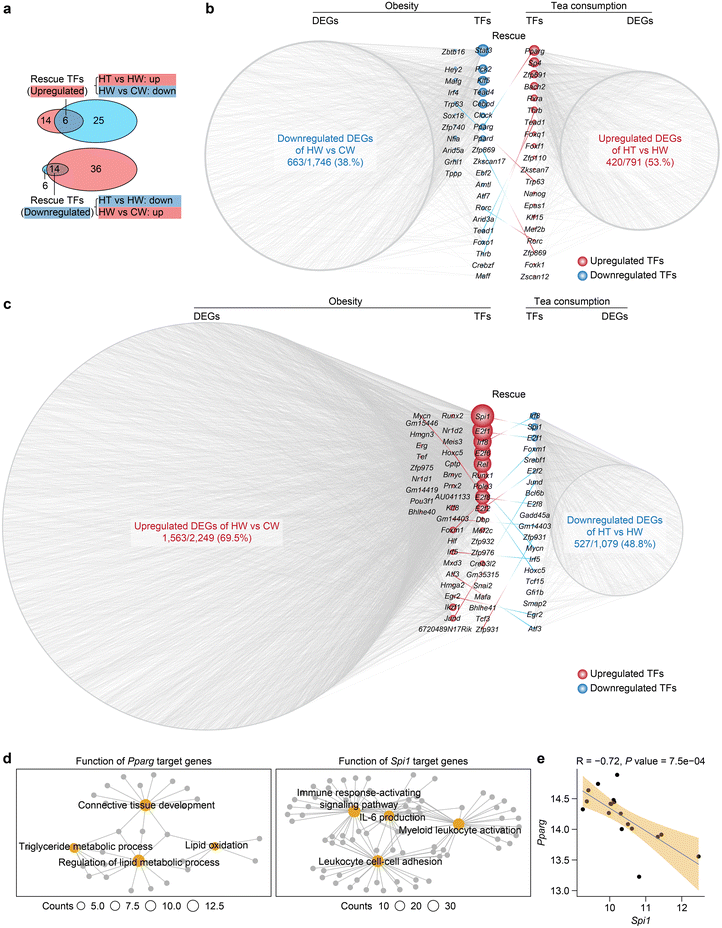 |
| Fig. 3 Alterations in core regulatory transcription factors during obesity and Tibetan tea consumption. (a) Venn diagrams presenting the count of obesity, Tibetan tea consumption, and rescue TFs. Overlapping regions show the count of downregulated or upregulated rescue TFs. (b and c) Network visualization of potential upregulated (b) and downregulated (c) rescue TFs. Internal nodes denote TFs; the gray circular edge indicates downstream target DEGs of these TFs. Node sizes correlate positively to the number of associated DEGs. The middle connecting line denotes rescue TFs. (d) Network visualization shows the enrichment results of target genes of Pparg and Spi1, respectively. (e) Pearson's correlation of the Spi1 and Pparg gene expression in WAT. | |
Further investigation of the functional enrichment of target genes elucidates the core roles of these transcription factors (Fig. 3d). Predominantly, Pparg, experiencing downregulation in obesity, has its target genes chiefly enriched in lipid metabolism pathways. This underscores the centrality of Pparg in metabolic processes, and thus its perturbation could significantly influence obesity progression. On the other hand, the target genes of Spil1, upregulated in obesity but mitigated by Tibetan tea consumption, are predominantly associated with immune cell adhesion and the production of interleukin-6 (IL-6), both of which contribute to the exacerbation of inflammatory responses. Therefore, the regulation of Spil1 may be an important component of the anti-inflammatory effects observed with Tibetan tea consumption (Fig. 3e).
These findings collectively suggest that Tibetan tea consumption reconfigures the transcriptional regulatory networks compromised by obesity. Moreover, transcription factors associated with inflammation and lipid metabolism might be co-regulated by obesity and Tibetan tea consumption.
3.5 Tibetan tea consumption reconstitutes the cellular ecosystem during obesity
To elucidate the dynamics of cellular composition changes during obesity and Tibetan tea consumption, we employed Scissor to select the cell subpopulations the most responsible for the differences in CW, HW, and HT phenotypes from single-cell RNA-seq data.34 Therefore, we first collected, reprocessed, integrated, and annotated scRNA-seq data for mouse WAT from publicly available datasets (Fig. S2a†), resulting in the WAT dataset consisting of 58
530 cells including 14
025 adipocytes, 21
947 adipose stem and progenitor cells (ASPCs), 2793 vascular cells and 19
765 immune cells from 9 samples (Fig. 4a). Comparing HW with CW, Scissor identified a total of 12
104 Scissor+ cells associated with the HW and 4268 Scissor− cells associated with the CW (Fig. S2b†). Similarly, HT compared to HW, Scissor identified a total of 7221 Scissor+ cells associated with the HT and 9409 Scissor− cells associated with the HW (Fig. 4b).
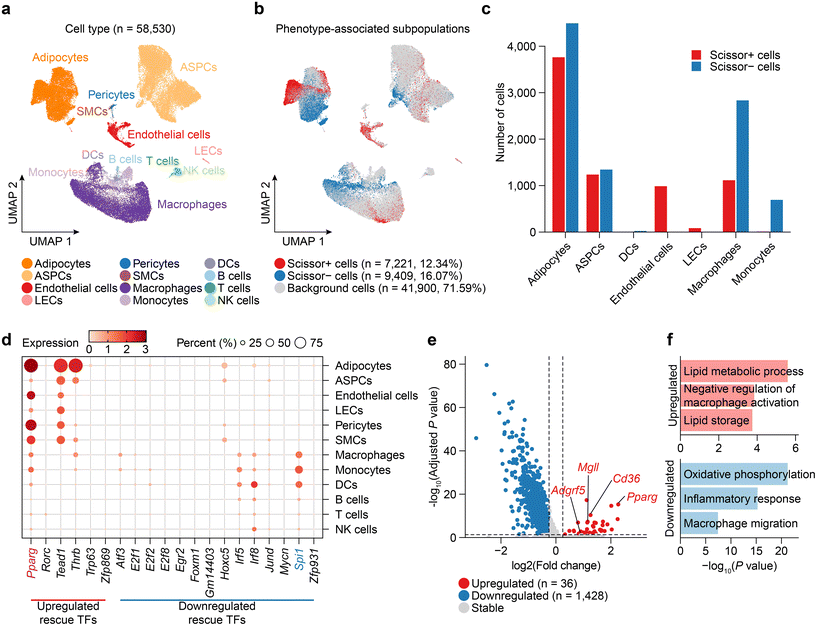 |
| Fig. 4 Changes in cell proportions during obesity and Tibetan tea consumption. (a) UMAP visualization of 58 530 cells from mouse WAT. (b) UMAP visualization was used to display the cells selected by Scissor, with the Scissor+ and Scissor− cells associated with HT and HW phenotypes represented by red and blue dots, respectively. (c) A bar plot was employed to present a detailed depiction of the phenotypic composition of these Scissor-selected cells. (d) The bubble plot shows the expression distribution of rescue TFs at the level of each cell type. (e) A volcano plot of differential gene expressions in Scissor+ endothelial cells versus Scissor-endothelial cells. (f) Enrichment bar plot of pathways in the GO biological process. | |
Next, upon comparing the changes in the cellular composition of various cell types in the HW group and the CW group, noticeable changes were observed, many of which were rescued by Tibetan tea consumption (Fig. 4c and Fig. S2c†). For instance, the proportions of adipocytes amplified during obesity were mitigated by Tibetan tea consumption, which suggests a potential role for Tibetan tea in preventing adipocyte hyperplasia, consistent with previous reports.38 In agreement with existing studies that report macrophage accumulation and associated inflammatory response in the WAT of obese mice,50 we observed increased macrophage and monocyte counts during obesity, which were counteracted by Tibetan tea consumption. Moreover, Tibetan tea consumption resulted in an elevation of endothelial cell counts, suggesting a potentially beneficial effect on endothelial cell health. This is significant, as endothelial dysfunction is a characteristic of obesity-related vascular complications,51 marked by impaired endothelial cell function, augmented oxidative stress, inflammation, and altered vasodilation.
We further observed the expression distribution of rescue TFs in various cell types in WAT and found that Pparg was not only highly expressed in adipocytes, but also widely distributed in vascular cells (endothelial cells, pericytes, and SMCs) (Fig. 4d). Then, we examined DEGs between Scissor+ cells and Scissor- cells in endothelial cells after Tibetan tea consumption (Fig. 4e and Table S3†). In endothelial cells, Pparg is upregulated after Tibetan tea consumption, which stimulates lipid and glucose utilization and exerts anti-inflammatory effects on the vascular wall and immune cells.52 In addition, multiple lipid metabolism-promoting genes (Cd63, Mgll) or anti-inflammatory genes (Adgrf5) are upregulated by Tibetan tea consumption in endothelial cells. Pathway enrichment analysis confirmed that the lipid metabolic process and negative regulation of the macrophage activation pathway are highly activated after Tibetan tea consumption to regulate the effect on excessive lipid accumulation and inflammatory response in WAT.
3.6 Metabolic reprogramming effects of Tibetan tea consumption
To investigate the main determinants of metabolic heterogeneity after Tibetan tea consumption, we first analyzed the global structure of metabolic gene expression using PCA based on the expression levels of 1754 metabolic genes. Clustering analysis revealed that the metabolic gene expression profiles of HW mice formed distinct clusters from other groups (Fig. 5a), suggesting that the expression program of metabolic genes was most severely disturbed in HW mice. Furthermore, observing the number of overlaps between rescue DEGs and metabolic genes, we found that 14.29% of the upregulated rescue DEGs were metabolic genes (Fig. 5b), and the functions of these genes were mainly enriched in amino acid-related metabolic pathways (for example, valine, leucine and isoleucine degradation; tryptophan metabolism, alanine, aspartate, and glutamate metabolism) (Fig. 5c), suggesting that Tibetan tea consumption may act through intervention in amino acid metabolic pathways to alleviate the occurrence of obesity.
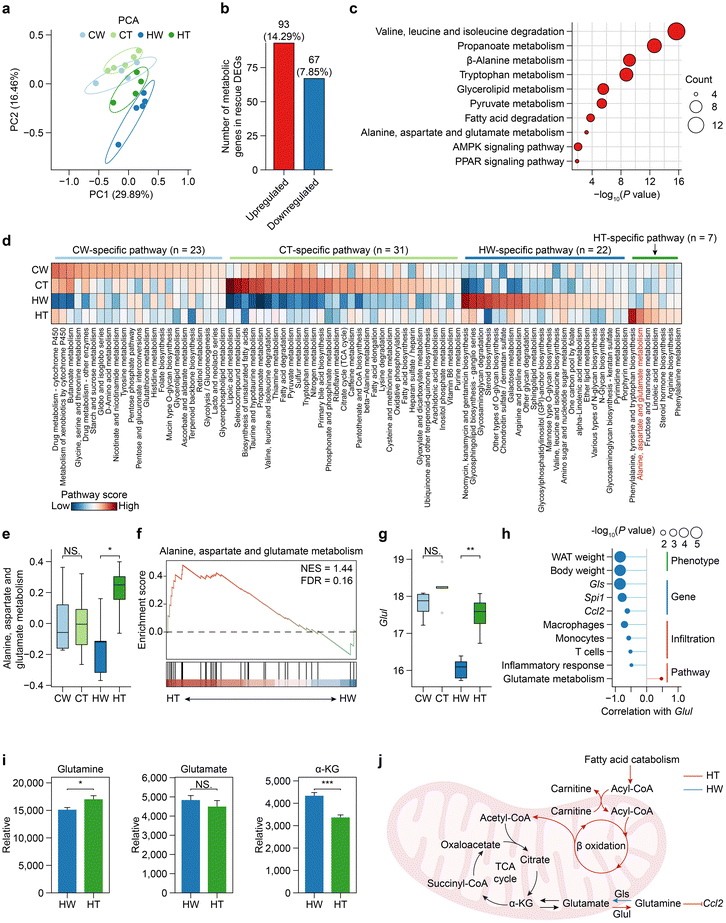 |
| Fig. 5 Tibetan tea consumption-specific metabolic reprogramming. (a) Principal component analysis (PCA) of metabolic gene expression transcriptomes for WAT. (b) A bar plot showing the overlapping numbers of rescue DEGs and metabolic genes in the WAT. (c) The bubble plots show the functional enrichment results of overlapping genes of upregulated rescue DEGs and metabolic genes. (d) Heatmap showing the metabolic pathway activity for each group. (e) A boxplot showing alanine, aspartate, and glutamate metabolism pathway activity for each group. (f) Gene set enrichment analysis for HT and HW. (g) A boxplot showing the Glul expression for each group. (h) A lollipop plot showing the correlation between the Glul expression and features of interest. (i) Bar plot shows the relative content changes of key metabolites in the glutamate metabolic pathway detected by metabolomics in the HW and HT groups. (j) A schematic diagram depicting that Tibetan tea consumption triggers metabolic reprogramming in cells, activating fatty acid oxidation and promoting glutamine synthesis and metabolism, which in turn suppresses the production of pro-inflammatory chemokine Ccl2. | |
We next sought to identify the overall features of metabolic pathway variation comprehensively and without bias among the different groups, especially between HT and HW. We quantified the metabolic activity of 83 metabolic pathways from KEGG containing at least 3 genes (Fig. 5d). HT mice had the largest number of metabolic pathways activated, and the activated pathways included many different parts of cellular metabolism such as lipoic acid metabolism, taurine and hypotaurine metabolism, and fatty acid degradation. Interestingly, we found that more than half of the metabolic pathways specifically activated in HT involve amino acid metabolism, including phenylalanine, tyrosine, and tryptophan biosynthesis, alanine, aspartate, and glutamate metabolism, and arginine biosynthesis. According to previous reports, glutamine metabolism is linked to WAT inflammation in obesity,53 so we focused on the alanine, aspartate, and glutamate metabolism pathway. The alanine, aspartate, and glutamate metabolism pathway was significantly downregulated during obesity and upregulated by Tibetan tea consumption (Fig. 5e). Gene set enrichment analysis (GSEA) also confirmed this result (Fig. 5f). Moreover, Glul, responsible for encoding glutamine synthetase and the enzyme that utilizes glutamate as a substrate and is the sole known enzyme involved in glutamine synthesis, was downregulated during obesity, and upregulated by Tibetan tea consumption (Fig. 5g).
To further determine the potential effects caused by Glul, we established the link of Glul with obesity-associated phenotypes, genes, immune infiltration, and pathway activity (Fig. 5h). Our findings revealed a significant negative correlation between Glul expression and both WAT weight and mouse body weight, consistent with previous human studies. Additionally, high Glul expression was found to inhibit the expression of Gls, an enzyme involved in glutamine hydrolysis to produce glutamate, suggesting an antagonistic relationship between Glul and Gls. Importantly, elevated Glul expression was associated with reduced immune cell infiltration and inhibited the release of Ccl2, indicating a protective role against inflammatory responses in WAT. We next validated the prediction that Tibetan tea consumption preferentially promotes the synthesis of glutamine. Metabolomics analysis shows that glutamine increased significantly after Tibetan tea consumption in WAT, while glutamate did not decrease significantly, but tended to decrease. In addition, alpha-KG also decreased significantly after Tibetan tea consumption (Fig. 5i).
Taken together, our findings, demonstrate that Tibetan tea consumption induces cellular metabolic reprogramming, activates fatty acid oxidation, and enhances glutamine synthesis, ultimately leading to the suppression of pro-inflammatory chemokine Ccl2 production (Fig. 5j). These results highlight the metabolic benefits of Tibetan tea in modulating inflammatory responses and suggest its potential as a therapeutic strategy for metabolic disorders.
4 Discussion
Tea in China has a history of over a millennium, serving as a traditional medicine and being valued for its obesity-prevention properties. It has been regarded as safe throughout generations. However, recent studies on rodents have indicated that high doses of EGCG, an isolated substance found in tea, may have cytotoxic and hepatotoxic effects.14 This suggests that using crude extracts, such as those from Tibetan tea, might be a safer option.
Our study reveals that Tibetan tea may be a promising candidate for combating obesity and its related disorders. By utilizing a combination of cellular, molecular, and bioinformatic approaches, we provide robust evidence on how Tibetan tea consumption modulates the cellular composition and reprograms the metabolic landscape of WAT, effectively mitigating obesity-related phenotypes.
Observational and interventional studies in humans and animals have highlighted the beneficial effects of tea consumption on weight regulation and obesity prevention, but the underlying molecular mechanisms remain elusive.54 Our research extends this body of knowledge by demonstrating that Tibetan tea, particularly rich in theabrownin, reduces weight gain and normalizes obesity-related phenotypic changes in a high-fat diet setting, independent of energy intake or drinking habits. Importantly, the body weight-regulating effect of Tibetan tea appears to be derived from its regulatory impact on glucose metabolism and tissue morphology, specifically reducing the size of adipocytes in WAT and minimizing ectopic lipid accumulation. Building upon these findings, it is imperative to consider the broader implications for human health. The regulation of glucose metabolism has direct ties to several metabolic conditions, including type 2 diabetes, with aberrant glucose metabolism being a recognized risk factor.55 Furthermore, reductions in ectopic lipid accumulation can mitigate risks associated with fatty liver disease, cardiovascular conditions, and even certain cancers.56,57 While our study was rooted in an animal model, the potential transference of these benefits to humans suggests that Tibetan tea might serve as a novel therapeutic or preventive dietary intervention against obesity and its array of associated comorbidities.
In addition to impacting phenotypic changes, Tibetan tea modifies gene expression profiles in WAT, shedding light on its impact at the molecular level. The upregulation of genes involved in lipid catabolism and downregulation of genes related to inflammatory signaling implies a multifaceted approach through which Tibetan tea combats obesity. The role of lipid metabolism in obesity is well documented, with studies showing that augmenting energy expenditure can effectively limit adiposity. Our findings suggest that Tibetan tea possibly enhances lipid catabolism over inhibiting lipid synthesis, thereby increasing energy expenditure. The transcription factor Pparg, which is involved in lipid metabolism and fatty acid oxidation, was found to be upregulated, providing further evidence of Tibetan tea's impact on metabolic pathways.
Furthermore, Tibetan tea consumption appears to orchestrate the cellular ecosystem during obesity, evident in the decreased adipocyte hyperplasia and immune cell infiltration in WAT. Adipocyte hyperplasia and immune cell accumulation, particularly macrophages, in WAT, are recognized as hallmarks of obesity-induced inflammation.48,58 Our observations propose a novel anti-inflammatory role for Tibetan tea, potentially exerted via the regulation of Spi1, a transcription factor involved in macrophage migration and infiltration. It is noteworthy to mention that chronic inflammation is closely intertwined with metabolic dysfunctions, and strategies that mitigate inflammation often correspondingly improve metabolic health.59,60 For instance, macrophage infiltration into adipose tissue is linked with insulin resistance, a key player in the pathogenesis of type 2 diabetes.61 By dampening the inflammatory response, particularly through the regulation of Spi1, Tibetan tea might hold promise in safeguarding against metabolic disorders. Future studies would be instrumental in understanding the depth of these associations and the broader implications of Tibetan tea's effects on metabolism.
Our study also illuminates the profound effects of Tibetan tea on metabolic reprogramming. Tibetan tea consumption seems to intervene in amino acid metabolic pathways, which is in alignment with recent reports emphasizing the relevance of amino acid metabolism in obesity and metabolic disorders.53,62 The upregulation of the alanine, aspartate, and glutamate metabolism pathway, coupled with enhanced glutamine synthesis and suppressed pro-inflammatory chemokine production, presents a compelling metabolic reprogramming strategy orchestrated by Tibetan tea consumption.
In addition, metabolic disorders, encompassing conditions such as type 2 diabetes mellitus, dyslipidemia, and non-alcoholic fatty liver disease, share intricate links with obesity.63 The fact that Tibetan tea instigates a shift in WAT gene expression, particularly promoting lipid catabolism and elevating energy expenditure, points to its potential to modulate metabolic homeostasis. Furthermore, obesity's intricate association with chronic inflammation plays a pivotal role in the pathogenesis of cardiovascular diseases, such as hypertension and atherosclerosis.64 With Tibetan tea demonstrated to stifle inflammation in WAT and restrain adipocyte hyperplasia and immune cell infiltration, it holds promise as a therapeutic strategy not only for obesity but also for associated cardiovascular complications. It is also worth contemplating Tibetan tea's broader impact on overall health, given the systemic implications of WAT inflammation. Beyond metabolic disorders and cardiovascular diseases, chronic inflammation is implicated in various malignancies, neurodegenerative disorders, and immune dysregulations.65,66 Thus, Tibetan tea's capacity to curtail inflammation might present broader health implications, meriting further in-depth investigations.
Our study has shed light on the molecular targets potentially influenced by Tibetan tea consumption, yet there is a pressing need for more comprehensive research to accurately identify these targets. These insights hold value both therapeutically and as a benchmark in the clinical oversight of obesity treatments. As we look ahead, Tibetan tea, along with other dietary interventions, might become essential in a holistic approach to obesity, pivoting from solely addressing symptoms to targeting root molecular and metabolic irregularities. To harness this potential, future research must focus on transitioning these findings from animal to human studies, determining the ideal Tibetan tea dosage, and understanding any associated side effects. This deeper exploration could provide critical markers for clinicians overseeing obesity treatment effectiveness.
5 Conclusions
Tibetan tea has been shown to have potential anti-obesity effects. Our studies on mice demonstrated that Tibetan tea consumption led to significantly lower weight gain, particularly in those on a high-fat diet, without affecting energy intake. The tea also stabilized blood glucose levels and prevented morphological changes associated with obesity, such as adipose tissue accumulation. At the molecular level, Tibetan tea reversed many obesity-related gene expression changes, particularly in pathways associated with lipid metabolism and inflammation. Additionally, this tea reshaped the cellular ecosystems of white adipose tissue, promoting beneficial changes like reducing adipocyte hyperplasia and macrophage infiltration while enhancing endothelial cell counts. The metabolic reprogramming effects of the tea were profound, with clear indications of intervention in amino acid metabolic pathways, especially in alanine, aspartate, and glutamate metabolism, which are known to be linked to obesity-associated inflammation. These findings underscore the potential therapeutic benefits of Tibetan tea in managing and possibly preventing obesity and its related complications.
Author contributions
Songqi Duan: conceptualization, investigation, methodology, software, visualization, and writing – original draft. Hongyu Li: investigation, validation, and methodology. Ziqi Wang: validation, formal analysis, and writing – review & editing. Junqi Li: data curation and formal analysis. Weimin Huang: data curation and formal analysis. Zhengfeng Fang: project administration. Cheng Li: resources. Zhen Zeng: supervision and project administration. Baofa Sun: supervision. Yuntao Liu: conceptualization, supervision, project administration, funding acquisition, and writing – review & editing.
Conflicts of interest
The authors declare no conflict of interest in regard to this manuscript.
Acknowledgements
This work was supported by the Ya'an Science and Technology Program Project (202110626036 and 22SXHZ0042). We sincerely thank Wuhan Metware Biotechnology Co., Ltd and Jian Luo for their technical support. The calculations in this study were performed on the Siyuan-1 cluster, facilitated by the Center for High-Performance Computing at Shanghai Jiao Tong University.
References
- Y. C. Chooi, C. Ding and F. Magkos, The epidemiology of obesity, Metabolism, 2019, 92, 6–10 CrossRef CAS.
- S. P. Bapat, C. Whitty, C. T. Mowery, Y. Liang, A. Yoo, Z. Jiang, M. C. Peters, L. Zhang, I. Vogel, C. Zhou, V. Q. Nguyen, Z. Li, C. Chang, W. S. Zhu, A. T. Hastie, H. He, X. Ren, W. Qiu, S. G. Gayer, C. Liu, E. J. Choi, M. Fassett, J. N. Cohen, J. L. Sturgill, L. E. Crotty Alexander, J. M. Suh, C. Liddle, A. R. Atkins, R. T. Yu, M. Downes, S. Liu, B. S. Nikolajczyk, I.-K. Lee, E. Guttman-Yassky, K. M. Ansel, P. G. Woodruff, J. V. Fahy, D. Sheppard, R. L. Gallo, C. J. Ye, R. M. Evans, Y. Zheng and A. Marson, Obesity alters pathology and treatment response in inflammatory disease, Nature, 2022, 604, 337–342 CrossRef CAS.
- A. Christ, M. Lauterbach and E. Latz, Western Diet and the Immune System: An inflammatory connection, Immunity, 2019, 51, 794–811 CrossRef CAS.
- M. Roden and G. I. Shulman, The integrative biology of type 2 diabetes, Nature, 2019, 576, 51–60 CrossRef CAS.
- E. Pilitsi, O. M. Farr, S. A. Polyzos, N. Perakakis, E. Nolen-Doerr, A.-E. Papathanasiou and C. S. Mantzoros, Pharmacotherapy of obesity: Available medications and drugs under investigation, Metab., Clin. Exp., 2019, 92, 170–192 CrossRef CAS.
- K. K. Kim, H.-J. Cho, H.-C. Kang, B.-B. Youn and K.-R. Lee, Effects on Weight Reduction and Safety of Short-Term Phentermine Administration in Korean Obese People, Yonsei Med. J., 2006, 47, 614–625 CrossRef CAS.
- J. S. Torgerson, J. Hauptman, M. N. Boldrin and L. Sjöström, XENical in the Prevention of Diabetes in Obese Subjects (XENDOS) study: A randomized study of orlistat as an adjunct to lifestyle changes for the prevention of type 2 diabetes in obese patients, Diabetes Care, 2004, 27, 155–161 CrossRef CAS.
- J. Xu, M. Li, Y. Zhang, S. Chu, Y. Huo, J. Zhao and C. Wan, Huangjinya Black Tea alleviates obesity and insulin resistance via modulating fecal metabolome in high-fat diet-fed mice, Mol. Nutr. Food Res., 2020, 64, 2000353 CrossRef CAS.
- L. Trigueros, S. Peña, A. V. Ugidos, E. Sayas-Barberá, J. A. Pérez-Álvarez and E. Sendra, Food Ingredients as Anti-Obesity Agents: A Review, Crit. Rev. Food Sci. Nutr., 2013, 53, 929–942 CrossRef CAS.
- J.-J. Li, C. J. Huang and D. Xie, Anti-obesity effects of conjugated linoleic acid, docosahexaenoic acid, and eicosapentaenoic acid, Mol. Nutr. Food Res., 2008, 52, 631–645 CrossRef CAS PubMed.
- N. Xu, J. Chu, M. Wang, L. Chen, L. Zhang, Z. Xie, J. Zhang, C.-T. Ho, D. Li and X. Wan, Large Yellow Tea attenuates macrophage-related chronic inflammation and metabolic syndrome in high-fat diet treated mice, J. Agric. Food Chem., 2018, 66, 3823–3832 CrossRef CAS PubMed.
- X.-Y. Xu, C.-N. Zhao, B.-Y. Li, G.-Y. Tang, A. Shang, R.-Y. Gan, Y.-B. Feng and H.-B. Li, Effects and mechanisms of tea on obesity, Crit. Rev. Food Sci. Nutr., 2021, 1–18 Search PubMed.
- J. D. Lambert, S. Sang and C. S. Yang, Possible controversy over dietary polyphenols: Benefits vs. risks, Chem. Res. Toxicol., 2007, 20, 583–585 Search PubMed.
- R. A. Isbrucker, J. A. Edwards, E. Wolz, A. Davidovich and J. Bausch, Safety studies on epigallocatechin gallate (EGCG) preparations. Part 2: Dermal, acute and short-term toxicity studies, Food Chem. Toxicol., 2006, 44, 636–650 CrossRef CAS.
- Y. Yuan, J. He, M. Tang, H. Chen, T. Wei, B. Zhang, D. Liang and X. Nie, Preventive effect of Ya'an Tibetan tea on obesity in rats fed with a hypercaloric high-fat diet revealed by gut microbiology and metabolomics studies, Food Res. Int., 2023, 165, 112520 CrossRef CAS.
- Y. Liu, W. Huang, C. Zhang, C. Li, Z. Fang, Z. Zeng, B. Hu, H. Chen, W. Wu, T. Wang and X. Lan, Targeted and untargeted metabolomic analyses and biological activity of Tibetan tea, Food Chem., 2022, 384, 132517 CrossRef CAS.
- Y. Tan, M. Li, K. Kong, Y. Xie, Z. Zeng, Z. Fang, C. Li, B. Hu, X. Hu, C. Wang, S. Chen, W. Wu, X. Lan and Y. Liu, In vitro simulated digestion of and microbial characteristics in colonic fermentation of polysaccharides from four varieties of Tibetan tea, Food Res. Int., 2023, 163, 112255 CrossRef CAS PubMed.
- S. Hu, Y. Chen, S. Zhao, K. Sun, L. Luo and L. Zeng, Ripened Pu-Erh tea improved the enterohepatic circulation in a circadian rhythm disorder mice model, J. Agric. Food Chem., 2021, 69, 13533–13545 CrossRef CAS.
- S. Pérez-Burillo, R. Giménez, J. A. Rufián-Henares and S. Pastoriza, Effect of brewing time and temperature on antioxidant capacity and phenols of white tea: Relationship with sensory properties, Food Chem., 2018, 248, 111–118 CrossRef PubMed.
- Y. Liu, Y. Luo, L. Zhang, L. Luo, T. Xu, J. Wang, M. Ma and L. Zeng, Chemical composition, sensory qualities, and pharmacological properties of primary leaf hawk tea as affected using different processing methods, Food Biosci., 2020, 36, 100618 CrossRef CAS.
- Y. Zhao, W. Lai, A. Xu, J. Jin, Y. Wang and P. Xu, Characterizing relationships among chemicals, sensory attributes and in vitro bioactivities of black tea made from an anthocyanins-enriched tea cultivar, LWT, 2020, 132, 109814 CrossRef CAS.
- Y. Liu, Y. Li, Y. Ke, C. Li, Z. Zhang, Y. Wu, B. Hu, A. Liu, Q. Luo and W. Wu, In vitro saliva–gastrointestinal digestion and fecal fermentation of Oudemansiella radicata polysaccharides reveal its digestion profile and effect on the modulation of the gut microbiota, Carbohydr. Polym., 2021, 251, 117041 CrossRef CAS PubMed.
- F. Qu, X. Zhu, Z. Ai, Y. Ai, F. Qiu and D. Ni, Effect of different drying methods on the sensory quality and chemical components of black tea, LWT, 2019, 99, 112–118 CrossRef CAS.
- E. a. H. Roberts and R. F. Smith, Spectrophotometric measurements of theaflavins and thearubigins in black tea liquors in assessments of quality in teas, Analyst, 1961, 86, 94–98 RSC.
- A. Kharitonenkov, T. L. Shiyanova, A. Koester, A. M. Ford, R. Micanovic, E. J. Galbreath, G. E. Sandusky, L. J. Hammond, J. S. Moyers, R. A. Owens, J. Gromada, J. T. Brozinick, E. D. Hawkins, V. J. Wroblewski, D.-S. Li, F. Mehrbod, S. R. Jaskunas and A. B. Shanafelt, FGF-21 as a novel metabolic regulator, J. Clin. Invest., 2005, 115, 1627–1635 CrossRef CAS PubMed.
- S. Chen, Ultrafast one-pass FASTQ data preprocessing, quality control, and deduplication using fastp, iMeta, 2023, 2, e107 CrossRef.
- D. Kim, J. M. Paggi, C. Park, C. Bennett and S. L. Salzberg, Graph-based genome alignment and genotyping with HISAT2 and HISAT-genotype, Nat. Biotechnol., 2019, 37, 907–915 CrossRef CAS PubMed.
- Y. Liao, G. K. Smyth and W. Shi, featureCounts: An efficient general purpose program for assigning sequence reads to genomic features, Bioinformatics, 2014, 30, 923–930 CrossRef CAS.
- M. I. Love, W. Huber and S. Anders, Moderated estimation of fold change and dispersion for RNA-seq data with DESeq2, Genome Biol., 2014, 15, 550 CrossRef PubMed.
- T. Wu, E. Hu, S. Xu, M. Chen, P. Guo, Z. Dai, T. Feng, L. Zhou, W. Tang, L. Zhan, X. Fu, S. Liu, X. Bo and G. Yu, clusterProfiler 4.0: A universal enrichment tool for interpreting omics data, Innovation, 2021, 2, 100141 CAS.
- V. A. Huynh-Thu, A. Irrthum, L. Wehenkel and P. Geurts, Inferring regulatory networks from expression data using tree-based methods, PLoS One, 2010, 5, e12776 CrossRef.
- P. Shannon, A. Markiel, O. Ozier, N. S. Baliga, J. T. Wang, D. Ramage, N. Amin, B. Schwikowski and T. Ideker, Cytoscape: A software environment for integrated models of biomolecular interaction networks, Genome Res., 2003, 13, 2498–2504 CrossRef CAS PubMed.
- Y. Hao, S. Hao, E. Andersen-Nissen, W. M. Mauck, S. Zheng, A. Butler, M. J. Lee, A. J. Wilk, C. Darby, M. Zager, P. Hoffman, M. Stoeckius, E. Papalexi, E. P. Mimitou, J. Jain, A. Srivastava, T. Stuart, L. M. Fleming, B. Yeung, A. J. Rogers, J. M. McElrath, C. A. Blish, R. Gottardo, P. Smibert and R. Satija, Integrated analysis of multimodal single-cell data, Cell, 2021, 184, 3573–3587 CrossRef CAS PubMed.
- D. Sun, X. Guan, A. E. Moran, L.-Y. Wu, D. Z. Qian, P. Schedin, M.-S. Dai, A. V. Danilov, J. J. Alumkal, A. C. Adey, P. T. Spellman and Z. Xia, Identifying phenotype-associated subpopulations by integrating bulk and single-cell sequencing data, Nat. Biotechnol., 2022, 40, 527–538 CrossRef CAS PubMed.
- M. P. Emont, C. Jacobs, A. L. Essene, D. Pant, D. Tenen, G. Colleluori, A. Di Vincenzo, A. M. Jørgensen, H. Dashti, A. Stefek, E. McGonagle, S. Strobel, S. Laber, S. Agrawal, G. P. Westcott, A. Kar, M. L. Veregge, A. Gulko, H. Srinivasan, Z. Kramer, E. De Filippis, E. Merkel, J. Ducie, C. G. Boyd, W. Gourash, A. Courcoulas, S. J. Lin, B. T. Lee, D. Morris, A. Tobias, A. V. Khera, M. Claussnitzer, T. H. Pers, A. Giordano, O. Ashenberg, A. Regev, L. T. Tsai and E. D. Rosen, A single-cell atlas of human and mouse white adipose tissue, Nature, 2022, 603, 926–933 CrossRef CAS.
- A. M. Newman, C. B. Steen, C. L. Liu, A. J. Gentles, A. A. Chaudhuri, F. Scherer, M. S. Khodadoust, M. S. Esfahani, B.
A. Luca, D. Steiner, M. Diehn and A. A. Alizadeh, Determining cell type abundance and expression from bulk tissues with digital cytometry, Nat. Biotechnol., 2019, 37, 773–782 CrossRef CAS PubMed.
- S. Hänzelmann, R. Castelo and J. Guinney, GSVA: Gene set variation analysis for microarray and RNA-Seq data, BMC Bioinf., 2013, 14, 7 CrossRef.
- F.-J. Lin, X.-L. Wei, H.-Y. Liu, H. Li, Y. Xia, D.-T. Wu, P.-Z. Zhang, G. R. Gandhi, H.-B. Li and R.-Y. Gan, State-of-the-art review of dark tea: From chemistry to health benefits, Trends Food Sci. Technol., 2021, 109, 126–138 CrossRef CAS.
- M. Manikkam, R. Tracey, C. Guerrero-Bosagna and M. K. Skinner, Plastics derived endocrine disruptors (BPA, DEHP and DBP) induce epigenetic transgenerational inheritance of obesity, reproductive disease and sperm epimutations, plos One, 2013, 8, e55387 CrossRef CAS.
- Y. Xi, W. Shen, L. Ma, M. Zhao, J. Zheng, S. Bu, S. Hino and M. Nakao, HMGA2 promotes adipogenesis by activating C/EBPβ-mediated expression of PPARγ, Biochem. Biophys. Res. Commun., 2016, 472, 617–623 CrossRef CAS.
- Y. Takayanagi, Y. Kasahara, T. Onaka, N. Takahashi, T. Kawada and K. Nishimori, Oxytocin receptor-deficient mice developed late-onset obesity, NeuroReport, 2008, 19, 951 CrossRef CAS PubMed.
- P. Morigny, J. Boucher, P. Arner and D. Langin, Lipid and glucose metabolism in white adipocytes: Pathways, dysfunction and therapeutics, Nat. Rev. Endocrinol., 2021, 17, 276–295 CrossRef CAS.
- S. M. Reilly and A. R. Saltiel, Adapting to obesity with adipose tissue inflammation, Nat. Rev. Endocrinol., 2017, 13, 633–643 CrossRef CAS PubMed.
- M. Lehrke and M. A. Lazar, The many faces of PPARγ, Cell, 2005, 123, 993–999 CrossRef CAS PubMed.
- V. Barquissau, D. Beuzelin, D. F. Pisani, G. E. Beranger, A. Mairal, A. Montagner, B. Roussel, G. Tavernier, M.-A. Marques, C. Moro, H. Guillou, E.-Z. Amri and D. Langin, White-to-brite conversion in human adipocytes promotes metabolic reprogramming towards fatty acid anabolic and catabolic pathways, Mol. Metab., 2016, 5, 352–365 CrossRef CAS PubMed.
- L. Ma, A. K. Mondal, M. Murea, N. K. Sharma, A. Tönjes, K. A. Langberg, S. K. Das, P. W. Franks, P. Kovacs, P. A. Antinozzi, M. Stumvoll, J. S. Parks, S. C. Elbein and B. I. Freedman, The effect of ACACB cis-variants on gene expression and metabolic traits, PLoS One, 2011, 6, e23860 CrossRef CAS PubMed.
- T. Yamauchi, J. Kamon, Y. Minokoshi, Y. Ito, H. Waki, S. Uchida, S. Yamashita, M. Noda, S. Kita, K. Ueki, K. Eto, Y. Akanuma, P. Froguel, F. Foufelle, P. Ferre, D. Carling, S. Kimura, R. Nagai, B. B. Kahn and T. Kadowaki, Adiponectin stimulates glucose utilization and fatty-acid oxidation by activating AMP-activated protein kinase, Nat. Med., 2002, 8, 1288–1295 CrossRef CAS.
- B. Niemann, S. Haufs-Brusberg, L. Puetz, M. Feickert, M. Y. Jaeckstein, A. Hoffmann, J. Zurkovic, M. Heine, E.-M. Trautmann, C. E. Müller, A. Tönjes, C. Schlein, A. Jafari, H. K. Eltzschig, T. Gnad, M. Blüher, N. Krahmer, P. Kovacs, J. Heeren and A. Pfeifer, Apoptotic brown adipocytes enhance energy expenditure via extracellular inosine, Nature, 2022, 609, 361–368 CrossRef CAS.
- A. Zakrzewska, C. Cui, O. W. Stockhammer, E. L. Benard, H. P. Spaink and A. H. Meijer, Macrophage-specific gene functions in Spi1-directed innate immunity, Blood, 2010, 116, e1–e11 CrossRef CAS PubMed.
- A. Kriebs, Accumulation of macrophages in adipose tissue, Nat. Rev. Endocrinol., 2021, 17, 4–4 CrossRef.
- M. Graupera and M. Claret, Endothelial cells: New players in obesity and related metabolic disorders, Trends Endocrinol. Metab., 2018, 29, 781–794 CrossRef CAS PubMed.
- D. Montaigne, L. Butruille and B. Staels, PPAR control of metabolism and cardiovascular functions, Nat. Rev. Cardiol., 2021, 18, 809–823 CrossRef CAS.
- P. Petrus, S. Lecoutre, L. Dollet, C. Wiel, A. Sulen, H. Gao, B. Tavira, J. Laurencikiene, O. Rooyackers, A. Checa, I. Douagi, C. E. Wheelock, P. Arner, M. McCarthy, M. O. Bergo, L. Edgar, R. P. Choudhury, M. Aouadi, A. Krook and M. Rydén, Glutamine links obesity to inflammation in human white adipose tissue, Cell Metab., 2020, 31, 375–390 CrossRef CAS PubMed.
- R. Hursel and M. S. Westerterp-Plantenga, Catechin- and caffeine-rich teas for control of body weight in humans, Am. J. Clin. Nutr., 2013, 98, 1682S–1693S CrossRef CAS PubMed.
- H. Steinbrenner, L. H. Duntas and M. P. Rayman, The role of selenium in type-2 diabetes mellitus and its metabolic comorbidities, Redox Biol., 2022, 50, 102236 CrossRef CAS PubMed.
- D. Ferrara, F. Montecucco, F. Dallegri and F. Carbone, Impact of different ectopic fat depots on cardiovascular and metabolic diseases, J. Cell. Physiol., 2019, 234, 21630–21641 CrossRef CAS.
- T. Suganami, M. Tanaka and Y. Ogawa, Adipose tissue inflammation and ectopic lipid accumulation [Review], Endocr. J., 2012, 59, 849–857 CrossRef CAS PubMed.
- M. Kratz, B. R. Coats, K. B. Hisert, D. Hagman, V. Mutskov, E. Peris, K. Q. Schoenfelt, J. N. Kuzma, I. Larson, P. S. Billing, R. W. Landerholm, M. Crouthamel, D. Gozal, S. Hwang, P. K. Singh and L. Becker, Metabolic dysfunction drives a mechanistically distinct proinflammatory phenotype in adipose tissue macrophages, Cell Metab., 2014, 20, 614–625 CrossRef CAS.
- G. S. Hotamisligil, Inflammation, metaflammation and immunometabolic disorders, Nature, 2017, 542, 177–185 CrossRef CAS.
- G. S. Hotamisligil, Inflammation and metabolic disorders, Nature, 2006, 444, 860–867 CrossRef CAS PubMed.
- Y. T. Wondmkun, Obesity, insulin resistance, and type 2 diabetes: Associations and therapeutic implications, Diabetes, Metab. Syndr. Obes., 2020, 13, 3611–3616 CrossRef CAS PubMed.
- C. B. Newgard, J. An, J. R. Bain, M. J. Muehlbauer, R. D. Stevens, L. F. Lien, A. M. Haqq, S. H. Shah, M. Arlotto, C. A. Slentz, J. Rochon, D. Gallup, O. Ilkayeva, B. R. Wenner, W. S. Yancy, H. Eisenson, G. Musante, R. S. Surwit, D. S. Millington, M. D. Butler and L. P. Svetkey, A branched-chain amino acid-related metabolic signature that differentiates obese and lean humans and contributes to insulin resistance, Cell Metab., 2009, 9, 311–326 CrossRef CAS.
- T. V. Rohm, D. T. Meier, J. M. Olefsky and M. Y. Donath, Inflammation in obesity, diabetes, and related disorders, Immunity, 2022, 55, 31–55 CrossRef CAS PubMed.
- J.-J. Li and J.-L. Chen, Inflammation may be a bridge connecting hypertension and atherosclerosis, Med. Hypotheses, 2005, 64, 925–929 CrossRef CAS PubMed.
- L. M. Coussens and Z. Werb, Inflammation and cancer, Nature, 2002, 420, 860–867 CrossRef CAS.
- S. Amor, F. Puentes, D. Baker and P. Van Der Valk, Inflammation in neurodegenerative diseases, Immunology, 2010, 129, 154–169 CrossRef CAS PubMed.
|
This journal is © The Royal Society of Chemistry 2024 |