Tiger nut (Cyperus esculentus) starch: extraction, composition, structure, properties, modification and uses
Received
20th December 2023
, Accepted 13th March 2024
First published on 26th March 2024
Abstract
Although tiger nut, the tuber of Cyperus esculentus L., is regarded as a new source of edible oil, it also constitutes up to 14–37% of starch on a dry basis. Since it is globally popular and widely cultivated in Africa, Asia, Europe and America, tiger nut is a promising and underutilized source of commercially available starch. To date, there is a lack of systematic understanding of tiger nut starch. Herein, we mainly focus on tiger nut starch, including its extraction, chemical composition, structure, properties, modification and application aspects. The chemical composition of tiger nut starch is proven to be markedly affected by its extraction method. The amylose content of tiger nut starch is reported to vary from 9.71% to 27.01%. Tiger nut starch is mostly spherical and oval, and its granule size ranges from 2 to 18.53 μm with an A-type crystallinity. Compared with common starch (such as wheat, corn, potato, and cassava starch), tiger nut starch shows unique differences in fine molecular structures, swelling power, solubility, thermal properties, pasting properties and in vitro digestibility. In order to improve its properties and potentially widen its uses, tiger nut starch has been modified by physical, chemical, enzymatic and dual methods. Besides, tiger nut starch has great potential for food and non-food uses. This review is worthy for the further development of tiger nut as a sustainable crop as well as for the value-added utilization of tiger nut starch.
Sustainability spotlight
Tiger nut (Cyperus esculentus L.) is a worldwide cash crop and has long served as a food source in many countries. Recent investigations show that tiger nut is a valuable source of vegetable oils, starch, and bioactives, providing about 400–450 kcal·100 g−1 energy. Despite the potential health benefits, tiger nut and its starch are largely underutilized. Starch is the main component in the by-product of “horchata” and/or the tiger nut oil manufacturing process. The exploitation of this underutilized starch relates to the sustainable utilization of tiger nut by-products and development of new materials. This review summarizes the present knowledge of the extraction, chemical composition, granular and molecular structural characteristics, physicochemical properties, digestibility, modifications, and uses of tiger nut starch and provides future research directions. Our work emphasizes the importance of the following UN's Sustainable Development Goals (SDGs): no poverty (SDGs 1), zero hunger (SDGs 2), and good health and well-being (SDGs 3).
|
1 Introduction
Tiger nut, scientifically known as Cyperus esculentus L., is a monocotyledonous graminoid flowering plant that belongs to the Cyperaceae family.1 It produces spherical tubers at the end of its rhizomes. These rhizomatous tubers are edible and have a sweet taste with a nutty flavour, like almonds. Tiger nut is known by several names, such as earth almond, earth chestnut, tiger nut, tiger nutsedge, edible rush, chufa and Zulu nut. Tiger nut has been cultivated in Egypt and the surrounding Mediterranean regions for centuries.2 Over time, tiger nut has spread to other parts of the world, including Spain, Ghana, Nigeria, the United States, China, Ivory Coast, India, Chile, Brazil and Australia.3,4 Since its introduction in China in the 1950s, large-scale commercial plantations of tiger nut began in the early 21st century. In 2019, the plantations and productions of tiger nut in China reached 1.33 × 104 hectares and 7.98 × 104 to 2.66 × 105 tonnes (dry matter).5 Tiger nut has become a subsistence crop as well as a cash crop in many different parts of the world due to its high yield and nutritional and commercial values.
Tiger nut has long been used as a food source in many civilizations, utilized in a variety of culinary dishes, and even renowned for its therapeutic benefits. Tiger nut is a good source of starch, fat, protein, minerals (such as sodium, magnesium, manganese, iron, potassium and calcium), unsaturated fatty acids (mostly oleic acid), dietary fibers and vitamins (C and E).6,7 In addition, it contains various bioactive compounds (such as alkaloids, tannins, phytates, phytosterols, glycosides, saponins, and flavonoids), which are essential to human health.8 In particular, tiger nut can be a major source of edible oil production because of the 19.79–37.83% fat accumulated in its tuber.6 Recently, tiger nut has attracted attention as a functional food due to biological activities, such as antioxidant, antimicrobial, anti-inflammatory, antitumor, anti-sickling, aphrodisiac potential, anti-atherosclerotic activity, and hepatoprotective activity.8 Hitherto, various food products have been developed from tiger nut. In Spain, tiger nut has been served as a milk-like beverage called “Horchata de chufa”. Horchata de chufa has gained enormous popularity in various countries, including France, Portugal, the United Kingdom, China and Argentina.9 The cold-pressed tiger nut oil is excellent for cooking and salad dressing. Tiger nut flour has been commonly used as an ingredient for bread, pasta, biscuits, cakes, and cookies.10–13 Apart from the tuber of tiger nut for food uses, it is also used as an agricultural fertilizer, animal feed and even medical use, but these have not yet been scientifically investigated.
Among all the nutrients, starch is a major component of tiger nut, which accounts for 14–37% of the dry weight of tiger nut tubers. Extraction of starch from tiger nut tubers, defatted tiger nut meals or oil-cakes is feasible on an industrial scale. In recent years, the starch market has expanded and improved. Due to increasing limitations on traditional starch supplies such as wheat, maize, potato and cassava, the exploration of new starches from non-traditional, underutilized sources is highly encouraged. Compared with commercial starches, tiger nut starch (TNS) possesses some distinctive characteristics such as moderate amylose content (9.71–27.01%),14,15 excellent pasting properties and low digestibility (11.0% of resistant starch in the gelatinized starch), which indicates the potential uses of TNS as a promising starch resource. Recent research has shown that TNS can be an ingredient for food and non-food applications.16–18 However, a lack of systematic knowledge hinders the efficient utilization of TNS as a sustainable crop resource.
Some previous reviews have summarized some aspects of tiger nut, including the taxonomy, biology,19 distribution, habitat requirements,20 traditional and culinary uses, nutritional and health-promoting value, extraction of tiger nut oil,6 tiger nut drink “horchata de chufa”21 and by-product valorisation.2 Therefore, the objective of this review is to summarize the present knowledge on the extraction, chemical composition, granular and molecular structural characteristics, physicochemical properties, digestibility, modifications, and uses of TNS and to provide future research directions.
2 Extraction of tiger nut starch
Tiger nut is rich in starch, oil, protein, dietary fibre, and other nutrients. Starch accumulated in tiger nut tuber starts at the beginning of tuber development and grows continuously until the tuber reaches its mature stage (harvest stage).22 After harvest, tiger nut is always dried for storage (Fig. 1A). At the lab scale, the extraction of TNS can be achieved by a wet-milling method involving the following processes: washing the dried tubers with water, steeping (Fig. 1B), grinding (the image of tiger nut flour is shown in Fig. 1C), filtering (repeated if necessary), precipitation, drying and packaging.23,24 Practically, due to oil in the tuber, starch isolation is performed after oil extraction in most cases.25 Various oil extraction methods were applied to remove the oil. The conventional oil extraction techniques include organic solvent extraction (mainly n-hexane) and expeller mechanical pressing (cold pressing and hot pressing). Miao et al.26 and Lv et al.16 applied n-hexane to remove the oil before TNS extraction. If necessary, the n-hexane soaking is repeated up to twice. Similarly, Manek et al.27 and Liu et al.28 applied petroleum ether to extract oil from the tubers before starch isolation. The new developing technologies for oil extraction or removal include supercritical CO2 extraction and aqueous enzymatic extraction. As the oil removal is completed, the resulting meals or cakes are further processed for starch extraction. In order to facilitate starch granules liberating from the matrix or the removal of impurities, neutral or alkali solutions instead of distilled water were employed to steep the dried tubers or press-cakes. Umerie et al.23 steeped the tuber in potassium metabisulphite solution and reported a yield of 20.51% on a dry weight basis. Apart from potassium metabisulphite solution, sodium metabisulphite solution was also applied in the steeping process.27,29 When sodium hydroxide was used in the steeping process, a so-called alkali method was applied for starch isolation. Jing et al.25 applied the alkali method, in which the tiger nut meal was soaked in 0.15% sodium hydroxide at the solid–liquid ratio of 1
:
15 (w/v). The starch purity was 89.81% in their study. Different extraction methods can alter the compositions and properties of starch to a certain extent, such as starch yield, ash content, whiteness value, swelling power and so on.28,30 Liu et al.28 applied ultrasound-assisted alkali method to extract starch from tiger nut tubers and the extraction yield was 92.2%. They concluded that ultrasonic treatment could reduce the combination of starch, protein, and dietary fibre in tiger nut tubers during extraction of the starch, thereby improving the extraction yield and shortening of the extraction time. Liu et al.29 found that the TNS isolated from the cake after cold press extraction exhibited the highest water absorption capacity. Miao et al.31 reported that starch yield from alkali extraction of cold-pressed press-cake was 15.99%, which was significantly higher compared with that of native tiger nut flour (11.74%).
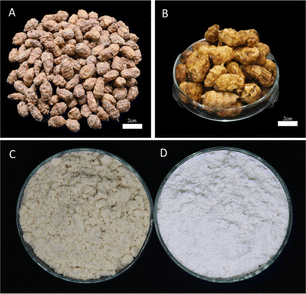 |
| Fig. 1 The images of the dried tubers of tiger nut (Cyperus esculentus) (A), rehydrate tubers of tiger nut (B), tiger nut flour (C), and isolated starch of tiger nut (D). | |
Some researchers investigated the effects of oil extraction methods on the properties of TNS. Liu et al.32 found that starches isolated from the meal after various oil extraction processes varied significantly in granule size and amylose content. Compared with that of starch isolated from raw tiger nut tuber, the proportion of amorphous phase in the isolated starches from meals was relatively low. The starches isolated from meals differed in the branch-chain length distribution and exhibited a low average chain length of amylopectin. A change in starch molecules and a disruption in the ordered structure of starch granules were caused by the oil extraction processes. Furthermore, they found that starches isolated from tiger nut tuber after various oil extraction processes varied significantly in gelatinization and retrogradation properties.30
To date, several papers have focused on TNS isolation, which is far from industrial application. Methods for more efficient starch extraction, such as the physical field-assisted extraction method, are suggested. Moreover, the starch may undergo some modification by the extraction of tiger nut oil. Hence, further studies are needed regarding isolation methods for improving the quality and nutrition of TNS.
3 Chemical composition and structural characteristics of tiger nut starch
Compositionally, starch granules contain two major components, amylose and amylopectin, and other endogenous minor components, such as moisture, ash, protein and lipid. The components and structure of the starch markedly affect its properties and even applications. Thus, it is important to figure out the chemical compositions and structural characteristics of TNS.
3.1 Chemical composition
The TNS is an odourless, bright white or greyish-white powder (Fig. 1D).27 The TNS is the isolated form of the tuber, with the starch purity ranging from 87–97% (Table 1). However, the TNS extracted from tiger nut meal or cake has been characterized for its chemical compositions, and its purity of starch is quite high (Table 1). The content of starch in TNS samples is around 99.40% (w/w, dry basis).33 Thus, the contents of other components containing protein, lipid, and ash are relatively low.32,34,35 Besides, some samples show slightly higher contents of protein (1.23–4.40%) and lipid (8.41–9.26%) in the extracted starch.17,29 The reason was probably that protein and lipid were not thoroughly removed.29 The TNS granules are mainly composed of amylopectin and amylose. The amylose content of TNS has been quantified by various methods such as amylose/amylopectin assay kit (based on a Con A precipitation procedure),14 iodine binding colorimetric/spectrophotometry,24–26,29,32 and size-exclusion chromatography (SEC) of whole starch by high-performance SEC coupled with a differential refractive index detector.36 Different methods of quantification could provide different values of amylose content. Unfortunately, to date, there have been no studies comparing the analysis of different methods for the same starch sample. Results showed that the amylose content displayed a wide range, i.e., from 9.71% to 27.01% (Table 1). As compared with the amylose content of available starches, it is lower than that of mung bean starch (30.9–34.3%)37 and high-amylose wheat starch (28.0–36.9%).38 In most cases, the content of amylose in TNS was 15.0–24.0%, which was comparable with cassava starch (15.2–26.5%)39 and similar to sweet potato starch (8.5–37.4%),37 non-waxy rice starch (12.2–28.6%),40 normal potato starch (20.1–31.0%),41 and normal corn starch (22.4–32.5%).41 A lot of factors decide the amylose content of TNS, including genotypes, growing conditions and agronomic practices.1,42 Because TNS is one of relatively less known and underutilized starch sources, there is little known about other components in TNS, such as lipids, proteins, minerals and phytochemicals, which is of common occurrence in other tuber starches.43,44
Table 1 Chemical composition of tiger nut starche
Origin |
Extraction method |
Yield (%) |
Starcha (g/100 g) |
Amyloseb (g/100 g) |
Proteina (g/100 g) |
Lipida (g/100 g) |
Asha (g/100 g) |
Reference |
Dry matter.
On the basis of starch.
Not applicable.
Total carbohydrate.
n.d. not determined.
|
China |
Alkaline extraction |
26.16 |
87.74 |
24.07 |
— |
— |
— |
16
|
Nigeria |
—c |
— |
— |
— |
1.23 |
9.26 |
2.28 |
17
|
China |
Alkaline extraction |
— |
>90% |
23.57 |
— |
— |
— |
45
|
China |
Alkaline extraction |
— |
— |
9.71 |
— |
— |
— |
14
|
China |
Alkaline extraction |
— |
— |
14.88 |
0.25 |
0.34 |
— |
32
|
China |
Alkaline extraction |
21.95 |
— |
16.49 |
0.15 |
0.34 |
— |
34
|
Nigeria |
Potassium metabisulphite solution extraction |
20.51 |
— |
— |
— |
— |
0.07 |
23
|
China |
Alkaline extraction |
— |
89.81 |
16.18 |
0.53 |
0.42 |
0.21 |
25
|
China |
Ultrasound-assisted alkali method |
92.2 |
— |
— |
— |
— |
— |
28
|
Brazil |
Sodium hydrogen sulphite solution |
33.5 |
87.4d |
16.100 |
4.04 |
8.41 |
0.15 |
29
|
China |
Alkaline extraction |
13.22 |
91.13 |
14.92 |
0.22 |
0.55 |
— |
26
|
Ghana |
Wet milling |
— |
— |
19.1 |
— |
— |
— |
24
|
Ghana |
Wet milling |
— |
— |
21.9 |
— |
— |
— |
24
|
China |
Wet milling |
— |
96.8 |
27.01 |
— |
— |
— |
15
|
Nigeria |
Sodium metabisulfite solution |
37 |
— |
11.5 |
— |
0.3 |
1.0 |
27
|
China |
Alkaline extraction |
13.25 |
88.9 |
— |
0.35 |
0.6 |
0.3 |
46
|
China |
Alkaline extraction |
11.74–15.99 |
— |
— |
— |
— |
— |
31
|
China |
Alkaline extraction |
— |
90.0 |
17.0 |
0.2 |
0.6 |
0.3 |
35
|
China |
Wet milling |
— |
96.8 |
— |
— |
— |
— |
47
|
China |
Wet milling |
— |
91 |
23.96 |
— |
— |
— |
36
|
China |
Extracted from the tiger nut meal |
27.77 |
99.40 |
n.d. |
0.22 |
n.d. |
0.07 |
33
|
Egypt |
Alkaline extraction |
38.1 |
92.75 |
— |
0.83 |
1.11 |
0.74 |
48
|
3.2 Granular structure
The morphology of TNS has been investigated by various techniques, including light microscopy with polarized lens, scanning electron microscopy (SEM), and laser light diffraction-based particle size analysis.16,32 As shown in Fig. 2, TNS generally exists in the state of granules, and the starch granules have different sizes and shapes. According to SEM analysis, most of the TNS granules are spherical, oval or elliptical, and only a few were irregular.16,34 The TNS granules exhibited a polarization cross during the polarized light microscopic observation, indicating the existence of a spherocrystal structure.25 The starch granules had an average size of 2–18.53 μm (Table 2). Compared with the potato starch granules, the TNS granules were more compact and smaller.25 The particle size analysis indicated that the starch from some cultivars of tiger nut tuber had the largest size (average diameter, 18.53
μm), which was much higher than potato starch (average diameter, 15.45
μm) and corn starch (average diameter, 13.28
μm).16 Besides, a very small particle size of 0.182 μm was reported by Neto et al.29 according to the particle size distribution analysis. However, the data seemed inconsistent with SEM analysis, which could be estimated to 1.59–12.7 μm from the SEM image under the guidance of a scale bar. It should be noted that a great diversity in the granule size occurred when the samples were observed using different techniques. Interestingly, except for different shapes, the TNS exists as granules with varying sizes.25,29 Although the size dependencies on physicochemical and functional properties of starches from maize, potato, lotus root, etc. were examined by some researchers,49,50 to date, there is no report focusing on the differences in molecular structure and properties of TNS with varied granule sizes.
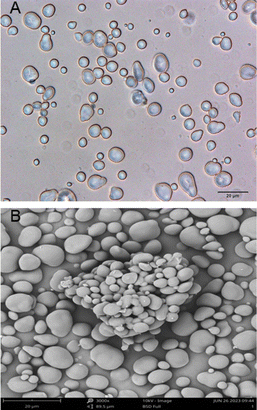 |
| Fig. 2 Characterization of tiger nut starch through optical microscopy (A) and scanning electron microscopy (B). Scale bar = 20 μm. | |
Table 2 Semi-crystalline structure and morphology of tiger nut starch
Polymorphic type |
2θ (°) |
Degree of relative crystallinity (%) |
Granule size (μm) |
Morphological characteristics |
References |
No mention.
RC by 13C NMR.
D50, i.e., median diameter.
D[4,3] i.e., the volume-weighted mean diameter.
|
A-type |
15°, 17°,18°, 23° |
38.22 |
—a |
Spherical granules with uneven sizes and relatively smooth surfaces |
45
|
A-type |
15°, 17°,18°, 23° |
20.6 |
8.78 |
Round, triangular or irregular shapes |
34
|
A-type |
18°, 23°, 26° |
10.36 |
8.60 |
Spherical, or elliptical and only a small number showed irregular shapes |
25
|
A-type |
15°, 17°, 18°, 22° |
— |
2–15 |
Ovoid, closer to those of tuber starch |
28
|
A-type |
15°, 17°, 18°, 23° |
— |
0.182 |
Smooth surface, mostly oval shapes |
29
|
A-type |
15.5°, 17.6°, 18.3°, 23.2° |
— |
8.66c |
— |
15
|
A-type |
15°, 17°, 18°, 23° |
20.14; 34.85b |
8.21d |
Irregular or oval in shape; smooth |
26
|
A-type |
15°, 17°, 18°, 23° |
14.91 |
8.22d |
Starch granule surface was irregular, with protruding horns |
31
|
A-type |
15°, 16.9°, 17.8°, 20.8°, 23°, 26.5°, 27.8° |
20.4 |
— |
A small amount was spherical granules, most were irregular |
51
|
A-type |
15°, 17°, 18°, 23° |
39.4 |
8.25 |
Oval to elliptical particles with a smooth surface |
27
|
A-type |
15°, 17°, 18°, 23° |
— |
— |
Either round or oval in shape |
52
|
A-type |
15°, 17.2°, 18.1°, 23.1° |
20.67 |
— |
Irregular shape with protruding horns |
46
|
A-type |
15°, 17.2°, 18.1°, 23.1° |
24.68 |
— |
Oval with protruding horns |
35
|
A-type |
15.4°, 17.5°, 18.3°, 23.4° |
46.12 |
— |
— |
53
|
A-type |
15.1°, 17.2°, 18.1°, 23.0° |
34.68 |
7.649 |
Elliptic with a smooth surface |
36
|
A-type |
15°, 17°, 18°, 23° |
30.47 |
12.34c |
Smooth surface; large particles were oval, and small particles were spherical |
54
|
A-type |
15.0°, 17.0°, 17.9°, 23.0° |
30.82 |
9.05c |
Smooth surface, with uneven sizes |
55
|
— |
— |
— |
11.1 |
Spherical granules with smooth surfaces; uneven sizes |
24
|
— |
— |
— |
6.1 |
Spherical granules with smooth surfaces; uneven sizes |
24
|
— |
— |
— |
4.57 |
— |
17
|
C-type |
5.6°, 15°, 17°, 23° |
25.0; 33.6b |
8.6 |
Triangular, irregular and round; smooth surface |
32
|
C-type |
15°, 17°, 23° |
11.3 |
— |
— |
56
|
C-type |
5.6°, 15°, 17°, 23° |
10.2 |
18.53 |
Most of the granules were spherical and oval, and a few were irregular |
16
|
3.3 Semi-crystalline structure
Similar to other sources of starch, TNS granules are formed by alternating growth rings of semi-crystalline and amorphous regions. The semi-crystalline region is mainly composed of amylopectin and a small amount of amylose.32,38 Different forms of crystalline polymorphism, including A, B and C-types, are present in the diverse starch sources. In general, most cereal starches show an A-type diffraction pattern, while some tubers and rhizome starches show a B-type pattern. Legume starches often present the C-type pattern, and starches with amylose compounded by lipids and other compounds have the V-type pattern. According to the XRD patterns,32 most of the TNS samples displayed a typical A-type diffraction pattern. The A-type diffraction pattern was probably due to the low intensity of the diffraction peak at 5.6°, which was vulnerable to moisture content of the samples Manek et al.27 However, some researchers reported that TNS showed a C-type crystal structure.28 Lv et al.16 reported that TNS was a C-type starch with a relative crystallinity of 10.2%. There were strong diffraction peaks at approximately 15°, 17°, and 23° and a small diffraction peak at 5.6°, which was the typical C-type starch XRD pattern. Liu et al.32 also reported the C-type crystal structure of TNS. The starch showed small but visible peaks at 2θ of approximately 5.6° and 15° and strong diffraction peaks at 2θ of approximately 17° and 23°. They also indicated a low amount of the amylose–lipid complexes in the extracted TNS, which was proven by the small peak at 2θ of approximately 20°.32 Great variation in the degree of relative crystallinity is indicated in Table 2, ranging from 10.36% to 46.12%. The data from the reported publications for these starches were isolated from tiger nut tubers of different origins all over the world. These starches differed in the amount and molecular structures of amylose and amylopectin, crystal sizes, and particle sizes. Thus, the crystalline structure of TNS was affected by these factors and showed great diversity.31,34
Small-angle X-ray scattering (SAXS) is a non-disruptive technique that can probe starch granules in their intact state to analyse the amorphous, ordered and lamellar structures of starch. After SAXS measurements, two-dimensional scattering images were obtained and then converted to one-dimensional images using the software. Parameters such as the thickness of semi-crystalline lamellae (dBragg), power-law index (α) and the fractal dimensions (D) could be calculated by analysing the SAXS data with fitting methods. To the best of our knowledge, there has been only one study reported in the literature on applying SAXS to discover differences in the lamellar structure for TNS.36 The results indicated that the native TNS showed a scattering peak at 0.64 nm−1. By thermal treatments, the peak almost disappeared and the intensity of scattering at scattering vector (q) lower than 0.6 nm−1 increased. The lamellar repeat distance (d) and the thickness of crystalline lamellae (dc) and amorphous layers (da) of native TNS were 9.27, 3.73, and 5.55 nm.36 The dielectric barrier discharge plasma treatment had little effect on d (9.29 nm), dc (3.80 nm), and da (5.49 nm) of TNS.36 In this paper, we adopted SAXS to study the semi-crystalline lamellae of TNS and the results are presented in Fig. 3. The presence of the typical scattering peak around q of 0.86 nm−1 was clearly demonstrated (Fig. 3A), implying that the TNS granules displayed almost 7.31 nm long period structure on the basis of the Bragg's law D = 2π/q. In order to characterize the fractal structure of TNS, the SAXS intensity curve is transformed into a power-law curve (I ∼ qα).57 The value of α could be calculated from the slope of double-logarithmic SAXS patterns (Fig. 3B). The α value was −3.37 (−4 < α < −3). In this context, the fractal dimension could be calculated as Ds = 6 + α, which referred to a surface fractal.
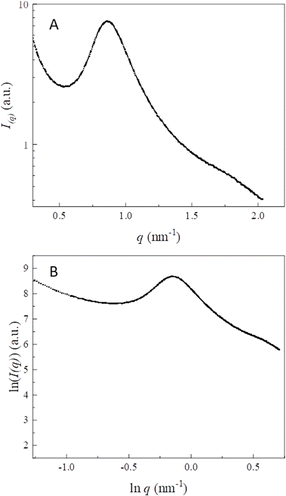 |
| Fig. 3 Small-angle X-ray scattering (SAXS) intensity profile of tiger nut starch (A), and its double-logarithmic SAXS intensity curve (B). The experiment was completed at the 1W2A beamline of Beijing Synchrotron Radiation Facility (BSRF) in Beijing, China. The tiger nut starch slurry (50% w/w) was loaded onto the well (5 mm diameter) of a 2 mm thick aluminum sample holder by KAPTON™ tape. The X-ray beam (Cu Kα) had a wavelength of 0.154 nm. | |
3.4 Molecular structure
The molecular size of starch has been measured by high-performance size-exclusion chromatography (HPSEC) coupled with a multi-angle laser light scattering (MALLS) and refractive index detector (RID). The molecular weight-average (Mw), number average molecular weight (Mn), and Mw polydispersity (Mw/Mn) values were analysed by using the software provided.32 Previous studies (Table 3) reported that TNS had a molecular weight of 7.43 × 107 g mol−1 (Mw) and 4.50 × 107 g mol−1 (Mn), with a polydispersity of 1.37 (PDI).14 The TNS had much greater Mw and Mn than jackfruit starch (Mw = 1.74–4.61 × 107 g mol−1; Mn = 1.10–2.34 × 107 g mol−1) and potato starch (Mw = 2.74 × 107 g mol−1).58,59 However, Mw of TNS was markedly lower than rice starch (3.04–8.20 × 108 g mol−1).60 Starch normally consists of two polysaccharide components: linear amylose and branched amylopectin. As reported by Liu et al.,32 the amylose fraction had a molecular weight of 6.93 × 105 g mol−1 (Mw) and the amylopectin fraction had a molecular weight of 4.41 × 106 g mol−1 (Mw). The reported Mw value of both TNS amylose and amylopectin was much lower than that of molecules from cowpea starch (amylose: 3.0–3.2 × 106 g mol−1; amylopectin: 8.16–8.74 × 107 g mol−1) and mung bean starch (amylose: 1.8–3.0 × 106 g mol−1; amylopectin: 6.65–8.88 × 107 g mol−1).61 However, the amylose Mw value of TNS was comparable to that of rice starch amylose (2.20–8.31 × 105 g mol−1).62
Table 3 Chain length distribution of amylopectin of tiger nut starch
Sample origin |
f
a (DP6-12) |
f
b1 (DP13-24) |
f
b2 (DP25-36) |
f
b3 (DP > 37) |
CLave |
Reference |
China |
33.1 |
53.05 |
9.00 |
2.41 |
2.09 |
14
|
China |
27.10 |
49.79 |
14.89 |
8.23 |
19.76 |
31
|
China |
26.13 |
51.25 |
11.12 |
11.51 |
20.03 |
26
|
China |
22.6 |
55.8 |
14.4 |
7.3 |
19.5 |
32
|
China |
23.83 |
51.05 |
13.31 |
11.80 |
21.01 |
34
|
China |
14.89 |
42.01 |
18.83 |
24.26 |
20.18 |
36
|
The branch-chain length distribution was determined using a high-pressure anion exchange chromatograph equipped with a pulsed amperometric detector (HPAEC-PAD). Prior to analysis, starch samples were gelatinized and debranched to obtain linear α-glucan amylopectin.32 Grouping of DP numbers categorized branched chains into four fractions: DP 6–12 (fa), DP 13–24 (fb1), DP 25–36 (fb2), and DP ≥ 37 (fb3), which correspond to A-chains (external short chains), B1 (singly branched chains), B2, (chains spanning two clusters) and long B3-chains (chains spanning three clusters), respectively.36 As shown in Table 3, the TNS has the greatest proportion of B1 chains at DP 13–24 (42.01–55.8%).14,26,31,32,34,36 Most of the samples are reported to have a smaller proportion of B2 and B3 chains than A chains. To date, only one case reported that TNS has the smallest proportion of the A chain (14.89%).36 Besides, the average chain length of amylopectin of TNS was reported in the range of 19.5–21.01.
4 Properties of tiger nut starch
The distinct properties of starch are important for its applications. Hence, we summarize the physicochemical and functional properties of TNS, such as swelling power, solubility, gelatinization properties, pasting and rheological properties, paste clarity or light transmittance, freeze–thaw stability, gel textural property and in vitro digestibility.
4.1 Swelling power and solubility
The ability of starch to swell and eventually produce a paste when heated in water is one of its essential features. The swelling power (SP) of starch is the mass fraction of water adsorbed by dried starch after heating in aqueous solution at a given temperature, while the solubility (SOL) represents the percentage of leached fraction or the dissolution rate of starch granules. As discussed in the literature (Table 4), great diversity exists in SP and SOL of TNS. It may be influenced by cultivars,24 extraction methods, starch granular characteristics, molecular structure63 and aqueous temperatures.64 Some researchers reported that TNS of different cultivars showed the SP and SOL values at 75 °C varying from 9.41–21.4 g g−1 and 4.87–17.8%, respectively.32,35,46 Akonor et al.24 compared the SP and SOL of starch isolated from two varieties of tiger nut. The SP of yellow and black TNS was 11.3 and 9.9 g g−1, respectively, while the SOL of yellow and black TNS was 13.5% and 11.6%, respectively. They explained that the black cultivar showed lower SP and SOL due to a significantly higher content of amylose. Liu et al.32 reported that the SP and SOL of TNS were significantly affected by the extraction method. The TNS extracted from raw tiger nut tubers had the lowest SOL (4.87%) and SP (9.41 g g−1) at 75 °C than the starch from the defatted meals and cakes (SOL 10.51–18.94%; SP 11.77–25.52 g g−1). Liu et al.35 observed that the SOL and SP of TNS were very limited (1.5% and 3.4 g g−1) at 65 °C, but it soared to 17.8% and 21.4 g g−1 at 75 °C. However, Lv et al.16 indicated that the SP and SOL of TNS at 65–95 °C increased in a temperature-dependent manner. Lv et al.56 indicated that by increasing the heating temperature from 50 °C to 90 °C, the SP and SOL of TNS (variety, Yuyousha 1) steadily grew from around 4 g g−1 at 50 °C to around 12 g g−1 at 90 °C, and the SOL ranged from around 2% at 50 °C to around 11% at 90 °C.
Table 4 Thermal properties of tiger nut starch measured using differential scanning calorimetry
Origin |
Starch : water |
Scanning rate (°C min−1) |
T
o (°C) |
T
p (°C) |
T
c (°C) |
T
c − To/(°C) |
ΔH (J g−1) |
Reference |
No mention of the amount of water.
Enthalpy of gelatinization [J (g−1 K−1)].
Not applicable.
|
China |
3 mg : 7 mg |
10 |
66.18 |
71.53 |
78.15 |
11.97 |
12.02 |
45
|
China |
70% water (3 : 7) |
10 |
64.4 |
69.8 |
84.0 |
19.6 |
23.9 |
32
|
Brazil |
4.0 mg starcha |
10 |
62.27 |
67.52 |
75.55 |
13.28 |
228.86 |
29
|
China |
2.5 mg : 7.5 μL |
10 |
65.9 |
70.9 |
80.2 |
14.3 |
15.6 |
26
|
China |
1 : 3 (w/w) |
10 |
64.73 |
69.89 |
80.50 |
15.77 |
16.34 |
46
|
Nigeria |
5 mg starcha |
10 |
60.35 |
125.59 |
134.44 |
74.09 |
1926.95b |
64
|
China |
2.5 mg : 7.5 μL |
10 |
65.48 |
70.96 |
80.39 |
14.91 |
16.57 |
35
|
China |
3.0 mg : 9.0 μL |
10 |
60.87 |
70.63 |
81.26 |
20.39 |
14.01 |
36
|
China |
—c |
— |
67.86 |
72.13 |
79.61 |
11.75 |
12.94 |
28
|
China |
70% water (3 : 7) |
10 |
68.03 |
72.47 |
79.59 |
11.56 |
13.77 |
70
|
China |
2 mg : 4 μL |
10 |
65.26 |
72.09 |
— |
— |
3.61 |
54
|
China |
1 : 3 (g mL−1) |
5 |
68.13 |
72.69 |
79.52 |
11.39 |
3.54 |
55
|
4.2 Thermal properties
Starch gelatinization can be studied using various techniques. Among them, differential scanning calorimetry (DSC) is commonly used. The parameters of gelatinization, including the onset (To), peak (Tp), and conclusion (Tc) temperatures, are related to the energy consumption for the initial, peak and conclusion gelatinization of starch, respectively.32 The diversity in thermal properties of TNS measured by DSC is summarized in Table 4. The gelatinization temperatures To, Tp, and Tc of TNS are in the range of 60.87–68.13 °C, 67.52–72.69 °C, and 75.55–84.0 °C, respectively. As shown in Table 4, the Nigeria sample shows unusual Tp and Tc values, which is probably due to the limited water in the DSC sample. Many factors may affect starch gelatinization, such as starch sources, cultivars, granule size, amylose content, molecular structure, crystalline structure, and even processing conditions. Up to date, it is difficult to directly compare the thermal properties of TNS due to the lack of data from the references. However, it is feasible to compare TNS with other starches with different polymorphs (A-, B- and C-type polymorphs). TNS have higher To, Tp, and Tc than some A-type starches from cereal sources such as wheat (57.1 °C, 61.6 °C, 66.2 °C, respectively), barley (56.3 °C, 59.5 °C, 62.9 °C, respectively), and waxy rice (56.9 °C, 63.2 °C, 70.3 °C, respectively), while having similar or lower gelatinization temperatures than B- and C-type starches such as green banana (68.6 °C, 72.0 °C, 76.1 °C, respectively), water chestnut (58.7 °C, 70.1 °C, 82.8 °C, respectively), Amylomaize V (71.0 °C, 81.3 °C, 112.6 °C, respectively), and sweet potato starches (65.91–73.94 °C, 75.21–79.35 °C, 82.14–85.71 °C, respectively).65,66 The enthalpy of gelatinization (ΔH) is connected to the quantity and quality of crystallinity and is an indicator of the loss of starch molecular order with the granules.41 The ΔH of TNS was comparable to rice (9.0–13.0 J g−1)67 and cassava (11.2–13.4 J g−1)68 starches, and higher than wheat69 (4.63–6.32 J g−1) and sweet potato44,66 starches (2.96–11.64 J g−1). The gelatinization of starch could be affected by several factors, such as cultivars, particle size, amylose content, molecular structure of amylose and amylopectin, co-existing constituents and even the processing operations. Liu et al.32 reported that starches isolated from tiger nut tubers before and after the oil extraction processes showed differences in gelatinization properties. Among them, the starch from the cakes after hot pressing of tiger nut tubers exhibited the narrowest range of transition temperature (ΔT = Tc − To = 14.2 °C) and the lowest conclusion temperature (Tc = 78.9 °C) and enthalpy (ΔH = 13.4 J g−1), while starch from untreated tiger nut tubers had the greatest values of ΔT (19.6 °C), Tc (84.0 °C) and ΔH (23.9 J g−1). This could be mainly attributed to the alteration of crystalline structure and short-range conformation of starch during the oil extraction process.32 Wang et al.36 found that, compared to natural TNS, a slight decrease in Tc and ΔH and a significant increase in To (P < 0.001) of the starch was caused by dielectric barrier discharge plasma treatment (16 kV, 50 Hz, 10 min). They explained that it was ascribed to the mildly reduced long-range order degree of starch. Cao et al.54 reported that heat moisture treatment of TNS led to an increase in To and Tp from 65.26 °C to 72.07 °C, and from 72.09 °C to 78.64 °C, respectively, while a decrease in ΔH from 3.61 J g−1 to 1.63 J g−1.
4.3 Pasting and rheological properties
Pasting properties could decide the applications of starches. The measurement of pasting properties of starch is performed using a Brabender viscometer or a Rapid Visco Analyzer. When analysed by a Rapid Visco Analyzer, 3.0 g of starch sample was uniformly dispersed in 25 mL of distilled water for pasting property determination. The peak, trough, final, breakdown, setback viscosities and pasting temperature were obtained from pasting curves. The pasting temperature is the temperature at which the viscosity of the starch aqueous solution begins to increase and it measures the ability of granules to remain intact.26 As shown in Table 5, the pasting temperature of raw TNS was ranging from 66.5 °C to 80.9 °C. The peak viscosity of TNS was ranging from 2929 cP to 4653 cP. The trough viscosity of TNS was ranging from 1703 cP to 4048 cP. The final viscosity of TNS was ranging from 3152 cP to 6074 cP. Furthermore, the pasting properties of TNS could be altered by the addition of non-starch polysaccharides or other additives, which is attributed to the additive interacting with or without amylose or amylopectin leached from starch granules.35 Builders et al.71 found that the pasting temperature was highest at pH 7 compared to pH 4 and 9.2, and the pasting viscosities were affected by pH. They concluded that the peak and breakdown viscosities in pH 9.2 were about twice that in pHs 7 and 4, respectively. The set back and final viscosities in pH 9.2 were about five times and fourteen times at pHs 7 and 4, respectively.
Table 5 Swelling power, solubility and pasting properties of tiger nut starcha
Temperature (°C) |
Swelling power (g g−1) |
Solubility (%) |
Pasting properties |
Reference |
Ratio of starch to water |
PT (°C) |
PV (cP) |
TV (cP) |
FV (cP) |
BD (cP) |
SB (cP) |
SB (%) |
The pasting properties of tiger nut starch were measured using RVA; pasting temperature (PT), peak viscosity (PV), trough viscosity (TV), and final viscosity (FV) were recorded, while breakdown viscosity (BD = PV − TV) and setback viscosity (SB = FV − TV) were calculated; SB% = SB/TV × 100%; “mPa s” was used as the unit of viscosity in some studies; it is converted to “cP” (1 mPa s = 1 cP) here.
Not applicable.
Brabender units (BU).
|
—b |
— |
— |
10% (w/w) |
— |
235.50 |
231.00 |
233.00 |
4.50 |
2.00 |
0.87 |
45
|
80 °C, 30 min |
8.19% |
5.50 |
— |
— |
— |
— |
— |
— |
— |
— |
63
|
55–85 °C, 60 min |
1.93–14.80 |
0.22–8.60 |
— |
— |
— |
— |
— |
— |
— |
— |
32
|
— |
— |
— |
3.0 g : 25 mL |
76.3 |
4443 |
1931 |
3152 |
2512 |
1221 |
63.23 |
34
|
— |
— |
— |
3.0 g : 25 mL |
76.3 |
4413 |
4048 |
6074 |
365 |
2026 |
50.05 |
30
|
85 °C, 30 min |
11.3 |
13.5 |
10% starch slurry |
66.5 |
866.4c |
310.1c |
515.1c |
556.3c |
205.1c |
66.14 |
24
|
85 °C, 30 min |
9.9 |
11.6 |
10% starch slurry |
71.4 |
879.3c |
421.3c |
783.1c |
458.2c |
363.2c |
86.21 |
24
|
55–95 °C, 30 min |
∼1–7.5 |
∼1–19 |
3.0 g : 25 mL |
77.22 |
4076 |
3215 |
5094 |
860 |
1879 |
58.44 |
16
|
— |
1.13 |
2.46 |
3.0 g : 25 mL |
79.05 |
3976.00 |
2056.00 |
3414.00 |
1920.00 |
1358.00 |
66.05 |
17
|
75 °C, 85 °C, 60 min |
18.3–27.7 |
17.6–20.2 |
3.0 g : 25 mL |
76.0 |
3947 |
1890 |
3206 |
2058 |
1317 |
69.68 |
46
|
— |
— |
— |
3.0 g : 25 mL |
77.3 |
3668.7 |
1848.3 |
3049.3 |
1851.7 |
1200.0 |
64.92 |
26
|
30–90 °C, 30 min |
2.33–7.44 |
— |
— |
— |
— |
— |
— |
— |
— |
— |
64
|
65–95 °C, 60 min |
3.4–33.2 |
1.5–18.4 |
3.0 g : 25 mL |
77.0 |
3705 |
1882 |
3143 |
1864 |
1147 |
60.95 |
35
|
50–90 °C, 30 min |
∼4–12 |
2–11 |
3.0 g : 25 mL |
78.07 |
2929 |
2098 |
3249 |
831 |
1151 |
54.86 |
56
|
— |
— |
— |
3.0 g : 25 mL |
73.10 |
4653 |
1703 |
3062 |
2950 |
1359 |
79.80 |
36
|
— |
— |
— |
3.0 g : 25 mL |
79.52 |
3910 |
2952 |
4272 |
958 |
1320 |
44.72 |
33
|
— |
— |
— |
2.5 g : 18.5 mL |
72.65 |
7356 |
3517 |
5959 |
3839 |
2442 |
69.43 |
76
|
The rheological properties of starch paste could be probed using a rheometer. The information regarding the flow properties of the paste was consequently obtained with a steady flow test, which was performed at 25 °C at a shear rate typically ranging from 0.1 to 100 s−1 to measure the effect of the shear rate (γ) on the apparent viscosity (ηap) and shear stress (τ). The data were described by the Ostwald–DeWaele model (power-law model.) and Herschel–Bulkley model. By interpreting the equations, some parameters, including consistency coefficient (K) and flow behavior index (n), could be calculated to indicate the flow behavior of the paste. Some researchers14,15,30 reported that the Herschel–Bulkley model was suitable for the description of flow characteristics of starch paste. In some other cases, the experimental data were described by the Ostwald–DeWaele model.26,35,46 Typically, the tiger nut starch paste showed a shear-thinning behavior, in which the viscosity of the paste decreased gradually with the increasing shear rate.15 Except for the steady shear properties, the viscoelastic properties of the paste could be interpreted by the measurement results of a dynamic oscillatory shear using strain sweep and frequency sweep. Usually, the strain sweep test was applied to determine the linear viscoelastic region (LVR) of the samples. Then, the frequency sweep test over a range of 0.1 to 100 rad s−1 at a certain strain within the LVR was performed and obtained the effect of frequency (ω) on the storage modulus (G′), loss modulus (G′′) and loss tangent (tan
δ = G′′/G′) of the sample. Miao et al.26 observed that, for the tiger nut starch paste that stood for 24 h at 4 °C, the variation of G′ and G′′ with frequency was not significant.26 However, Li et al.14 found that the magnitudes of G′ and G′′ increased with an increase in ω, showing a frequency dependency.
4.4 Light transmittance
Light transmittance or clarity is an essential property of starch pastes or starch gels, especially for industrial uses, and varies considerably among different botanical sources. As hypothesized by Craig, Maningat, Seib, and Hoseney,72 swollen starch granules allow light to pass through them (instead of being reflected) because their ability to reflect light weakens as starch molecules dissociate. This phenomenon is responsible for the clarity or opacity of starch gels. Consequently, the presence of granule remnants and the interaction between leached materials cause high opacity. Additionally, factors that restrict swelling and dispersibility, such as amylose content and its conformation, lipids, and cross-linking, negatively affect the clarity of starch gels, whereas the presence of sucrose improves clarity. Generally, while starches from tuber crops (e.g., cassava and potato) form transparent gels, gels from cereals such as maize and wheat are opaque. Jing et al.25 reported that the paste of TNS was less transparent (transmittance 62.40%) than the potato starch paste (87.60%) when they were in 1% (w/w) concentration. The paste clarity (%) of starches from yellow and black tiger nuts were 30.8 and 14.7, respectively, which was measured at 650 nm on a UV-vis spectrophotometer against a water blank by Akonor et al.24 As they explained, this probably was a result of its lower amylose content and larger granule size. Liu et al.32 observed that the paste clarity (% transmittance) of starch from raw tiger nut tubers was 7.05. They also found that the paste clarity of starch extracted from TNS tuber meals or oil-cakes was significantly improved. Paste clarity of starch from the meal after hexane extraction of the tiger nut tubers was the highest among them (24.90), which was comparable to yam73 and wheat74 starches. Although the paste clarity was attributed to several factors, they explained that the higher paste clarity for the TNS isolated from meals and cakes might be attributed to the relatively larger granules, granular swelling power and smaller proportion of A-chains in amylopectin compared with the starch from raw tiger nut tubers.32 The paste clarity decreased with storage time at 4 °C.32 After 4 d, the starch from the meals after hexane extraction showed maximum paste clarity (10.55%), whereas starch from the raw tiger nut tubers showed minimum paste clarity (2.55%). Therefore, the TNS has its choice for use in food formulations where clarity is required to different extents, such as in soups, creams, desserts, pies, gravies, or sauces.
4.5 Freeze–thaw stability
The freeze–thaw stability of starch pastes is defined as the ability to withstand undesirable physical changes during the freezing and thawing cycles. The freeze–thaw stability of starch is commonly evaluated by measuring syneresis, i.e., the amount of water released from the gelatinized starch, which can be used to indicate its tendency to retrograde. Jing et al.25 reported that the freeze–thaw stability of TNS paste was slightly higher than that of potato starch. Liu et al.32 reported that freeze–thaw starches from the meals and cakes had significantly higher syneresis values (27.71–40.31%) compared with the starch from raw tiger nut tubers (14.99%) after the fifth cycle. Lv et al.16 reported that the freeze–thaw stability (%) of starches from tiger nut, corn and potato were 15.23, 26.56, and 37.67, respectively. The results indicated that starch from this cultivar had potential application in frozen foods. Besides, assessing syneresis is the most direct way to determine the degree of retrogradation and stability of a gel system. Builders et al.71 reported that the TNS gels showed significant pH sensitivity in terms of syneresis. The TNS gels prepared with a buffer solution of pH 9.2 exhibited minimal syneresis, and the extent of syneresis of TNS gels decreased from acidity (pH 4) through neutrality (pH 7) to alkalinity (pH 9.2). Akonor et al.24 found that yellow and black TNS showed an exudate of 65% and 68% of the initial weight of the gel. Miao et al.46 investigated the freeze–thaw stability of TNS gels and found that the gel syneresis increased from 34.62% to 41.04% with an increase in the freeze–thaw cycle from 1 to 5. Liu et al.35 found that syneresis of the TNS ranged from 45% to 58% after one to five freeze–thaw cycles, and these values were lower than those of corn, mung bean, and potato starches, as reported by Kaur et al.75
4.6 Gel textural property
The textural properties of the TNS gels were measured with a texture profile analyser. Accordingly, textural parameters of cohesiveness, hardness, adhesiveness, and springiness of the gels were determined. There is a great diversity of textural properties of TNS gels, as per various reports. Lv et al.16 investigated the gel strength of starches from tiger nut tubers, corn and potato at different concentrations (8%, 10%, and 12%, w/w). The gel strength showed no significant difference among these starches at a starch concentration of 8%. However, the gel strength of the gel developed by TNS at the concentrations of 10% and 12% was higher than that of corn and potato starch. Besides, the TNS gel at 10% starch concentration had the greatest springiness (0.92) and hardness (3196 g) compared with the corn and potato starches.16
4.7
In vitro digestibility
Starch is one of the major energy sources for human beings in daily life. From a nutritional perspective, starch is simply classified into rapidly digestible starch (RDS), slowly digestible starch (SDS) and resistant starch (RS), and their corresponding contents are calculated according to the released amount of glucose during digestion.76 RDS can give rise to a high postprandial glycemic response, whereas SDS and RS slowly release glucose and are good for humans to maintain normal blood glucose levels.77 In this context, it is meaningful to investigate the digestibility of TNS in raw (granular), gelatinized, and retrograded states. Lv et al.63 reported that the RDS, SDS, and RS of the uncooked TNS from the cultivar Yuyousha No. 1 were 30.22%, 34.47%, and 35.31%, respectively. The structural compactness of starch granules largely hindered enzymatic digestion. After cooking, the RDS, SDS, and RS of TNS were changed to 59.32%, 16.45%, and 24.23%, respectively. Liu et al.28 found that the RDS, SDS, and RS of TNS in the gelatinized state were 75.13%, 13.87% and 11.0%, respectively. The gelatinized TNS showed the highest RS content (11.0%) among the six starches (from sweet potato, potato, cassava, wheat, dent corn, and tiger nut) in the study. However, Li et al.14 reported that gelatinized TNS digested rapidly in vitro, showing RDS (97.97%), SDS (1.24%) and RS (0.79%), respectively. The difference in digestibility was probably due to the structural diversity of TNS from different cultivars. However, TNS from some cultivars (such as Yuyousha No. 1) could be utilized as a potential ingredient for the development of slowly digestible starchy foods. Usually, retrogradation could reduce the digestibility of starch. Up to now, the digestibility of retrograded TNS has not yet been explored.
5 Modification of tiger nut starch
Native starch has limited functional properties, and modification is adopted to overcome the drawbacks of native starch and create a wide scope of functionalities for food and non-food uses. To date, TNS has been modified physically, chemically, and/or biologically to endow new techno-functionalities for industrial demands. The recent findings on the modification of TNS are described here.
5.1 Physical modifications
The isolated TNS has been subjected to dry heat treatment, heat moisture treatment and other physical modifications. Dry heat treatment confines the starch chain through low moisture content (<10%) and destroys the starch structure at high temperatures (100–150 °C), resulting in changes in the structure of the crystalline and amorphous regions in the starch molecule. Miao et al.46 found that dry heat treatment destroyed the surface and crystal structure of the starch granules. Besides, the peak, trough and final viscosity of starch with dry heat treatment (130 °C for 2 h, 7% moisture) were 16%, 16%, and 10% more than that of the raw starch, respectively. They confirmed that the treatment with dry heat (130 °C for 2 h, 7% moisture) and the addition of Chinese quince seed gum (1%, w/w) further improved the physicochemical properties of TNS, in particular, by the increasing peak, trough, and final viscosities and freeze–thaw stability.46 Heat moisture treatment refers to the treatment for a period of time with low moisture content (typically 10–35%) and a certain temperature (above the glass transition temperature but below the gelatinization temperature). Cao et al.54 found that the SOL and SP gradually decreased as the moisture content was increased, while the in vitro digestibility was the lowest at the moisture content of 25% (w/w), i.e., the proportion of SDS and RS in the TNS was 18.72% and 6.76%, respectively. Thus, heat moisture treatment could be considered a promising tool to promote TNS in the formulation of low glycemic index food. More recently, in the work of Wang et al.,36 TNS was treated with dielectric barrier discharge plasma (non-thermal, 16 kV, 10 min) and baking (120 °C, 40 min), high-pressure cooking (70 kPa, 13 min), radio frequency (130 mm, 40 min) and microwave (700 W, 90 s), respectively. Results showed that the properties of TNS underwent modifications to different extents by the non-thermal and four thermal treatments. Dielectric barrier discharge plasma caused much weaker effects on the starch structures than the thermal treatments, and the dielectric barrier discharge plasma-treated starch showed the highest final viscosity, apparent rheological viscosity, and freeze–thaw stability among them. However, thermal treatments increased the RS3-type resistant starch compared to dielectric barrier discharge plasma treatment. According to the above findings, the non-thermal and thermal treatment may have a potential to modify TNS for different end-use purposes.
5.2 Chemical modifications
TNS has been subjected to esterification, cross-linking, carboxymethylation, and oxidation.63,64,78 Lv et al.63 prepared esterified TNS with citric acid. The degree of substitution (DS) of esterified TNS varied from 0.033 to 0.124. The increase in DS led to a gradual decrease in the solubility and swelling power and a marked increase in the proportion of RS (from 24.23% to 85.34%). The esterification also enhanced the capability of the starch to stabilize Pickering emulsion. Olayemi et al.64 investigated the properties of TNS cross-linked with citric acid. Results showed that the pH of the cross-linked starches was lower (3.39–4.07) than that of the raw starch (5.25). The water-holding capacity of the starch was improved from 85.55% to 96.69% by citric acid cross-linking, whereas its emulsion capacity (15.33%) was similar to that of the raw starch (15.33%). It was noted that the cross-linked starch was found to have an improved flow profile and better compactness during tablet compression, which suggested that it may have potential as a binding agent in pharmaceutical industries. Neto et al.29 chemically modified TNS with octenyl succinic anhydride. The results showed that the modified starch was more resistant to heat and the gelatinization temperature increased from 62.27 °C to 104.76 °C, as depicted by differential scanning calorimetry curves. Si et al.79 prepared cross-linked TNS by using sodium trimetaphosphate as the agent. Results showed that, with the increase in phosphorus content, the water holding capacity increased from 89.04% to 97.86%, the oil holding capacity decreased from 73.75% to 69.99% and the RS content increased from 38.38% to 64.33%, while the transparency of the paste reduced from 8.00% to 1.33%. Besides, sodium trimetaphosphate cross-linking markedly restricted the gelatinization of starch. The cross-linked starch did not gelatinize when the starch was cross-linked with 2.0% sodium trimetaphosphate. Zheng et al.78 prepared modified TNS by carboxymethylation. Results showed that the carboxymethylated TNS had greater viscosity, better paste transparency and higher freeze–thawing stability than the native TNS. Han et al.80 prepared oxidized TNS using sodium hypochlorite. They found that the water binding capacity of the oxidized starch decreased from 81.70% to 64.00% with increasing the content of sodium hypochlorite from 1.5% to 6%. Han et al.81 prepared octenyl succinic esters of granular TNS by the aqueous phase method (pH 8.5, 2 h, 35 °C). Compared with the raw starch, starch esterification led to an increase in RS content (from 0.39 to 0.50 g g−1) and contact angle (from 31.88° to 47.72°). The findings indicated that octenyl succinic esters of granular TNS had potential use as Pickering stabilizer in foods.
5.3 Enzymatic modifications
Some researchers conducted enzymatic modifications on TNS. Enzymatic modifications have been commonly utilized due to high specificity, high selectivity and environmental friendliness. Li et al.14 modified the TNS with pullulanase (0–100 U, pH 5.0, 40 °C, 2 h). The enzymatic treatment significantly lowered the in vitro digestibility, i.e., the SDS content was increased from 1.24% to 22.92% and the RS content was increased from 0.79% to 6.20%. Moreover, pullulanase-modified starch showed sharply lower consistency index (K) and yield stress (τ0) in the steady shear rheological test and smaller values of storage modulus (G′) and loss modulus (G′′) in the dynamic rheological test than the raw starch. Except for a single enzyme mode, compound enzyme treatment has also been used to modify the TNS. Liu et al.82 reported the preparation of tiger nut RS by a series of operations, including gelatinization, thermostable α-amylase hydrolysis, pullulanase debranching, retrogradation, purification and drying. The highest content of RS (57.72%) can be obtained at an appropriate amount of enzyme addition (α-amylase 2 U g−1, pullulanase 15 U g−1), enzymatic hydrolysis time (α-amylase 15 min, pullulanase 20 h), and enzymatic hydrolysis temperature (α-amylase 95 °C, pullulanase 50 °C). Bao et al.83 prepared porous starch of tiger nut by α-amylase and glucoamylase. Compared with the raw starch, the oil absorption rate increased from 76.95% to 167.10% and the water absorption rate increased from 63.55% to 139.30%, respectively. Besides, the enzymatic treatment lowered the RS content from 24.82% to 17.30%.
5.4 Dual modifications
Dual modification is a way of combining various single modification methods in order to optimize the functionalities of raw starch for applications in the food and non-food industries. Recently, dual modification has been applied to improve the properties of TNS. Yan et al.53 prepared TNS nanoparticles by citrate esterification and ultrasound treatment. As the ultrasonic treatment time increased, the mean size and PDI of the particles decreased gradually. By optimization, they obtained starch nanoparticles with a mean size and PDI of 352.8 nm and 0.292, respectively. Wang et al.45 prepared Cyperus esculentus porous starch with α-amylase and amyloglucosidase and then performed the cross-linking of C. esculentus porous starch with sodium phytate. The surface areas of raw starch, porous starch and cross-linked porous starch were 0.0084 m2 g−1, 1.7303 m2 g−1, and 2.7734 m2 g−1, respectively. Besides, the adsorption capacity of porous starch and cross-linked porous starch was 1.3606 g g−1 and 1.2744 g g−1, respectively, which were significantly higher than 0.5419 g g−1 of the raw starch. Yan et al.47 prepared the starch–palmitic acid complex nanoparticles by TNS with enzymatic hydrolysis for different time durations and then compounded it with palmitic acid. They obtained starch–palmitic acid complex nanoparticles with a mean size of 500–567.2 nm. As the enzymatic hydrolysis time increased, the size distribution of the nanoparticles became more uniform. The results also indicated that the starch–palmitic acid complex nanoparticles had the potential as stabilizer for Pickering emulsions.
5.5 Modification of TNS by directly treating the tubers of tiger nut
To some extent, the properties of TNS would be altered during the pre-treatment of tiger nut tubers. Ocloo et al.84 prepared the dried tiger nut tubers, irradiated at 0.0, 2.5, 5.0 and 10.0 kGy, and then milled into flours. Results showed that irradiation did not significantly (P > 0.05) affect water and oil absorption capacities, SP, and bulk density of the resultant flours. However, SOL significantly (P < 0.05) increased but pasting viscosities significantly decreased with the increase in irradiation dose. The alteration of starch properties could be mainly attributed to irradiation-induced starch degradation, i.e., the formation of carboxylic acid moieties and breakdown in glycosidic linkages. Miao et al.26 investigated the influence of tiger nut tuber roasting on the structural and functional properties of TNS. Results showed that roasting altered the crystalline structure, A-chain of amylopectin, and average chain length (CLave) of the starch. As the roasting temperature increased from 105 °C to 150 °C, relative crystallinity decreased from 16.43% to 15.54%, and CLave decreased from 19.57 to 19.04. Consequently, the breakdown viscosity, consistency coefficients and syneresis of the paste decreased, indicating that roasting pre-treatment to provide a better application in starch-based frozen foods. The decrease in syneresis indicated the starch gel showed stronger binding with water, which was ascribed to an increase in short-branched chains by the roasting pre-treatment. Besides, recent studies have focused on the effects of different oil extraction processes on the property of TNS.2 Liu et al.32 compared the influences of different oil extraction techniques (hexane extraction, hot pressing, cold pressing, and subcritical fluid extraction) on the physicochemical properties of the starches in tiger nut meals. All of the oil extraction processes increased the SOL, SP and paste clarity of TNS, whereas they obviously decreased the freeze–thawing stability in response to syneresis of the starches. Similarly, Miao et al.31 investigated the effects of the cold-pressing process during oil extraction on the properties of starch from tiger nut tuber press cakes. Results showed that cold-press pre-treatment had little effect on the granular size and molecular structure of TNS, while the syneresis and storage modulus of the starch gel (6%, w/v) increased slightly. These findings are promising for the recovery of both oil and starch from the tuber of tiger nut. Furthermore, Miao et al.34 studied the influence of extrusion pre-treatment prior to oil extraction on the structure and functional properties of starch from tiger nut meals. Extrusion conditions, i.e., barrel temperature and feed moisture, were varied in the range of 100–160 °C, 7–15%, respectively. Results showed that extrusion slightly reduced the relative crystallinity (20.6% → 19.2%) and increased the ratio of B2-chains (13.31% → 15.52%) in amylopectin. These changes resulted in reductions in peak viscosity from 4443 mPa s to 4121 mPa s while improving the thermal stability of the paste due to the sharp decline in the breakdown viscosity. Moreover, extrusion led to a significant increase in the final viscosity of the paste and the elastic properties of the starch gel.
5.6 Modification of TNS by blending with other additives
The modifications to the starch occur in the course of various processing operations due to the interactions of starch with native and added food constituents such as carbohydrates, proteins, lipids, salts, polyphenols, surfactants, etc., resulting in much altered physicochemical characteristics of starches and their functional properties.85 Liu et al.35 found that the functional and rheological properties of TNS were influenced by incorporating Chinese quince seed gum. Chinese quince seed gum markedly increased the peak, breakdown, final, and setback viscosities. Besides, the partial substitution of TNS with the gum improved the freeze–thaw stability of the gel. The study showed a promising way to enhance the properties of TNS instead of using chemical additives.
6 Uses of tiger nut starch
TNS is underutilized and considered a novel starch resource. Up to now, TNS in native and modified forms has presented some applications in the food industry, such as dispersing agents, texture improvers, ingredients for composite flour, and gelling/thickening agents. TNS could function as tablet excipients for pharmaceutical purposes. In addition, TNS has been processed into resistant starch and nanostarch with potential functionalities. In this section, the current applications of TNS in various food and non-food industries are summarized.
6.1 Food uses
6.1.1 Ingredient for horchata de chufa.
“Horchata de chufa”, or shortly called as “horchata”, is a traditional Spanish beverage produced from tiger nut tubers. It is a non-alcoholic, low-acid, milk-like drink with a sweet taste.21 Raw horchata has a pH range of 6.3–6.8 and is rich in starch (2–3 g/100 mL). The conventional “horchata” processing includes soaking (dried tubers only), grinding, pressing (extraction under pressure), sieving and mixing with sugar (10–12 g/100 mL) and cooling.21 Traditionally, horchata is consumed in households. To date, a well-developed industry has been established in Spain and other countries. Industrial-scale production requires storage, downstream processing, and quality control. Most of all, commercial products must achieve physicochemical, organoleptic and microbiological stability within their shelf life. Empirical evidence indicates that the fresh natural horchata should not be heated above 72 °C due to starch gelatinization and the subsequent adverse change of the sensory properties.86 However, the retention of starch granules has been shown to be necessary, for the removal of starch granules leads to the loss of the natural organoleptic properties of horchata. Under such circumstances, non-thermal processing technologies have been assessed for the development of stored horchata products.86 A series of works showed that pulsed electric fields were applicable to increase the shelf life of horchata while maintaining its organoleptic properties.87 Besides, horchata generally has deposits of starch granules, indicating that improvement of dispersion stability is required for stored products.88 Regarding the extraction of horchata, Kizzie-Hayford et al.88 reported that the duration of milling improved the colloidal stability of the product. In their work, an increase in the proportion of insoluble starch granules was observed. The wet-milling made starch granules with a less defined structure, which enhanced the dispersion stability, and consequently, improved the shelf life of the product. Codina-Torrella et al.89 applied ultra-high pressure homogenization (UHPH) to improve the physicochemical stabilization of horchata. They found that UHPH-treated horchata showed better colloidal stability than the conventional treated samples.
6.1.2 Ingredient for baked stuff.
Bakery products are very popular worldwide. Although wheat flour is a vital ingredient in bakery products, flours and starches from other cereals, tubers, and legumes are substantially investigated as new alternatives from sustainable sources to wheat in bakery foods due to various purposes such as improving the structure, mouth feel, acceptability, and nutritional properties.90 Chinma et al.13 investigated the effect of tiger nut flour addition on the quality of wheat-based cakes. The weight and volume of cakes increased with tiger nut substitution level increasing, while batter density decreased. Acceptable cakes could be made with up to 30% tiger nut flour substitution. Further, Chinma et al.91 studied the effect of germinated tiger nut and Moringa flour addition on the quality of wheat-based bread. There was no significant (P ≥ 0.05) difference in sensory properties between 100% wheat bread and composite bread. However, the blended flour improved the nutritional quality of the bread. Kizzie-Hayford et al.92 reported that substituting wheat flour with tiger nut flour (10–30%) increased bread brownness and colour saturation but decreased lightness and bread-specific volume. The addition of an appropriate amount of tiger nut flour (10% for butter bread; 25% for tea bread) led to significantly higher consumer scores of overall acceptability compared with 100% wheat flour-based samples. Kizzie-Hayford et al.10 substituted wheat flour with a 9 day-sprouted tiger nut flour for bread. Consumer acceptance of the sprouted tiger nut bread was comparable to wheat flour bread. However, the incorporation of sprouted tiger nuts added more nutrients to bread. Besides, tiger nut flour had been explored for the making of gluten-free bread.93 Aguilar et al.94 found that although tiger nut flour reduced bread-specific volume, the bread elaborated with chickpea and tiger nut flour maintained its baking characteristics even when shortening and/or emulsifiers reduced or eliminated. Demirkesen et al.93 found that microwave-infrared baking could produce gluten-free bread containing tiger nut flour and rice flour (20
:
80) with a crust colour similar to conventionally baked bread. Some researchers explored the potential of tiger nut flour in the preparation of biscuits, cookies, and snacks.95–97 Chinma et al.13 formulated blends of tiger nut and pigeon pea flours to prepare biscuits. The biscuits had poorer starch digestibility (25.43% to 44.18%) than 100% wheat flour-based biscuits (57.25%). Awolu et al.95 reported that maize-based snacks supplemented with soy and tiger nut flour were nutritious and of good overall acceptability. Dada et al.98 showed that biscuits from the flour blends of wheat (70%), African yam bean (15%) and tiger nut (15%) had acceptable sensory properties. Çinar et al.12 concluded that rice flour substituted with different ratios of tiger nut flour (0%, 10%, 20%, 30%, 40%, and 50%) was applicable for the preparation of gluten-free cookies.
6.1.3 Ingredient for noodles and pasta.
To date, no study has focused on the direct application of isolated TNS as an ingredient in noodles and pasta. However, tiger nut flour could be incorporated into wheat flour at different levels for the production of pasta or gluten-free noodles.99,100 Albors et al.101 indicated that durum wheat semolina replaced by 30% (w/w) of tiger nut flour had fair techno-functional properties and the resultant tiger nut tagliatelle were of acceptable sensory quality compared to traditional pasta (100% durum semolina). Moreover, the 30% substitution level of tiger nut flour ensured the product had more than 3% fibre content. Besides, Llavata et al.11 developed a gluten-free pasta with a composite flour of tiger nut, chickpea and fenugreek. Gasparre and Rosell99 prepared gluten-free noodles with tiger nut flour, using hydrocolloids as a modifier. Hydrocolloids increased dough consistency, resulting in fresh noodles with higher diameter and lower cooking losses as well as cooked noodles with greater hardness and firmness. Similarly, Martín-Esparza et al.100 applied hydrocolloids (carboxymethyl cellulose, xanthan gum, and locust bean gum) to improve the cooking properties and quality attributes of fresh tagliatelle based on tiger nut flour and durum wheat semolina. The results showed that employing xanthan gum at a concentration of about 0.6% would prepare ready-to-eat, fresh pasta with better textural characteristics.
6.1.4 Ingredients for low glycemic-index foods.
The digestibility of starch is related to the nutritional values and health functions of final products. The low digestible starches, i.e., SDS and RS, have gained considerable interest in recent decades. The intake of low-digestible starches showed a low glycemic response and other potential health benefits.14,36 Wang et al.36 gathered the results from in vivo digestion and indicated that native TNS had a lower glucose index than normal wheat starch. Their work also suggested that the production of the RS component in TNS could be achieved by gelatinization–retrogradation processing. Li et al.14 reported that the SDS and RS contents of TNS reached the maximum (22.92% and 6.20%, respectively) under pullulanase hydrolysis. Bian et al.55 reported that the RS content of raw TNS was 20.52%, and when the TNS was modified by different methods, i.e., autoclaving, enzymatic debranching and a combination of autoclaving and debranching, the RS content soared to 68.63%, 77.05%, and 81.26%, respectively. Their estimated glycemic index (GI) was 39.83–39.86, which indicated that modified TNS could be applied in low GI foods (GI < 55). Oluwajuyitan et al.102 dried the tubers of tiger nut in an oven and milled them into flour. They found that formulated dough meals containing 11.5% tiger nut flour, 51.07% plantain flour and 37.43% defatted soybeans flour showed better blood glucose-reducing potential than traditional plantain-based dough meals. The composite flour and the resultant dough meals could be suitable for diabetic patients. Llavata et al.11 reported that the composite tiger nut flour and chickpea (50
:
50, w/w) showed good dough machinability for the gluten-free pasta preparation, and the pasta had good sensory acceptance and obtained an estimated glycaemic index (eGI) of under 55, thus, classified as a low GI food.
6.1.5 Ingredient for meat products.
Starch is widely used in meat products as a quality enhancer.103,104 So far, there is limited study on the applicability of TNS in meat products. Baioumy et al.105 applied tiger nuts to low-fat patties. In the formulated beef patties, animal fat was replaced with a mixture of tiger nut and quinoa seeds (hydrated form 1
:
1, w/w) at 10%. The fat content of the formulated patties was only 8.87%, much lower than the control (18.26%). However, the patties (grilled) got 0.3 points higher overall acceptability than the control. More recently, Huang et al.106 investigated the performance of TNS in a coating batter on Chinese crispy meat. The coating batter was made of TNS : wheat flour (2
:
1; w/w) and liquid egg. Results showed that Chinese crispy meat cooked with TNS had a good expanding effect, crispness and tenderness.
6.1.6 Other food applications.
In the food industry, starch is used as versatile materials or additives for rheological, textural, nutritional and preservative purposes in baked foods, pasta, confectionaries, soups and sauces.23,27 The TNS could yield cooked pastes with good consistencies, which was a good choice for use as a thickener or binder in food products, such as soups and dessert powders. The TNS paste showed good thawing stability and gelling properties, which were applicable to frozen food and jellies.16,23,36 Lv et al.16 evaluated the applicability of TNS in steamed bread making. The addition of TNS reduced the specific volume of steamed bread. However, it could significantly enhance the chewiness and crumb structure of steamed bread when the added amount was appropriate. Interestingly, the structure of the steamed bread crumbs was markedly improved when 50% wheat flour was replaced by TNS. In addition, TNS can be used as starch-based bioactive films and fillers on chitosan films. Li et al.18 developed bioactive films based on TNS incorporating thymol (0%, 1%, 2%, and 3%) by the solution casting method. The TNS showed good film-forming properties and good compatibility with thymol. Interactions between the starch and thymol were mainly through hydrogen bonds. The addition of thymol did not affect the water vapour permeability but markedly enhanced the DPPH radical scavenging activity of the film. Besides, the film showed UV shielding properties. Results indicated that the film was an alternative for materials in food packaging.
6.2 Non-food uses
6.2.1 Excipients for pharmaceutical preparations.
Starch is included in the GRAS (Generally Recognized as Safe) list of the World Health Organization and is one of the most commonly used excipients in pharmaceutical formulations. Starches have been applied in the pharmaceutical industry as binders, disintegrants, diluents (filler), lubricants, glidants, and tablet coating materials.107 Thus, the application of TNS in the pharmaceutical industry has also been explored. Manek et al.27 reported that TNS conformed well to the United States Pharmacopeia standards established for widely used starches like corn and potato. The TNS might have comparable flow properties with respect to maize and potato starch, as indicated by Carr's index and Hausner ratio. The TNS was employed as a binder for the formulation of metronidazole tablets, which showed better hardness and negligible friability as compared to those with potato starch. Builders et al.52 reported that TNS has the potential as an efficient excipient for direct compression of tablets. Once the TNS was treated by acid gelation and accelerated retrogradation, TNS as an excipient showed enhanced direct compression, flow and disintegrant properties compared with microcrystalline cellulose (as the standard excipient). Builders et al.71 assessed the binder properties of TNS, and they prepared ascorbic acid granules and tablets by wet granulation using the pastes of TNS as the binder. Results showed that the ascorbic acid granules were free-flowing (Cars index 22.2%; Angle of repose 18.40°). The granules were compressed to form ascorbic acid tablets. The tablets had good mechanical strength (friability 7.9%) and showed almost complete dissolution within 10 min. Agboola et al.17 evaluated the glidant property of TNS in comparison with purified talc and aerosil in metronidazole tablet formulation. The results showed that TNS could be suitable as a glidant in pharmaceutical tablet formulations because it improved the flow ability of granules used for the tablet formulations, thereby enhancing the production process and outcome. Okorie et al.108 reported that TNS could be used in pharmaceutical suspensions as a dispersion agent. Olayemi et al.109 investigated the TNS-alginate microbeads in the oral delivery of ibuprofen. The microbeads were made using an ionotropic gelation technique with calcium chloride solution and sodium alginate solution containing raw TNS and its cross-linked derivative. Results showed that raw and cross-linked starch improved the entrapment efficiency and mucoadhesive ability of the microbeads, which could have potential for sustained drug delivery and targeted delivery to the lower gastrointestinal tract. Conclusively, the TNS has potential as an excipient, binder, filler, glidant and suspending agent for formulating tablets or other pharmaceutical preparations.
6.2.2 Preparation of starch nanoparticles and uses.
Structurally, natural starch granules contain nano-sized semi-crystalline blocklets. The nano-blocklets could be isolated from the granule by physical treatments or mild hydrolysis with inorganic acids and/or enzymes.110 The obtained resultants are the so-called nano starch, and commonly, starch nanoparticles. Yan et al.53 prepared citrate-esterified TNS and made it into nanoparticles by ultrasound treatment. By the ultrasonic treatment time of 5 min, the prepared starch nanoparticles had a mean size and PDI of 352.8 nm and 0.292, respectively. The starch nanoparticles showed an A-type crystalline structure and good stability, as indicated by the XRD pattern and zeta-potential measurement. Yan et al.47 prepared nanoparticles of TNS–palmitic acid complex in two steps, i.e., enzymatic hydrolysis for a different time and then forming a complex with palmitic acid. The mean size of nanoparticles ranged from 500 nm to 567.2 nm. When the enzymatic hydrolysis time increased from 0 h to 4 h, the size distribution became more uniform, and the relative crystallinity increased from 14.99% to 47.72%. The diffraction peaks also indicated that two kinds of nanoparticles were formed, i.e., nanoparticles of amylose–palmitic acid complex and starch nanoparticles by ethanol precipitation. Enzymatic hydrolysis led to the formation of more amylose–palmitic acid complex nanoparticles due to an increase in amylose content from 27.01% to 52.63% by pullulanase treatment.47 In another paper, Yan et al.15 made TNS nanoparticles using pullulanase debranching and ethanol nanoprecipitation. Results showed that the nanoparticles became smaller and more uniform with the increasing ethanol ratio. The particle size attained 271.1 nm when the volume ratio of starch solution/ethanol was 1/5. Wang et al.51 prepared tiger nut nano starch by successive procedures, including gelatinization, ultra sonication and nanoprecipitation. Compared to native starch, the nano starch had a higher amylose content (39.05%) and increased by 15% compared with the native starch (24.10%).
Recently, the application of tiger nut nano starch has been explored. Yan et al.15 showed that in vitro digestibility (the RS contents 13.15–15.28%) of TNS nanoparticles prepared via debranching and nanoprecipitation was markedly greater than the native TNS (2.80%). Wang et al.51 reported that the RS contents of native and nano TNS were 33.53% and 40.38%, respectively. These interesting findings indicated the potential application of tiger nut nano starch in food and nutraceutical sectors. Yan et al.53 reported that the mechanical properties of chitosan-based films were significantly enhanced by adding citrate-esterified nanoparticles of TNS. The result implied that TNS nanoparticles could be further explored as fillers in composite materials. Besides, TNS nanoparticles could be used as emulsion stabilizers. Yan et al.47 found that starch–palmitic acid complex nanoparticles were capable of stabilizing Pickering emulsions. After 42 days of storage, the mean size and PDI of the Pickering emulsion changed insignificantly (P > 0.05). Moreover, the potential of starch–palmitic acid complex nanoparticles for encapsulation of bioactive compounds was proposed by the authors. More recently, Wang et al.51 also proposed OSA nano starch from tiger nuts as nanocapsules and emulsifying agents for the preparation of nanoemulsions. Nonetheless, nano starches are versatile and show numerous applications. Due to remarkable interfacial, stabilizing, rheological, encapsulating and nutritional properties, it is worth studying the applications of tiger nut nano starch such as packaging materials, emulsion stabilizers, nutraceutical nanocarriers, fat substitutes, and even cryoprotective agents in the future.111
7 Conclusions
The production of tiger nut is expected to rise. Tiger nut contains about 30% of starch, which is a particularly useful source of starch. Raw TNS granules are mostly spherical or elliptical, with a minority being irregular. The particle size of TNS varies from 2 to 18.53 μm with an average of 8.6 μm. The amylose content of TNS has been reported to be 9.71–27.01%. TNS has the greatest proportion of the B1 chain at DP 13–24. TNS shows good paste clarity and freeze–thaw stability. The gel strength of TNS is comparable to corn and potato starches. Raw, gelatinized and retrograded TNS are good sources of low-digestible starches.
To date, various physical, chemical, enzymatic and dual modifications have been employed to widen the range of functionalities of TNS. Collected data present the possible uses of starch from this sustainable plant in the food and non-food industries. Raw TNS is a necessary constituent in the popular vegetable beverage “horchata”. TNS and tiger nut flour are used in various foods such as baked stuff (bread, cakes, biscuits, and cookies), pasta and noodles, and even meat products. TNS is a promising material for RS and has the potential for developing low composite flour glycaemic-index foods. TNS also has a place in pharmaceutical preparations such as tablets and suspensions. In addition, tiger nut nano starch has unique characteristics and potential applications.
In order to expand the uses of TNS and to deeply understand their physical and chemical nature, the following topics are suggested to be addressed: (1) TNS varies in amylose content and XRD pattern. Therefore, the polymorph of TNS as affected by the cultivar genetics and growing conditions need to be conclusively studied. (2) Some structural aspects of TNS remain unknown. The fine structures of amylose and amylopectin of TNS and the relationship of chemical and molecular structure to physicochemical properties should be studied. (3) The development of TNS and tiger nut flour products for specific purposes should be explored. Namely, methods to increase the low digestible starch contents are worth developing. Thus, the modified starches can be applied to produce foods with low glycemic index. Besides, interactions of TNS with other food ingredients and/or additives, which subsequently affect the properties of TNS, are required to be investigated. In the end, the application of TNS in food and non-food industries is expected to attract more attention.
Author contributions
Yuchen Wu: writing – original draft preparation; Qihui Mao: writing – review and editing; Guohua Zhao: writing – review and editing, funding acquisition and supervision; Fayin Ye: conceptualization, writing – original draft preparation, writing – review, and editing, funding acquisition and supervision.
Conflicts of interest
There are no conflicts to declare.
Acknowledgements
This research was funded by the National Natural Science Foundation of China (32272239) and National Key Research and Development Program of China (2021YFD2100101).
References
- X. Yang, L. Niu, Y. Zhang, W. Ren, C. Yang, J. Yang, G. Xing, X. Zhong, J. Zhang, J. Slaski and J. Zhang, Plants, 2022, 11, 923 CrossRef CAS PubMed.
- E. Roselló-Soto, M. M. Poojary, F. J. Barba, J. M. Lorenzo, J. Mañes and J. C. Moltó, Innovative Food Sci. Emerging Technol., 2018, 45, 306–312 CrossRef.
- E. Sánchez-Zapata, J. Fernández-López and J. Angel Pérez-Alvarez, Compr. Rev. Food Sci. Food Saf., 2012, 11, 366–377 CrossRef.
- I. Codina-Torrella, B. Guamis and A. J. Trujillo, Ind. Crops Prod., 2015, 65, 406–414 CrossRef CAS.
- X.-K. Zhang, Sci. China Technol. Sci., 2019, 287, 67–69 Search PubMed.
- Y. Zhang and S. Sun, Food Chem.: X, 2023, 19, 100868 CAS.
- J. J. L. Bezerra, B. F. Feitosa, P. C. Souto, A. A. V. Pinheiro and B. Agri, Biotechnology, 2023, 47, 102606 CAS.
- G. I. Edo, F. O. Onoharigho, A. N. Jikah, G. O. Oloni, P. O. Samuel, O. A. Rapheal, O. Ikpekoro, P. O. Akpoghelie, J. J. Agbo, H. A. Ekokotu, U. Ugbune, G. O. Ezekiel, G. A. Abere, E. E. A. Oghroro, A. E. Ojulari, K. A. Okoronkwo, J. O. Owheruo and E. O. Akpoghelie, Food Chem. Adv., 2023, 3, 100511 CrossRef.
- P. A. Asare, R. Kpankpari, M. O. Adu, E. Afutu and A. S. Adewumi, Sci. World J., 2020, 1–11, DOI:10.1155/2020/7232591.
- N. Kizzie-Hayford, E. E. Abano, J. Akanson, E. Dankwa, H. Rohm and J. Ampofo-Asiama, J. Food. Sci., 2023, 88, 3681–3693 CrossRef CAS PubMed.
- B. Llavata, A. Albors and M. E. Martin-Esparza, Foods, 2019, 9, 11 CrossRef PubMed.
- A. T. Çinar, E. Turabi Yolaçaner and E. G. Ateş, J. Sci. Food Agr., 2023, 103, 5342–5352 CrossRef PubMed.
- C. E. Chinma, J. O. Abu and Y. A. Abubakar, Int. J. Food Sci. Technol., 2010, 45, 1746–1752 CrossRef CAS.
- X. Li, J. Fu, Y. Wang, F. Ma and D. Li, Int. J. Biol. Macromol., 2017, 102, 651–657 CrossRef CAS PubMed.
- X. Yan, M. Diao, Y. Yu, F. Gao, E. Wang, Z. Wang, T. Zhang and P. Zhao, Food Chem., 2022, 369, 130824 CrossRef CAS PubMed.
- X. Lv, S. Zhang, S. Zhen, Y. Shi and B. Liu, J. Food Process. Preserv., 2022, 46, e16792 CAS.
- B. S. Agboola, T. O. Ajala and O. A. Odeku, Starch, 2017, 70, 170004 Search PubMed.
- F. Li, S. Mao, X. Zhou, S. Li, C. Lu and T. Zhang, Int. J. Food Sci. Technol., 2023, 58, 4446–4454 CrossRef CAS.
- G. C. Akabassi, K. K. Palanga, E. A. Padonou, Y. D. Dagnon, K. Tozo and A. E. Assogbadjo, Genet. Resour. Crop Evol., 2022, 69, 1979–1992 CrossRef.
- S. Follak, R. Belz, C. Bohren, O. De Castro, E. Del Guacchio, N. Pascual-Seva, M. Schwarz, F. Verloove and F. Essl, Pl. Ecol. Evol. Syst., 2016, 23, 33–51 CrossRef.
- E. Roselló-Soto, M. M. Poojary, F. J. Barba, M. Koubaa, J. M. Lorenzo, J. Mañes and J. C. Moltó, Food Res. Int., 2018, 105, 945–951 CrossRef PubMed.
- H. Turesson, S. Marttila, K. E. Gustavsson, P. Hofvander, M. E. Olsson, L. Bülow, S. Stymne and A. S. Carlsson, Am. J. Bot., 2010, 97, 1884–1893 CrossRef PubMed.
- S. C. Umerie, N. A. N. Obi and E. O. Okafor, Bioresour. Technol., 1997, 62, 63–65 CrossRef.
- P. T. Akonor, C. Tortoe, C. Oduro-Yeboah, E. A. Saka and J. Ewool, Int. J. Food Sci., 2019, 1–7, DOI:10.1155/2019/3830651.
- S. Jing, X. Yan, W. Ouyang, H. Xiang and Z. Ren, Starch, 2012, 64, 581–589 CrossRef CAS.
- W.-B. Miao, S.-Y. Ma, X.-G. Peng, Z. Qin, H.-M. Liu, X.-S. Cai and X.-D. Wang, LWT--Food Sci. Technol., 2021, 151, 112149 CrossRef CAS.
- R. V. Manek, P. F. Builders, W. M. Kolling, M. Emeje and O. O. Kunle, AAPS PharmSciTech, 2012, 13, 379–388 CrossRef CAS PubMed.
- Z. Liu, S. Tian, C. Lv and Z. Chen, BioResources, 2022, 18, 60–72 Search PubMed.
- J. Costa Neto, R. d. Silva, P. Amaral, M. R. Leão, T. Gomes and G. Sant'Ana, Polímeros, 2018, 28, 319–322 CrossRef.
- H.-M. Liu, Y.-Y. Yan, X.-X. Liu, Y.-X. Ma and X.-D. Wang, Int. J. Biol. Macromol., 2020, 156, 144–152 CrossRef CAS PubMed.
- W. B. Miao, Y. J. Li, S. Y. Ma, J. H. Jiang, H. M. Liu, X. S. Cai, Z. Qin and X. D. Wang, Int. J. Food Sci. Technol., 2021, 57, 662–675 CrossRef.
- X.-X. Liu, H.-M. Liu, J. Li, Y.-Y. Yan, X.-D. Wang, Y.-X. Ma and G.-Y. Qin, Food Hydrocolloids, 2019, 95, 262–272 CrossRef CAS.
- R.-Y. Zhang, C. Liu, P.-X. Chen, M.-M. Jiang, W.-X. Zhu and H.-M. Liu, J. Food Meas. Charact., 2023, 17, 4357–4370 CrossRef.
- W.-B. Miao, Z.-W. Wu, J.-H. Jiang, Y.-J. Li, Z. Qin, H.-M. Liu, X.-S. Cai and X.-D. Wang, Carbohydr. Polym., 2022, 298, 120152 CrossRef CAS PubMed.
- H.-M. Liu, W.-B. Miao, R. Wang, N. Chen, S.-Y. Ma and X.-D. Wang, LWT--Food Sci. Technol., 2021, 143, 111180 CrossRef CAS.
- G. Wang, C. Li, X. Zhang, Q. Wang, R. Cao, X. Liu, X. Yang and L. Sun, Int. J. Biol. Macromol., 2023, 253, 126626 CrossRef CAS PubMed.
- H.-Z. Tan, Z.-G. Li and B. Tan, Food Res. Int., 2009, 42, 551–576 CrossRef CAS.
- M. Li, S. Dhital and Y. Wei, Compr. Rev. Food Sci. Food Saf., 2017, 16, 1042–1055 CrossRef CAS PubMed.
- T. Sánchez, E. Salcedo, H. Ceballos, D. Dufour, G. Mafla, N. Morante, F. Calle, J. C. Pérez, D. Debouck, G. Jaramillo and I. X. Moreno, Starch, 2009, 61, 12–19 CrossRef.
- A. A. Wani, P. Singh, M. A. Shah, U. Schweiggert-Weisz, K. Gul and I. A. Wani, Compr. Rev. Food Sci. Food Saf., 2012, 11, 417–436 CrossRef CAS.
- N. Singh, J. Singh, L. Kaur, N. Singh Sodhi and B. Singh Gill, Food Chem., 2003, 81, 219–231 CrossRef CAS.
- C. Cheng, J. Hu, Y. Zhi, J. J. Su, X. K. Zhang and B. Q. Huang, Genet. Mol. Res., 2015, 14, 18302–18314 CrossRef CAS PubMed.
- P. Vanhung and N. Morita, Food Chem., 2007, 105, 749–755 CrossRef CAS.
- O. K. Abegunde, T.-H. Mu, J.-W. Chen and F.-M. Deng, Food Hydrocolloids, 2013, 33, 169–177 CrossRef CAS.
- J. Wang, D. Zhang, J. Xiao and X. Wu, Foods, 2022, 11, 2583 CrossRef CAS PubMed.
- W.-B. Miao, Y.-Y. Ning, H.-R. Huang, H.-M. Liu, X.-S. Cai and X.-D. Wang, Arabian J. Chem., 2021, 14, 103407 CrossRef CAS.
- X. Yan, M. Diao, C. Li, C. Lu, P. Zhao and T. Zhang, Int. J. Biol. Macromol., 2022, 204, 685–691 CrossRef CAS PubMed.
- M. Abdel-Akher and A. N. Michalinos, Starch, 1963, 15, 329–334 CrossRef.
- S. Dhital, A. K. Shrestha and M. J. Gidley, Carbohydr. Polym., 2010, 82, 480–488 CrossRef CAS.
- L. Lin, J. Huang, L. Zhao, J. Wang, Z. Wang and C. Wei, Carbohydr. Polym., 2015, 134, 448–457 CrossRef CAS PubMed.
- J. Wang, R. Zhang, Z. Huang, M. Cai, W. Lou, Y. Wang, A. Gharsallaoui, H. Roubik, K. Yang and P. Sun, Food Qual. Saf., 2023, 7, 818–826 Search PubMed.
- P. F. Builders, P. A. Anwunobi, C. C. Mbah and M. U. Adikwu, AAPS PharmSciTech, 2013, 14, 818–827 CrossRef CAS PubMed.
- X. Yan, M. Diao, Y. Yu, F. Gao, E. Wang, Z. Wang and T. Zhang, Int. J. Biol. Macromol., 2021, 179, 154–160 CrossRef CAS PubMed.
- X. Cao, M.-H. Xin, T.-T. Liu, S.-S. Zhang and D.-W. Wang, J. Chin. Cereals Oils Assoc., 2022, 37(10), 131–137 Search PubMed.
- X.-J. Bian, L.-P. Ma, X.-L. Dong, Y. Wang and K.-P. Jiao, J. Chin. Cereals Oils Assoc., 2023, 38, 52–59 Search PubMed.
- X.-F. Lv, Y. Chen and W. Zhou, Food Sci. Technol., 2022, 42, 2583 Search PubMed.
- Q. Wang, L. Li, C. Liu and X. Zheng, Food Chem., 2022, 386, 132771 CrossRef CAS PubMed.
- L. Li, Y. Hong, Z. Gu, L. Cheng, Z. Li and C. Li, Food Hydrocolloid, 2018, 77, 825–833 CrossRef CAS.
- Y. Zhang, Y. Zhang, F. Xu, S. Li and L. Tan, Food Hydrocolloids, 2018, 80, 141–148 CrossRef CAS.
- C. Kowittaya and N. Lumdubwong, Carbohydr. Polym., 2014, 108, 216–223 CrossRef CAS PubMed.
- Y.-y. Kim, K. S. Woo and H.-J. Chung, Food Chem., 2018, 263, 104–111 CrossRef CAS PubMed.
- I. M. Park, A. M. Ibáñez and C. F. Shoemaker, Starch, 2007, 59, 69–77 CrossRef CAS.
- X. Lv, C. Guo, Y. Ma and B. Liu, Int. J. Biol. Macromol., 2022, 222, 2833–2842 CrossRef CAS PubMed.
- B. Olayemi, C. Y. Isimi, K. Ekere, A. Johnson Isaac, J. E. Okoh and M. Emeje, Turk. J. Pharm. Sci., 2021, 18, 34–43 CrossRef CAS PubMed.
- Y. Ai and J. l. Jane, Starch, 2015, 67, 213–224 CrossRef CAS.
- H. Wang, Q. Yang, U. Ferdinand, X. Gong, Y. Qu, W. Gao, A. Ivanistau, B. Feng and M. Liu, Int. J. Biol. Macromol., 2020, 160, 660–668 CrossRef CAS PubMed.
- J. Cai, J. Man, J. Huang, Q. Liu, W. Wei and C. Wei, Carbohyd. Polym., 2015, 125, 35–44 CrossRef CAS PubMed.
- P. Tappiban, Y. Ying, Y. Pang, S. Sraphet, N. Srisawad, D. R. Smith, P. Wu, K. Triwitayakorn and J. Bao, Int. J. Biol. Macromol., 2020, 150, 831–838 CrossRef CAS PubMed.
- W. Li, J. Gao, G. Wu, J. Zheng, S. Ouyang, Q. Luo and G. Zhang, Food Hydrocolloids, 2016, 60, 364–373 CrossRef CAS.
- C.-L. Lv, C.-Y. Zhu, Z.-Y. Dong, S.-T. Zhao, J.-X. Zuo and S.-Q. Tian, Sci. Technol. Food Ind., 2022, 43, 192–199 Search PubMed.
- P. F. Builders, C. C. Mbah, K. K. Adama and M. M. Audu, Starch, 2013, 66, 281–293 CrossRef.
- S. A. Craig, C. C. Maningat, P. A. Seib and R. C. Hoseney, Cereal Chem., 1989, 66, 173–182 CAS.
- C. O. Bernardo, J. L. R. Ascheri, D. W. H. Chávez and C. W. P. Carvalho, Starch, 2018, 70, 1700185 CrossRef.
- M. Irani, E. S. M. Abdel-Aal, S. M. A. Razavi, P. Hucl and C. A. Patterson, Cereal Chem., 2017, 94, 341–348 CrossRef CAS.
- A. Kaur, K. Shevkani, N. Singh, P. Sharma and S. Kaur, J. Food Sci. Tech., 2015, 52, 8113–8121 CrossRef CAS PubMed.
- T. H. Harris, J. M. Mansfield and D. M. Paulnock, Infect. Immun., 2007, 75, 2366–2373 CrossRef CAS PubMed.
- D. J. A. Jenkins, C. W. C. Kendall, L. S. A. Augustin, S. Franceschi, M. Hamidi, A. Marchie, A. L. Jenkins and M. Axelsen, Am. J. Clin. Nutr., 2002, 76, 266S–273S CrossRef CAS PubMed.
- G.-F. Zheng, Z.-X. Xu, B. Zhou, Z.-C. Qin and X.-W. Chen, Sci. Technol. Food Ind., 2002, 7(23), 27–29 Search PubMed.
- M.-S. Si, G.-H. Bao, N. Liu and X.-L. Wu, J. Chin. Cereals Oils Assoc., 2022, 12(37), 120–125 Search PubMed.
- S.-H. Han, H.-Y. Lee, J.-S. Kum and J.-D. Park, Korean J. Food Preserv., 2006, 13, 404–412 Search PubMed.
- M. Han, R. Fan, Y.-T. Yang, Y.-Q. Wang and X. Wu, J. Chin. Cereals Oils Assoc., 2022, 37, 75–80 Search PubMed.
- L. Liu, X.-F. Zou and H.-R. Lei, Food Sci. Technol., 2015, 40, 296–299 Search PubMed.
- G.-H. Bao, M.-S. Si and X.-L. Wu, Food Res. Dev., 2022, 43(14), 160–164 CAS.
- F. C. K. Ocloo, A. A. Okyere and I. K. Asare, Radiat. Phys. Chem., 2014, 103, 9–15 CrossRef CAS.
- H. Rostamabadi, D. Bajer, I. Demirkesen, Y. Kumar, C. Su, Y. Wang, M. Nowacka, P. Singha and S. R. Falsafi, Carbohydr. Polym., 2023, 314, 120905 CrossRef CAS PubMed.
- M. Corrales, P. M. de Souza, M. R. Stahl and A. Fernández, Innovative Food Sci. Emerging Technol., 2012, 13, 163–168 CrossRef CAS.
- C. Cortés, M. J. Esteve, A. Frígola and F. Torregrosa, Food Chem., 2005, 91, 319–325 CrossRef.
- N. Kizzie-Hayford, D. Jaros, Y. Schneider and H. Rohm, Int. J. Food Sci. Technol., 2014, 50, 381–388 CrossRef.
- I. Codina-Torrella, B. Guamis, V. Ferragut and A. J. Trujillo, Innovative Food Sci. Emerging Technol., 2017, 40, 42–51 CrossRef CAS.
- S. A. Siddiqui, M. M. C. Mahmud, G. Abdi, U. Wanich, M. Q. U. Farooqi, N. Settapramote, S. Khan and S. A. Wani, J. Food Biochem., 2022, 46, e14185 CAS.
- C. E. Chinma, J. O. Abu and S. N. Akoma, J. Food Process. Preserv., 2014, 38, 721–727 CrossRef CAS.
- N. Kizzie-Hayford, J. Akanson, E. E. Abano and J. Ampofo Asiama, Foods, 2022, 88, 381–388 Search PubMed.
- I. Demirkesen, G. Sumnu and S. Sahin, Food Bioprocess Technol., 2011, 6, 746–753 CrossRef.
- N. Aguilar, E. Albanell, B. Miñarro and M. Capellas, LWT--Food Sci. Technol., 2015, 62, 225–232 CrossRef CAS.
- O. O. Awolu, O. S. Omoba, O. Olawoye and M. Dairo, Food Sci. Nutr., 2016, 5, 3–13 CrossRef PubMed.
- E. E. Babiker, M. M. Özcan, K. Ghafoor, F. A. Juhaimi, I. A. M. Ahmed and I. A. Al, Food Chem., 2021, 349, 129–155 CrossRef PubMed.
- H. O. Agu, J. C. Ihionu, J. Musallam and C. Mba, Heliyon, 2023, 9, e15318 CrossRef CAS PubMed.
- M. A. Dada, F. A. Bello, F. O. Omobulejo and F. E. Olukunle, Heliyon, 2023, 9, e22477 CrossRef CAS PubMed.
- N. Gasparre and C. M. Rosell, Food Hydrocolloids, 2019, 97, 105–194 CrossRef.
- M. E. Martín-Esparza, M. D. Raigón, M. D. García-Martínez and A. Albors, Foods, 2021, 10, 2510 CrossRef PubMed.
- A. Albors, M. D. Raigon, M. D. García-Martinez and M. E. Martín-Esparza, LWT--Food Sci. Technol., 2016, 74, 183–190 CrossRef CAS.
- T. D. Oluwajuyitan and O. S. Ijarotimi, Heliyon, 2019, 5, e01504 CrossRef CAS PubMed.
- S. Verdú, J. M. Barat, C. Alava and R. Grau, Food Chem., 2017, 224, 69–77 CrossRef PubMed.
- G. Bortnowska, A. Krudos, V. Schube, W. Krawczyńska, N. Krzemińska and K. Mojka, Food Chem., 2016, 202, 31–39 CrossRef CAS PubMed.
- A. A. Baioumy, I. V. Bobreneva, A. A. Tvorogova and T. G. Abedelmaksoud, Int. Food Res. J., 2021, 28, 1038–1047 CAS.
- H.-R. Huang, W.-J. Cheng, S.-Q. Liao, X.-P. Jia, Z.-B. Wang, X.-S. Cai, H.-M. Liu and Y.-S. Cheng, China Food Addit., 2023, 7, 181–187 Search PubMed.
- M. A. V. T. Garcia, C. F. Garcia and A. A. G. Faraco, Starch, 2020, 72, 1900270 CrossRef CAS.
- O. Okorie, J. E. Azaka and E. Ibeshi, Am. J. Biomed., 2016, 1(1), 1–7 Search PubMed.
- O. J. Olayemi, Y. E. Apeji and C. Y. Isimi, J. Pharm. Innov., 2020, 17, 366–375 CrossRef.
- H.-Y. Kim, S. S. Park and S.-T. Lim, Colloids Surf., B, 2015, 126, 607–620 CrossRef CAS PubMed.
- S. Zihan, L. Lu, W. Tao, Z. Bolin and Z. Hongfei, Int. J. Biol. Macromol., 2023, 251, 126395 CrossRef PubMed.
|
This journal is © The Royal Society of Chemistry 2024 |