Integrated microfluidic-based ultrafine water condensation particle counter (UWCPC) for monitoring of airborne nanoparticle generation and growth mechanisms
Received
26th September 2023
, Accepted 19th March 2024
First published on 3rd April 2024
Abstract
In this study, we present an integrated microfluidic-based ultrafine water condensation particle counter (UWCPC) developed by innovatively combining lab-on-printed circuit board (PCB) and three-dimensional (3D)-printing technologies. The combined lab-on-PCB and 3D-printing technology used for UWCPC development is an optimized method for manufacturing low-cost miniaturized integrated microfluidic devices. Notably, high-precision, harmless nanoparticle measurements were realized using a sheath/sample air separator and a moderated water condensation method. This novel methodology provides a highly accurate, harmless, low-cost, miniaturized system for multipoint monitoring of airborne nanoparticle generation and growth mechanisms. Our system consisted of three main parts—a sample/sheath air separator, a nanoparticle growth sector, and a miniaturized optical particle counter (OPC)—and was rigorously evaluated using simulations to confirm its optimal design and performance. The nanoparticle growth sector consisted of PCBs and 3D-printed structures; the heater, various sensors, and super hydrophilic micro-pillar array wick necessary for nanoparticle growth were integrated on the PCBs. Through quantitative laboratory experiments, we determined that our system was capable of counting particles 3.4 nm in diameter. In addition, the role and effect of the sheath air were confirmed by measuring the particle counting efficiency according to the ratio of the sample and sheath air. Finally, high concordance was confirmed through one-to-one comparison with a reference aerosol instrument in a wide particle number concentration range (0–57
000 N cm−3). Accordingly, if sufficient field tests in the environment confirm the practical use of the developed UWCPC, the generation and growth mechanism of nanoparticles in the air can be monitored.
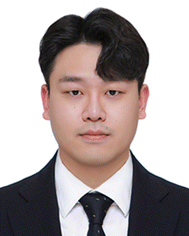 Seong-Jae Yoo | Seong-Jae Yoo received the B.S. degree from the School of Nano-Optical Engineering, Korea Polytechnic University, Republic of Korea, in 2017. He is currently a Ph.D. student at the Yonsei University, Republic of Korea. His research interests include MEMS and particle sensors. |
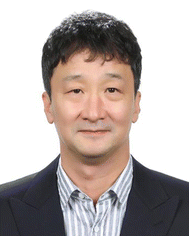 Yong-Jun Kim | Yong-Jun Kim received the B.S. degree in electrical engineering from Yonsei University, Seoul, Korea, in 1987, and the M.S. degree in electrical and computer engineering from the University of Missouri-Columbia, in 1989. He received the Ph.D. degree in electrical engineering from Georgia Institute of Technology in 1997. From 1996 to 2000, he was with Samsung Electronics Co. as a senior engineer. Since 2000, he joined the faculty of Yonsei University as a Professor in the School of Mechanical Engineering. His current research includes general micromachining, bio- and environmental sensors, and applications of sensor fusion. |
Environmental significance
Nanoparticles are more likely to penetrate the alveoli rather than be filtered into the bronchi. These nanoparticles have a large specific surface area, which increases their biological toxicity. The need for monitoring the generation and growth mechanism of nanoparticles is increasing, but high-accuracy monitoring devices that are also miniaturized and low-cost have not been developed for this purpose. In this study, an integrated microfluidic-based ultrafine water condensation particle counter was developed. Our system was capable of measuring particles as small as 3.5 nm and showed comparable performance when compared with reference instruments. Through this, we believe that utilizing our system will benefit various fields that require nanoparticle analysis such as monitoring the generation and growth mechanism of airborne nanoparticles.
|
Introduction
Airborne nanoparticles (particle diameter <100 nm) account for the majority of particulate matter (PM) number concentration and are more likely to infiltrate the alveoli without being filtered by the bronchi.1–4 These infiltrating nanoparticles are potentially more toxic than micron-sized particles and are thus regarded as more important from a health standpoint.5–7 Accordingly, the need for source tracking and the prediction of airborne nanoparticles has increased dramatically.8–10 Nanoparticles in urban areas are mainly generated from various combustion sources (e.g., gas-to-particle conversion and black carbon).11,12 In other words, to understand the mechanisms leading to the generation of new nanoparticles from combustion sources in urban areas in detail, careful measurements of the nucleation and growth (<10 nm) of atmospheric nanoparticles are required.13,14 Accordingly, to efficiently monitor nanoparticles in cities, it is necessary to track the sources of nanoparticles and predict their growth by constructing multiple monitoring systems that measure particles with a size of several nanometers. Moreover, nanoparticle monitoring is crucial not only for public health but also for enhanced yields of high-precision industries operating in cleanrooms.15,16 In the semiconductor industry, contamination by particles half the size of the minimum feature adversely affects the yield, and nanoparticle measurement has become increasingly significant since the minimum feature size has decreased to <10 nm.17,18 Unfortunately, nanoparticle contamination can occur at any manufacturing stage due to various generation sources (e.g., manufacturing equipment under operating conditions and gas-to-particle conversion via chemical reactions).19,20 Therefore, to improve yields in high-tech industries, it is necessary to build multi-point nanoparticle monitoring networks to track the source and control of contaminants in the workplace. To realize this, a portable, low-cost system capable of measuring under 10 nm sized nanoparticles is required.21
A condensation particle counter (CPC) is a widely employed device for measuring nanoparticle concentrations in diverse environments, including atmospheric research, industrial hygiene, and cleanroom monitoring.22–24 The primary reason for the widespread use of CPCs in nanoparticle measurements is their exceptional accuracy. CPCs operate by creating a controlled environment in which air is in a supersaturated state, causing the nanoparticles to condense and form micron-sized droplets.25 Subsequently, each droplet is measured using an optical counter to determine the nanoparticle number concentration. Theoretically, this CPC measurement technique can detect even a single nanoparticle, and CPCs have gradually evolved to measure smaller nanoparticles.26 Early CPCs had a minimum detectable particle size of 10 nm.27 However, because it is not possible to analyse the nucleation and growth of atmospheric nanoparticles by measuring those in the 10 nm size range, an ultrafine condensation particle counter (UCPC) close to 1 nm was developed and used in various studies.28,29 Recently, an ultrafine water condensation particle counter (UWCPC), in which alcohol as the working fluid was replaced by water, was developed, enabling harmless nanoparticle measurements in various environments.30 However, commercial CPCs, including UWCPCs, are typically expensive (minimum 10
000 USD) and large, making them unsuitable for deployment in the multi-point monitoring of airborne nanoparticle generation and growth mechanisms.
Accordingly, we first grafted technologies suitable for low-cost, integrated mass production, such as microelectromechanical systems (MEMS) and lab-on-printed circuit boards (PCBs), onto a CPC.31–33 Through this, the CPC achieved lower costs and miniaturization as well as comparable performance to commercial CPCs. The developed CPCs were general laminar flow CPCs that did not have separate parts to improve particle counting efficiency, such as sheath air supplier; therefore, they had a particle counting efficiency of around 10 nm. This allowed performance without major problems regarding the monitoring of the airborne nanoparticle number concentration. However, the monitoring of airborne nanoparticle generation and growth mechanisms was limited.
To fill the limitation of previous research, our research team developed an integrated microfluidic-based UWCPC for measuring airborne nanoparticle generation and growth mechanisms. Our system was fabricated by combining lab-on-PCB and 3D-printing technologies to ensure low cost and miniaturization of the UWCPC. Further, these two technologies are easy to access because they do not require expensive facilities such as cleanrooms, and their processes can be completed within a day. In addition, because 3D-printing can be performed at a very high resolution, it is easy to fabricate a complex and miniaturized integrated microfluidic device.34 Our system, unlike commercial UCPCs and previous CPCs developed by our research team, uses water as the working fluid and has been optimized to generate more vapor (high supersaturation state) to grow smaller nanoparticles into droplets. Also, our system enables the stable microdroplet growth of nanoparticles by applying sheath air in the nanoparticle growth sector.30 In addition, the consumption of the working fluid can be minimized using the moderated water condensation method to collect external and surplus vapor after nanoparticle growth.35 Our system was subjected to quantitative experiments and various laboratory characterization methods using reference aerosol instruments. The results showed high consistency with the reference aerosol instruments and stable operation for nanoparticles.
Experimental
UWCPC working principle
As shown in Fig. 1, the developed UWCPC was composed of a reservoir, sample/sheath air separator, nanoparticle growth sector, and miniaturized optical particle counter (OPC). The reservoir stored and supplied the working fluid (water) required for nanoparticle growth. A super hydrophilic micro-pillar array wick was configured on the wall of the reservoir and the nanoparticle growth sector, enabling a continuous working fluid supply using capillary force. The UWCPC supplied two types of air using a sample/sheath air separator: sample air containing nanoparticles and sheath air filtered using a high-efficiency particulate air (HEPA) filter. The sample and sheath air were introduced at a 1
:
1 ratio, with 0.15 liter per minute (LPM) each. The introduced sheath air envelops the sampling air, focusing it on the centerline of the channel, thus passing through regions with high saturation ratios to enhance particle growth efficiency and reduce particle diffusion losses.36 Then, the air introduced through the sample/sheath air separator reaches the nanoparticle growth sector, which is divided into three temperature sections: conditioner, initiator, and moderator. Within this section, the nanoparticles are grown into microdroplets using a moderated water condensation method based on three temperature regions. The conditioner, the first region the introduced air reaches, cools the airflow to saturation, similar to its use in a water-based condensation particle counter (WCPC).37 The initiator then uses the difference between the thermal diffusivity (0.22 cm2 s−1) and the mass diffusion coefficient (0.28 cm2 s−1) of water vapor to generate supersaturated air to grow nanoparticles into microdroplets.27 Specifically, before the high temperature of the initiator is transferred to the center of the channel, the generated steam creates supersaturated air, at which point the nanoparticles grow into microdroplets. The initiator design ensured a sufficiently long channel to achieve the maximum centerline supersaturation state. The supersaturated air from the initiator is then cooled in the moderator, which maintains saturated air to retain particles in a microdroplet state. Additionally, the moderator collects surplus vapor, reducing the temperature and humidity of the outflow air.38 This method reduces measurement errors caused by the condensation of water vapor on the OPC and minimizes the amount of working fluid consumed within the system. The grown microdroplets are then passed through a miniaturized OPC that illuminates them with a laser. The scattered light is measured to count the number of airborne nanoparticles, enabling the measurement of nanoparticle concentrations in air.
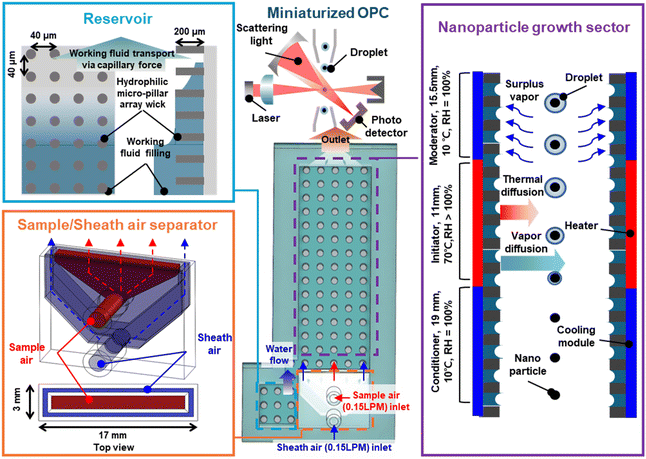 |
| Fig. 1 Working principle of the integrated microfluidic-based ultrafine water condensation particle counter (UWCPC), which consisted of three key components: a sample/sheath air separator, a nanoparticle growth sector, and a miniaturized optical particle counter (OPC). | |
UWCPC chip design
To effectively miniaturize the system, key elements for generating supersaturated steam (e.g., heater, super hydrophilic micro-pillar array wick, and water level sensor) were integrated on the PCBs, and structures with complex shapes required for airflow were printed with a 3D printer. Thus, we enabled the growth of nanoparticles into microdroplets on a microfluidic chip.
Simulation of the nanoparticle growth sector.
To confirm that the nanoparticles grew into microdroplets inside the nanoparticle growth sector, simulations were conducted to optimize the design. Computational fluid dynamics (CFD) software (Fluent 19.2, Ansys, USA) was used to calculate streamline plots, relative humidity profiles, and Kelvin activation diameter (DK). Using the integrated mesh generator included in the CFD program, we created a tetrahedral mesh with 1
388
708 nodes and 6
531
395 elements. A diffusion transport model was employed to simulate the vapor mass transfer. Streamline plots within the nanoparticle growth section are shown in Fig. 2(a). Sample air and sheath air were each injected at 0.15 LPM. The sheath air enveloped the sample air, inducing it to flow towards the centerline. The relative humidity contours show the 1.5 mm profile in the middle of a 3 mm thick channel (Fig. 2(b)). The relative humidity profile confirmed that the air passing through the conditioner was saturated. The initiator generated a supersaturated state in which nanoparticles could grow into microdroplets. At this time, the supersaturation ratio was higher at the centre than at the edge of the channel. When linked to streamline plots, the sample air surrounded by sheath air passes through a section with a high supersaturation area. The airflow through the initiator returned to a saturated state after cooling by the moderator.
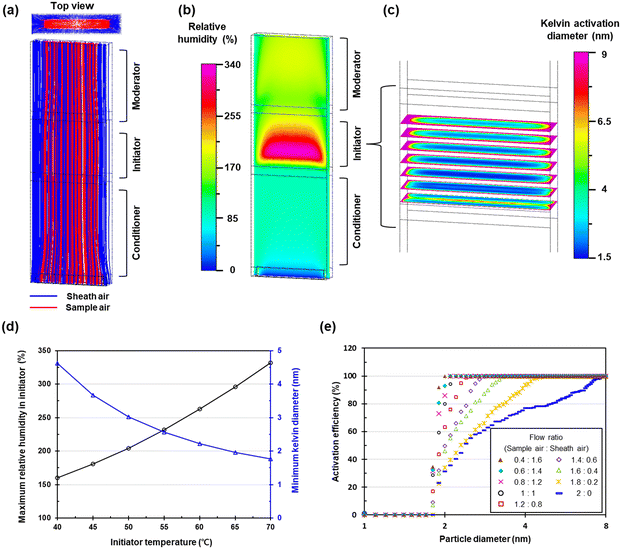 |
| Fig. 2 Simulation results of our system: (a) streamline plots of sample and sheath air inside the nanoparticle growth sector. (b) Relative humidity contour inside the nanoparticle growth sector. (c) Kelvin activation diameter contour of the initiator. (d) Maximum relative humidity and Kelvin activation diameter with the initiator temperature. (e) Particle activation efficiency according to the sample-to-sheath air ratio. | |
Fig. 2(c) shows the calculated profile of the Kelvin activation diameters within the initiator, which is defined as the minimum particle diameter that can grow in a supersaturated state and expressed as follows:
|  | (1) |
where
σ,
M,
Rg,
T,
ρ and
S represent the water surface tension (0.0727 N m
−1), molecular weight (18 g mol
−1), gas constant (8.31 J mol
−1 K
−1), temperature, density (1000 kg m
3) and saturation ratio, respectively.
39 Water vapor was immediately supplied upon initiator introduction, and a region located 3.1 mm downstream from the initiator was confirmed as the minimum Kelvin activation diameter range. The minimum Kelvin activation diameter for the sample flow was calculated as 1.77 nm; the average Kelvin activation diameters of the sample and sheath air were 1.88 and 5.27 nm, respectively. These simulation results confirmed the effectiveness of the sheath air and the feasibility of particle growth below 2 nm.
Fig. 2(d) shows the maximum relative humidity and minimum Kelvin activation diameter with respect to the initiator temperature. The x-axis is the temperature of the initiator, the y-axis is the maximum relative humidity point inside the channel, and the auxiliary y-axis is the minimum Kelvin activation diameter inside the channel. The lower the temperature of the initiator, the lower the relative humidity, and the larger the Kelvin activation diameter. In contrast, as the temperature of the initiator increases, more vapor was generated, increasing the relative humidity, and decreasing the Kelvin activation diameter. Therefore, to grow nanoparticles of smaller diameter into droplets, the supersaturation ratio must be increased by generating more vapor. Accordingly, to grow nanoparticles, a large amount of vapor must be generated by heating the initiator to a higher temperature. Fig. 2(e) shows the particle activation efficiency, the probability that nanoparticles in the sample air grow into microdroplets during supersaturation, according to the ratio of sample to sheath air. As the sheath air ratio decreased, the sample air passed through the low-supersaturation section; thus, the activation efficiency slope gradually decreased. When the ratio of sample to sheath air was 1
:
1, an activation efficiency of 90% was calculated at 2.1 nm; at a ratio of 2
:
0 (cutting off the sheath air supply), it was calculated at 6.6 nm, suggesting decreased CPC performance. Additionally, in situations where the sheath air exceeded the sample air, an activation efficiency of 90% was calculated for particle diameters between 1.9 and 2.1 nm. These values are similar to those obtained when the ratio of sample air to sheath air is 1
:
1. However, if the flow rate of sample air flow is reduced, particle loss due to diffusion increases when the sample air is introduced, which may lead to inaccurate measurement of the nanoparticle concentration.
Fabrication process.
Fig. 3(a) shows component optical and scanning electron microscope (SEM) images of the nanoparticle growth sector, which consisted of the top and bottom PCBs (76.5 mm × 41 mm in width and height, respectively) and 3D-printed structures. The water-level sensor and temperature sensor electrodes were patterned on the bottom PCB, whereas the heater and super hydrophilic micro-pillar array wick were integrated on both the top and bottom PCBs. The super hydrophilic micro-pillar array wick was fabricated through a simple photolithography process using a SUEX film with high thermal and chemical stability based on our previous research.33 First, a seed layer was produced by laminating a 100 μm-thick SUEX film on all PCBs except the soldering electrodes. Then, a 200 μm-thick SUEX film was laminated on the PCB board and photolithography was performed to fabricate the pattern of the micro-pillar array with diameters and pitches of 40 and 100 μm, respectively. However, because the fabricated micro-pillar array was hydrophobic, it was not suitable for application in CPCs using water as the working fluid. Accordingly, a treatment to graft the acrylamide monomer was performed to create a super hydrophilic micro-pillar array surface.40 PCBs with micro-pillar arrays were incubated in a mixture of 1 M nitric acid (HNO3) and 0.1 M cerium(IV) for 12 h to activate the epoxide rings. Then, the PCB was incubated again in a mixture of 75 mM nitric acid, 7.5 mM CAN, and 10 wt% acrylamide monomers.
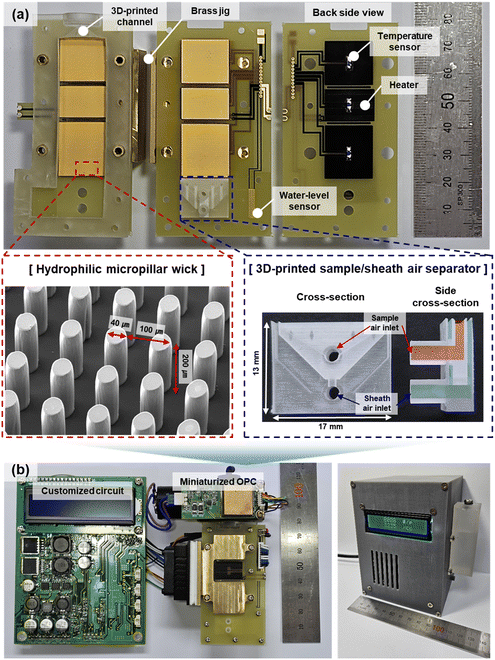 |
| Fig. 3 (a) Optical image and scanning electron microscope (SEM) images of the fabricated lab-on-PCB and 3D-printed structures. (b) Optical image of the assembled system. | |
The 3D-printed channel was printed with acrylic monomers using the UV stereolithography apparatus (SLA) method. The acrylic polymer used in the SLA method is a rubber material with excellent flexibility and elasticity owing to which sealing components such as separate O-rings are not required to assemble PCBs and 3D-printed channels. The 3D-printed sample/sheath air separator was printed with an acrylic polymer using the multi-jet modeling (MJM) method. The MJM printing technique has a minimum resolution of about 300 μm, which makes it suitable for fabricating complex microfluidic structures.
The two fabricated PCBs and two 3D-printed structures were assembled using brass jigs including Peltier element (MPADV-127-140
160-S, MULTICOMP PRO, UK) and heatsink-fan assembly (CEBF0140401605-00, MALICO, Taiwan), and a customized miniaturized OPC was installed (Fig. 3(b)). The Peltier element, heatsink-fan assembly, heater, and air pumps (G6/01-K-LC, Thomas, USA) installed through the customized circuit were controlled via a pulse width modulation (PWM) control using a temperature sensor (PTS0805M, Vishay, USA) and a flow sensor (AWM3100V, Honeywell, USA) and identified the number of particles by processing the signal of the miniaturized OPC.
Performance evaluation setup
A schematic diagram of the system performance evaluation setup is presented in Fig. 4, and it was set up based on the experimental method for UCPC performance evaluation.41 The nanoparticle generation section shows the setup for test particle generation. Test sodium chloride (NaCl) and ammonium sulfate ((NH4)2SO4) particles were generated through evaporation in a furnace.42 To minimize particle contamination, nitrogen was used as the carrier gas. Sodium chloride crystals were expected to produce nanoparticles of the same composition. However, in the case of ammonium sulfate, thermal decomposition may lead to variations in composition involving ammonium bisulfate, pyrosulfate, ammonia, and sulfur dioxide, resulting in non-uniform nanoparticles.43
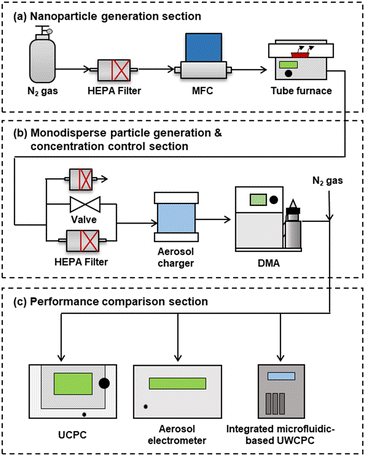 |
| Fig. 4 Experimental setup for the performance evaluation of our system. | |
The generated nanoparticles were introduced into the monodisperse particle generation and concentration control sections. The monodisperse particles were adjusted to the target particle concentration as they passed through a bridge with a valve and a HEPA filter connected in parallel, and the airflow, except for the flow required by the equipment, was discharged. The polydisperse nanoparticles were charged using an aerosol charger and classified into monodisperse particles (2 to 20 nm) using a nano-differential mobility analyzer (DMA; 3085, TSI, USA).
The monodisperse particles adjusted to the target concentration were utilized for the evaluation of our system, as described in the performance comparison section. In this section, quantitative experiments were conducted using a reference aerosol instrument, an ultrafine condensation particle counter (UCPC; 3776, TSI, USA), and an aerosol electrometer (3068B, TSI, USA).
Results and discussion
Particle counting efficiency according to initiator temperature
The 50% cut-off diameter of particle-counting efficiency (D50) was taken as a representative performance indicator of the CPC. D50 is the point in the CPC where only half of the actual number of particles is measured. As the size of particles decreases, they are less likely to grow into droplets, and particle-counting efficiency gradually decreases. Accordingly, D50 is defined as the minimum detectable particle size. The particle-counting efficiency of the CPC is defined as: | ηtot(Dp) = ηl(Dp)·ηa(Dp)·ηd(Dp) | (2) |
where ηl(Dp) is the particle loss inside the device, ηa(Dp) corresponds to the activation efficiency of nanoparticle transformation into droplets, and ηd(Dp) is the droplet counting efficiency in the optical counter.44 Among the above parameters, ηa(Dp) has a dominant influence, which is directly related to Kelvin activation diameters. Therefore, the parameters that determine Kelvin activation diameters are highly dependent on the introduced nanoparticle diameter and the saturation ratio (air supersaturation rate due to vapor generated within the nanoparticle growth sector) inside the system. Therefore, the counting efficiency should be characterized as a function of the particle size and operating temperature of the nanoparticle growth sector.
Fig. 5 shows the particle counting efficiency results according to the initiator temperature of our system and particle type. The temperature of the initiator was increased from 50 °C to 70 °C with 10 °C intervals. At a temperature of ≥70 °C, D50 measurement was not performed because of concerns regarding damage to the super hydrophilic micro-pillar array wick. NaCl and (NH4)2SO4 particles 2–14 nm in diameter were used for the test. Using our system, it was possible to measure 2.5 nm NaCl particles (Fig. 5(a)), which is similar to the minimum Kelvin diameter (1.88 nm) calculated from the simulation. At 70 °C, the D50 was measured at an approximate particle diameter of 3.4 nm. At 60 °C and 50 °C, the D50 was measured at an approximate particle diameter of 4.2 nm and 5.1 nm, respectively. As the temperature of the initiator increased, the saturation ratio inside the system increased and the measurable particle size decreased rapidly. In the case of (NH4)2SO4 particles, the D50 was 6.0, 5.2, and 4.4 nm when the initiator temperature was 50, 60, and 70 °C, respectively (Fig. 5(b)). The D50 of the (NH4)2SO4 particles was on average approximately 0.96 nm larger than that of the NaCl particles. This is because NaCl particles have higher hygroscopicity than do (NH4)2SO4 particles, and thus the probability of droplet growth may be higher even in the same relative humidity environment; it can be inferred that the D50 of NaCl particles was lower.45
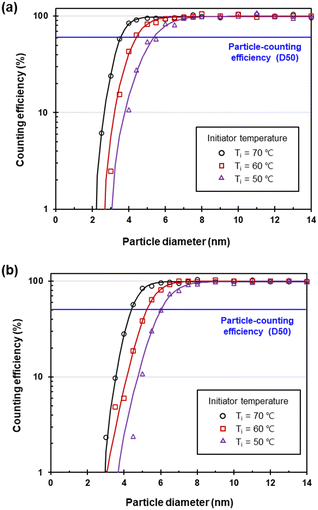 |
| Fig. 5 Particle counting efficiency of our system as a function of initiator temperature: (a) NaCl and (b) (NH4)2SO4 particles. | |
Particle-counting efficiency according to the sample-to-sheath air ratio
Fig. 6 illustrates the particle-counting efficiency with respect to the sample-to-sheath air ratio. The combined total of the sample and sheath air was set to 0.3 LPM. The evaluation was conducted by incrementally increasing the sample air by 0.03 LPM and correspondingly decreasing the sheath air by the same amount. NaCl was used as the test particle. As the amount of sheath air decreased, the slope of the particle-counting efficiency curve gradually decreased. These results suggest that the lower the sheath air, the higher the percentage of nanoparticles passing near the walls that have a low saturation ratio compared to the center of the channel, which has a high saturation ratio. When sheath air was increased, particle-counting efficiency was slightly higher than when the ratio of sample air to sheath air was 1
:
1. However, as this difference is minor, securing a large amount of sample air is more efficient in terms of measuring the nanoparticle concentration. These results highlight the significant impact of the sheath air on the distribution and behavior of nanoparticles within the channel.
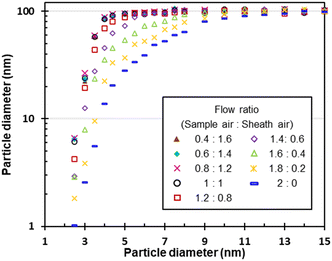 |
| Fig. 6 Particle counting efficiency of our system as a function of the sample-to-sheath air ratio. | |
Comparison between the lab-on-PCB and 3D-printing combination technology-based UWCPC and reference instruments
Fig. 7(a) and (b) show the particle number concentration comparisons between our system and a UCPC with time, while Fig. 7(c) shows a particle number concentration linearity comparison between the two systems. NaCl particles of 20 nm diameter were utilized, encompassing a number concentration spectrum spanning from 0 to 57
000 particles per cubic centimeter (N cm−3). Within the scope of a relatively low particle concentration (0–8000 N cm−3), the comparison between the herein proposed system and a UCPC revealed an observed discrepancy of approximately 11.41%. The reason for the high error in the low particle number concentration environment is the effect of the CPC's coefficient of uncertainty.46 The particle number concentration measured by our system exhibited a nearly one-to-one correlation with that of the reference UCPC when the concentration was <42
000 N cm−3 (Fig. 7(b)).
 |
| Fig. 7 (a) Low particle number concentrations over time in our system and a reference ultrafine condensation particle counter (UCPC); (b) high particle number concentrations over time in our system and a reference UCPC; (c) low particle number concentration linearity comparison between our system and a reference UCPC; and (d) high particle number concentration linearity comparison between our system and a reference UCPC. | |
However, as the concentration was elevated from 42
000 to 50
000 N cm−3, the relative difference between both systems grew from 5.69% to 8.84%. The increase in this difference is expected to have resulted from a coincidence error incorrectly measuring multiple droplets as a single one.
Conclusions
We developed a UWCPC based on a combination of lab-on-PCB and 3D-printing technologies for monitoring airborne nanoparticle generation and growth mechanisms. Our system consisted of two PCBs and two 3D-printed structures, and each part necessary for nanoparticle growth was integrated on a single chip. The lab-on-PCB and 3D-printing technologies used for fabrication are optimal methods for manufacturing low-cost, miniaturized microfluidic chips and are thus suitable tools for CPC sensorization.
Our system was evaluated through quantitative experiments and compared with reference aerosol instruments. The minimum detectable nanoparticle diameter (D50) of our system was 3.4 nm. In addition, simulations and experiments were conducted to confirm the effect of sheath air, a key element in UCPC performance. Finally, a one-to-one comparison with a reference UCPC in low and high number particle concentration ranges was conducted to confirm the high agreement between the two systems.
The lab-on-PCB and 3D-printing technology-based UWCPC is a low-cost, miniaturized UWCPC that can precisely measure nanoparticles. Accordingly, if our system is verified through various field testing, it could become a cornerstone tool in two-dimensional (2D) nanoparticle analysis over a wide area.
Glossary of abbreviations
2D | Two-dimensional |
3D | Three-dimensional |
CFD | Computational fluid dynamics |
CPC | Condensation particle counter |
D
50
| 50% cut-off diameter of particle-counting efficiency |
D
K
| Kelvin activation diameter |
DMA | Differential mobility analyzer |
HEPA | High-efficiency particulate air |
HNO3 | Nitric acid |
LPM | Liters per minute |
M
| Molecular weight |
MEMS | Microelectromechanical systems |
MJM | Multi-jet modeling |
NaCl | Sodium chloride |
(NH4)2SO4 | Ammonium sulfate |
OPC | Optical particle counter |
PCB | Printed circuit board |
PM | Particulate matter |
PWM | Pulse width modulation |
R
g
| Gas constant |
S
| Saturation ratio |
SEM | Scanning electron microscope |
SLA | Stereolithography apparatus |
T
| Temperature |
UCPC | Ultrafine condensation particle counter |
UWCPC | Ultrafine water condensation particle counter |
WCPC | Water condensation particle counter |
η
a(Dp) | Activation efficiency of nanoparticle transformation into droplet |
η
d(Dp) | Droplet counting efficiency in the optical counter |
η
l(Dp) | Particle loss inside the device |
η
tot(Dp) | Particle-counting efficiency of the CPC |
ρ
| Density of water |
σ
| Surface tension of water |
Conflicts of interest
There are no conflicts to declare.
Acknowledgements
This research was supported by the convergence R&D over Science and Technology Liberal Arts Program through the National Research Foundation of Korea funded by the Ministry of Science and ICT (Grant No. 2022M3C1B6081061). This work was supported by the National Research Foundation of Korea (NRF) grant funded by the Korea government (MSIT) (No. RS-2023-00222166).
Notes and references
- Z. Wu, M. Hu, P. Lin, S. Liu, B. Wehner and A. Wiedensohler, Particle number size distribution in the urban atmosphere of Beijing, China, Atmos. Environ., 2008, 42, 7967–7980 CrossRef CAS.
-
A. A. Shvedova, T. Sager, A. R. Murray, E. Kisin, D. W. Porter, S. S. Leonard, D. Schwegler-Berry, V. A. Robinson and V. Castranova, Critical issues in the evaluation of possible adverse pulmonary effects resulting from airborne nanoparticles, Nanotoxicology: Characterization, Dosing, and Health Effects, ed. N. A. Monterio-Riviere and C. L. Tran, CRC Press, New York, 2007, pp. 225–236 Search PubMed.
- M. E. Quadros and L. C. Marr, Environmental and human health risks of aerosolized silver nanoparticles, J. Air Waste Manage. Assoc., 2010, 60, 770–781 CrossRef CAS PubMed.
- G. Oberdörster and M. J. Utell, Ultrafine particles in the urban air: to the respiratory tract--and beyond?, Environ. Health Perspect., 2002, 110, A440–A441 CrossRef.
- K. Donaldson, X. Li and W. MacNee, Ultrafine (nanometre) particle mediated lung injury, J. Aerosol Sci., 1998, 29, 553–560 CrossRef CAS.
- H. Bahadar, F. Maqbool, K. Niaz and M. Abdollahi, Toxicity of nanoparticles and an overview of current experimental models, Iran. Biomed. J., 2016, 20, 1 Search PubMed.
- J. P. Vareda, C. A. García-González, A. J. Valente, R. Simón-Vázquez, M. Stipetic and L. Durães, Insights on toxicity, safe handling and disposal of silica aerogels and amorphous nanoparticles, Environ. Sci.: Nano, 2021, 8, 1177–1195 RSC.
- H. Sheikh, P.-Y. Tung, E. Ringe and R. Harrison, Magnetic and microscopic investigation of airborne iron oxide nanoparticles in the London Underground, Sci. Rep., 2022, 12, 20298 CrossRef CAS PubMed.
- V. Dhananjayan, B. Ravichandran, S. Sen and K. Panjakumar, Source, effect, and risk assessment of nanoparticles with special reference to occupational exposure, Nanoarchitecton. Biomed., 2019, 643–676 CAS.
- A. N. Al-Dabbous, P. Kumar and A. R. Khan, Prediction of airborne nanoparticles at roadside location using a feed–forward artificial neural network, Atmos. Pollut. Res., 2017, 8, 446–454 CrossRef.
- T. Rönkkö and H. Timonen, Overview of sources and characteristics of nanoparticles in urban traffic-influenced areas, J. Alzheimer's Dis., 2019, 72, 15–28 Search PubMed.
- Y. Wu, X. Wang, J. Tao, R. Huang, P. Tian, J. Cao, L. Zhang, K.-F. Ho, Z. Han and R. Zhang, Size distribution and source of black carbon aerosol in urban Beijing during winter haze episodes, Atmos. Chem. Phys., 2017, 17, 7965–7975 CrossRef CAS.
- R. Zhang, A. Khalizov, L. Wang, M. Hu and W. Xu, Nucleation and growth of nanoparticles in the atmosphere, Chem. Rev., 2012, 112, 1957–2011 CrossRef CAS PubMed.
- T. Nieminen, V.-M. Kerminen, T. Petäjä, P. P. Aalto, M. Arshinov, E. Asmi, U. Baltensperger, D. C. Beddows, J. P. Beukes and D. Collins, Global analysis of continental boundary layer new particle formation based on long-term measurements, Atmos. Chem. Phys., 2018, 18, 14737–14756 CrossRef CAS.
- S. P. Cunningham, C. J. Spanos and K. Voros, Semiconductor yield improvement: results and best practices, IEEE Trans. Semicond. Manuf., 1995, 8, 103–109 CrossRef.
-
S. A. Hoenig, in Particles on Surfaces 1: Detection, Adhesion, and Removal, Springer, 1988, pp. 3–16 Search PubMed.
-
R. P. Donovan, Particle control for semiconductor manufacturing, Routledge, 2018 Search PubMed.
- A. J. Steinman, Preventing electrostatic problems in semiconductor manufacturing, Compliance Engineering, 2004, 21, 89–93 Search PubMed.
- C. Osburn, H. Berger, R. Donovan and G. Jones, The effects of contamination on semiconductor manufacturing yield, J. Environ. Sci., 1988, 31, 45–57 CAS.
-
K. Kanzawa and J. Kitano, A semiconductor device manufacturer's efforts for controlling and evaluating atmospheric pollution, Proceedings of SEMI Advanced Semiconductor Manufacturing Conference and Workshop, IEEE, 1995 Search PubMed.
- P. Kumar, L. Morawska, C. Martani, G. Biskos, M. Neophytou, S. Di Sabatino, M. Bell, L. Norford and R. Britter, The rise of low-cost sensing for managing air pollution in cities, Environ. Int., 2015, 75, 199–205 CrossRef PubMed.
- M. Sipilä, K. Lehtipalo, M. Kulmala, T. Petäjä, H. Junninen, P. P. Aalto, H. E. Manninen, E.-M. Kyrö, E. Asmi and I. Riipinen, Applicability of condensation particle counters to measure atmospheric clusters, Atmos. Chem. Phys., 2008, 8, 4049–4060 CrossRef.
- R. B. Jørgensen, Comparison of four nanoparticle monitoring instruments relevant for occupational hygiene applications, J. Occup. Med. Toxicol., 2019, 14, 1–14 CrossRef PubMed.
- A. M. Dixon Heathman and D. Ensor, Monitoring of Nanoscale Particles in Cleanrooms: ISO 14644-12, J. IEST, 2019, 62, 50–59 CrossRef.
-
P. Kulkarni, P. A. Baron and K. Willeke, Aerosol measurement: principles, techniques, and applications, John Wiley & Sons, 2011 Search PubMed.
- P. H. McMurry, The history of condensation nucleus counters, Aerosol Sci. Technol., 2000, 33, 297–322 CrossRef CAS.
- G. Mordas, H. Manninen, T. Petäjä, P. Aalto, K. Hämeri and M. Kulmala, On operation of the ultra-fine water-based CPC TSI 3786 and comparison with other TSI models (TSI 3776, TSI 3772, TSI 3025, TSI 3010, TSI 3007), Aerosol Sci. Technol., 2008, 42, 152–158 CrossRef CAS.
- C. Kuang, M. Chen, P. H. McMurry and J. Wang, Modification of laminar flow ultrafine condensation particle counters for the enhanced detection of 1 nm condensation nuclei, Aerosol Sci. Technol., 2012, 46, 309–315 CrossRef CAS.
- N. T. Thanh, N. Maclean and S. Mahiddine, Mechanisms of nucleation and growth of nanoparticles in solution, Chem. Rev., 2014, 114, 7610–7630 CrossRef CAS PubMed.
- K. Iida, M. R. Stolzenburg, P. H. McMurry, J. N. Smith, F. R. Quant, D. R. Oberreit, P. B. Keady, A. Eiguren-Fernandez, G. S. Lewis and N. M. Kreisberg, An ultrafine, water-based condensation particle counter and its evaluation under field conditions, Aerosol Sci. Technol., 2008, 42, 862–871 CrossRef CAS.
- S.-J. Yoo, H.-B. Kwon, U.-S. Hong, D.-H. Kang, S.-M. Lee, J. Han, J. Hwang and Y.-J. Kim, Microelectromechanical-system-based condensation particle counter for real-time monitoring of airborne ultrafine particles, Atmos. Meas. Tech., 2019, 12, 5335–5345 CrossRef CAS.
- H.-B. Kwon, S.-J. Yoo and Y.-J. Kim, Microfluidic condensation nanoparticle counter using water as the condensing liquid for assessing individual exposure to airborne nanoparticles, Lab Chip, 2020, 20, 1092–1102 RSC.
- S.-J. Yoo, J.-W. Jeon and Y.-J. Kim, Lab-on-printed circuit board based water harvesting condensation particle counter for ubiquitous monitoring of airborne ultrafine particles, J. Aerosol Sci., 2022, 160, 105911 CrossRef CAS.
- H. T. Thanh, T. V. Quoc, P. N. Van, L. D. Quang, A. N. Ngoc, C. T. Nhu, N. N. Hoang, N. T. Le, T. T. Nguyen and T. T. Bui, A combination of 3D printing and PCB technologies in microfluidic sensing device fabrication, Microsyst. Technol., 2022, 28, 1607–1619 CrossRef.
- S. V. Hering, G. S. Lewis, S. R. Spielman and A. Eiguren-Fernandez, A MAGIC concept for self-sustained, water-based, ultrafine particle counting, Aerosol Sci. Technol., 2019, 53, 63–72 CrossRef CAS.
- W. Liu, S. L. Kaufman, B. L. Osmondson, G. J. Sem, F. R. Quant and D. R. Oberreit, Water-based condensation particle counters for environmental monitoring of ultrafine particles, J. Air Waste Manage. Assoc., 2006, 56, 444–455 CrossRef CAS PubMed.
- S. V. Hering, M. R. Stolzenburg, F. R. Quant, D. R. Oberreit and P. B. Keady, A laminar-flow, water-based condensation particle counter (WCPC), Aerosol Sci. Technol., 2005, 39, 659–672 CrossRef CAS.
- S. V. Hering, S. R. Spielman and G. S. Lewis, Moderated, water-based, condensational particle growth in a laminar flow, Aerosol Sci. Technol., 2014, 48, 401–408 CrossRef CAS PubMed.
-
W. C. Hinds and Y. Zhu, Aerosol technology: properties, behavior, and measurement of airborne particles, John Wiley & Sons, 2022 Search PubMed.
- Y. Wang, J.-H. Pai, H.-H. Lai, C. E. Sims, M. Bachman, G. Li and N. L. Allbritton, Surface graft polymerization of SU-8 for bio-MEMS applications, J. Micromech. Microeng., 2007, 17, 1371 CrossRef CAS.
- K. Iida, M. R. Stolzenburg and P. H. McMurry, Effect of working fluid on sub-2 nm particle detection with a laminar flow ultrafine condensation particle counter, Aerosol Sci. Technol., 2009, 43, 81–96 CrossRef CAS.
- H. Scheibel and J. Porstendo, Generation of monodisperse Ag-and NaCl-aerosols with particle diameters between 2 and 300 nm, J. Aerosol Sci., 1983, 14, 113–126 CrossRef CAS.
- R. Kiyoura and K. Urano, Mechanism, kinetics, and equilibrium of thermal decomposition of ammonium sulfate, Ind. Eng. Chem. Process Des. Dev., 1970, 9, 489–494 CrossRef CAS.
- M. R. Stolzenburg and P. H. McMurry, An ultrafine aerosol condensation nucleus counter, Aerosol Sci. Technol., 1991, 14, 48–65 CrossRef CAS.
- S. S. Vlasenko, H. Su, U. Pöschl, M. O. Andreae and E. F. Mikhailov, Tandem configuration of differential mobility and centrifugal particle mass analysers for investigating aerosol hygroscopic properties, Atmos. Meas. Tech., 2017, 10, 1269–1280 CrossRef.
- J. Kangasluoma and J. Kontkanen, On the sources of uncertainty in the sub-3 nm particle concentration measurement, J. Aerosol Sci., 2017, 112, 34–51 CrossRef CAS.
|
This journal is © The Royal Society of Chemistry 2024 |