DOI:
10.1039/D3EN00630A
(Critical Review)
Environ. Sci.: Nano, 2024,
11, 14-35
A critical review on the toxicity regulation and ecological risks of zinc oxide nanoparticles to plants†
Received
9th September 2023
, Accepted 6th November 2023
First published on 7th November 2023
Abstract
The advancement and application of nanotechnology inevitably cause the release of nanoparticles (NPs) into the environment, in particular, zinc oxide (ZnO) NPs may seriously threaten the ecological safety of plants. This review focuses on the translocation and physiological responses of ZnO NPs in plants to systematically summarize the toxicological effects and molecular mechanisms of NPs. The results indicated that ZnO NPs migrated to tissues via uptake and translocation, gradually accumulated in intracellular and intercellular spaces, and led to physiological inhibition, nutrient imbalance, and photosynthesis perturbation in plants. This abiotic stress triggered the overproduction of reactive oxygen species (ROS) by perturbing cellular redox homeostasis, while the activation of antioxidant genes and alteration of KEGG pathways enhanced the ability of plants to resist phytotoxicity. Furthermore, ZnO NPs significantly altered metabolites associated with oxidative stress, antioxidant defense, membrane disorder and energy expenditure, affected carbon/nitrogen metabolism via the TCA cycle and glycolysis pathway, and augmented cytotoxicity and genotoxicity by inducing DNA damage and inhibiting mitosis. More notably, the composite exposure of ZnO NPs with other substances is bifacial and may create potential mitigation or synergistic effects on plants in ecosystems, thus posing uncertain ecological risks. This review systematically provides clarification on the environmental fate of ZnO NPs in plants at the physiological and molecular levels, theoretical references on the toxicity mechanisms and potential risks of NPs, and directions and insights for future research to achieve strategies that minimize risks and maximize benefits.
Environmental significance
The continuous accumulation of zinc oxide nanoparticles (ZnO NPs) in the environment poses a potential threat to ecological security due to their unique physical and chemical properties. As important receptors for NPs in ecosystems, plants can interact with NPs at the cellular and subcellular levels, which can alter their physiological structure, metabolic response processes, and molecular regulation. This review clarifies the fate of interactions between ZnO NPs and plants, reveals the translocation mechanism and toxicity regulation of NPs, and emphasizes the uncertain ecological risks induced by composite systems of ZnO NPs with other substances. These perspectives provide a more comprehensive understanding of the environmental behavior and toxicological effects of NPs and broaden the research directions in this field.
|
1 Introduction
With the application of nanomaterials and advanced breakthroughs in nanotechnology,1 nanoparticles (NPs) are extensively applied in sensors,2 care products, biomedicines,3 food packaging materials,4 and nano-agricultural chemicals (eg., nano-pesticides5 and nano-fertilizers6) owing to their unique physical, chemical, and biological properties, such as superior charge transfer efficiency,7 high payload,8 and excellent antimicrobial characteristics.9 Despite the social benefits brought about by the application of nanotechnology, it is necessary to consider constructing a system for the accidental release risk probabilities10 and environmental exposure assessment11 of NPs. The release of NPs into the environment is primarily categorized into natural phenomena and anthropogenic emissions. It has been found that natural phenomena include volcanic eruptions, evaporation of water bodies, forest fires and soil erosion, while NPs produced by these natural sources do not appear to be harmful to organisms.12 As for the sources of anthropogenic emissions, NPs can be intentionally or unintentionally released into the ecosystem through personal consumption goods, industrial production, nano-agricultural product application,13 landfill14 and sewage treatment plant disposal,15 thus aggravating the ecological risk of particles in the soil and water environments. The NPs entering natural systems are extremely likely to be ingested by plants16 and animals17 from where they can be transmitted to higher trophic levels via the aquatic18 or terrestrial19 food chain. Mathematical models20 and biodynamic methods21 can predict the toxicity of NPs in trophic transfer, and the risk assessment also confirmed that the consumption of NP-contaminated plants may pose a potential health threat22 due to typical accumulation effects. The above-mentioned fate of the source and release of NPs in the environment is shown in Fig. 1. Exploring the interactions of NPs with organisms and their biotransformation in natural ecosystems can contribute to pioneering the emerging nanotoxicology research, which has attracted considerable attention from the chemical, environmental, agricultural, food, and medical communities.
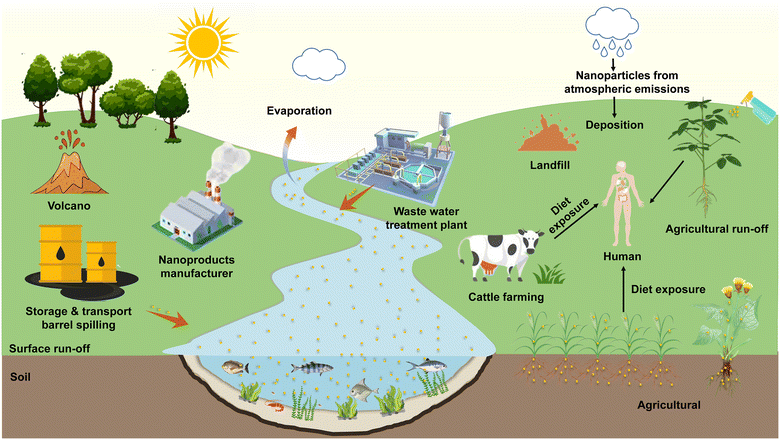 |
| Fig. 1 Environmental fate of sources and release of NPs in ecosystems. Adapted with permission from ref. 12. Copyright (2021) Elsevier. | |
As a representative metal-based nanomaterial with excellent sensing properties23 and antimicrobial characteristics,24 zinc oxide (ZnO) NPs have been massively produced for application in electronic sensors,25 optoelectronic devices,26 medical supplies,27 cosmetics,28 and food packaging materials.29 Due to the extensive application and continuous increase of consumer products with ZnO NPs, the cumulative concentration of their emission into the environment will inevitably be widely detected. For instance, Frechette-Viens et al. identified 4.4 × 105 particles per mL and 1.0 × 105 particles per mL of ZnO NPs in natural rivers and rainwater in Canada, respectively.30 Choi et al. also suggested that the concentration of ZnO NPs flowing into a wastewater treatment plant in Maryland, United States, ranged from 20.0 ± 12.0 to 212.0 ± 53.0 μg L−1.31 The toxicological effects and ecological risks caused by ZnO NPs to animals or plants have subsequently aroused intensive social concerns. The threat of ZnO NPs depends on their physicochemical properties (e.g., size, shape, surface charge, and ion release), culture conditions (e.g., exposure concentration, soil culture, and hydroponics), environmental factors (e.g., temperature, humidity, and light) and plant species. In certain treatments, ZnO NPs can produce negative effects32 or positive benefits33 and are mainly regulated by concentration dependence. More importantly, natural ecosystems show the presence of a large quantity of pollutants, biomolecules, and other components. Given the growing environmental exposure to NPs, they may have synergistic34 or antagonistic toxic effects35 in organisms36 when they are combined with different environmental mediators. Nevertheless, in addition to the biotoxicity induced by individual NPs, the behavior and toxicity of complexes of binary or multiple NPs are also largely subject to significant challenges.
Plants, as crucial species components in aquatic and terrestrial ecosystems, are both ecological receptors for NPs and carriers of transformation and bioconcentration in the food chain. More importantly, particles are absorbed primarily in the early stages of plant growth,37 thereby posing a threat to seedling development and gaining potential for translocation to edible parts.38 Ambient NPs can enter into plant cells and be transported to other tissues via root uptake and absorption through leaves or vesicles,39 where they not only interact at the cellular and subcellular levels but also alter plant physiology and morphology.40 It was confirmed that Zn precipitated outside the plasma membrane of root cells,41 induced strong oxidative stress and plasma membrane damage, up-regulated hydrogen peroxide (H2O2) levels, proline content and electrolyte leakage in leaves, and enhanced catalase (CAT), superoxide dismutase (SOD) and guaiacol peroxidase (GPX) activities.42 Interestingly, ZnO NPs acted as modifiers to alleviate the metabolic disorders and metal toxicity induced by Cd and improved plant physiology under heavy metal stress.43 In contrast, the adsorption of ZnO NPs on natural crystals in the aquatic environment could produce combined toxicity, exacerbating nutrient imbalance, biomolecular degradation, and protein folding in phytoplankton.44 These uncertain ecological risks raise a major challenge to the protection of plant health. Considering that ZnO NPs and composite systems of NPs can alter the normal physiological and biochemical states of plants, which inevitably threatens ecological security and environmental health. Consequently, further elucidation of the toxicity mechanisms and potential impacts of ZnO NPs in plants is extremely urgent.
2 Scope and methods of the review
The rapid evolution of nanotechnology has enabled the increasing development of nano-products, which are estimated to create an economic impact of several hundred billion dollars annually. The United States, China, Germany, Switzerland, and the United Kingdom are the top five countries manufacturing nano-products, with 3239, 816, 744, 465, and 404 nano-products respectively (Fig. 2a) for use in industries such as electronics, medicine, cosmetics, construction, textiles, automotive industry, environment, renewable energy and food (Fig. 2b). In particular, the global nano ZnO market was valued at US$ 958.5 Million in 2022 and is projected to reach US$ 2166.8 Million by 2028, growing at a compound annual growth rate of 14.19% during 2023-2028 (Nano Zinc Oxide Market: Global Industry Trends, Share, Size, Growth, Opportunity and Forecast 2023–2028).
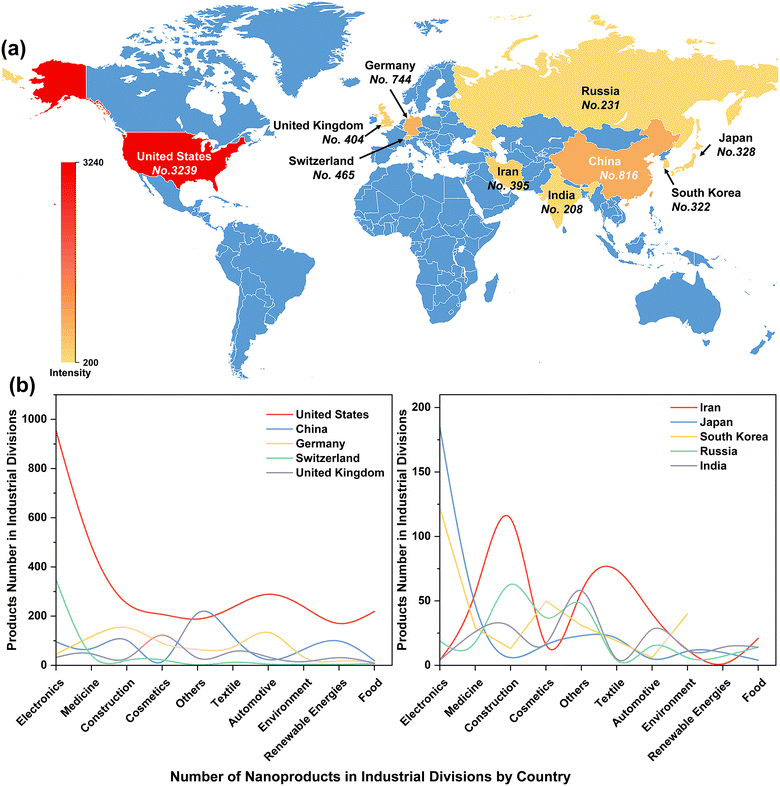 |
| Fig. 2 Number of nanotechnology products in major countries of the world from the nanotechnology product database (a), and statistics on the number of nanotechnology products in specific market classifications (b). | |
Scientific articles published from April 2013 to May 2023 were primarily searched using the ISI Web of Science standard engine. The search terms were “ZnO nano*”, “plant”, and “(toxic* or potential risk)”. The abstracts for retrieved articles were individually reviewed to screen for relevant literature. Only papers that addressed toxicity studies of ZnO NPs in plants were selected for analysis, while articles that failed to specify ZnO NPs and phytotoxicity were excluded. Ultimately, a total of 336 scientific papers were retrieved (Table S1†). The number of literature studies published annually was statistically evaluated using Origin 2022, and keywords that appeared more than 3 times repeatedly were visualized for network parsing using the VOS viewer.
The general upward trend in the number of publications on phytotoxicity studies of ZnO NPs over the last decade indicates that the environmental toxicology of NPs has gradually attracted widespread attention (Fig. 3a and b). Visual network analysis also revealed that researchers primarily analyzed the toxic effects of ZnO NPs on plants from a physiological perspective and focused on oxidative stress, antioxidant enzymes, lipid peroxidation, bioaccumulation, and bioavailability to elucidate the toxicity mechanisms of NPs (Fig. 3c). Apart from pristine ZnO NPs, the synergistic impacts of composite ZnO NPs on plants have also emerged as a research concern, especially the major challenge of unknown ecological risks of composite systems with heavy metals.
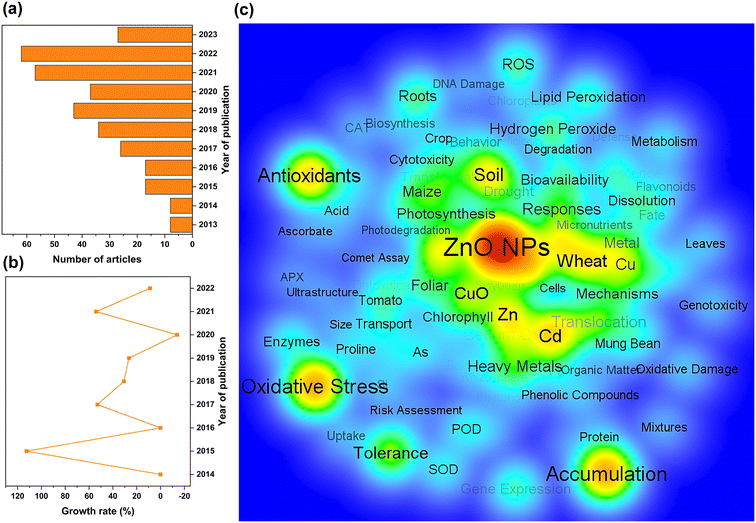 |
| Fig. 3 Number of literature studies on toxicity and risk of ZnO NPs to plants (a), progressive growth rate of targeted documents (b), and analysis of network visualization for keywords (c) from April 2013 to May 2023. | |
3 Translocation and toxicity mechanisms of ZnO NPs
3.1 Uptake and transport of NPs in plants
Studies have shown that once NPs enter specific organs such as leaves, stems and roots, they can be transported through the plant system to other parts.45 The uptake, translocation, and bioaccumulation of NPs by plants are related to the exposure pathways,46 where foliar spraying and root application are the two main treatments used in current studies (Fig. 4). Such applications commonly have a tendency to promote at low concentrations and inhibit at high concentrations in plants.47 Upon exposure, ZnO NPs can be absorbed by plants and translocated to tissues via different pathways. On the one hand, foliar-applied ZnO NPs mainly pass through the leaf epidermis via the stomatal pathway and accumulate in large amounts in the apoplastic space.48 Upon entering leaf tissues, ZnO NPs may be solubilized into Zn2+ in the intercellular space, while undissolved NPs can diffuse within the apoplast or partially move into the mesophyll cell49 and then be transported to other tissues.50 Similarly, the element Zn was capable of accumulating in mature Triticum aestivum L. leaves and migrating to the grain, with higher levels of Zn in the crease zone, aleurone layer, and embryo.51 The Zn homeostasis in cells is regulated by a family of Zn transporter protein members. For example, Sun et al. found that the elevation of Zn content in Solanum lycopersicum leaves after foliar spraying with ZnO NPs was regulated in negative feedback with Zn uptake in roots, which was confirmed by the lower expression levels of two Zn transporter protein genes of the ZIP family in roots, ZIP3 (Solyc02g081600.3) and ZIP5-like (Solyc07g043230.3).52 It was also found that the transcript level of the ZIP4 gene was considerably down-regulated to modulate high Zn bioavailability in Zea mays L. roots treated with ZnO NPs.53
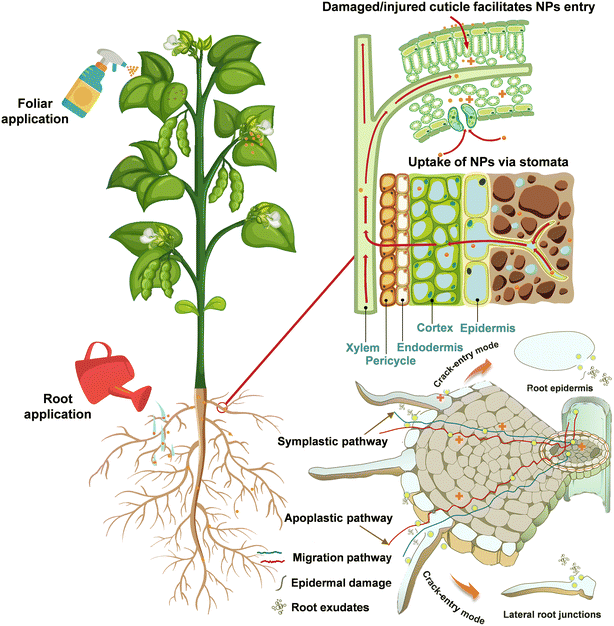 |
| Fig. 4 Root exposure and foliar application of ZnO NPs and transportation pathways of NPs in plant tissues. | |
On the other hand, for root application, aggregates of ZnO NPs could be observed at the root surface and at sites of lateral root junctions,54 speculating that crack generation may facilitate the entry of NPs. The pores of the cell wall and the vascular tissue at the root tip are potential pathways for NPs to subsequently enter the roots.55 The reactive oxygen species (ROS) generation triggered by abiotic stresses cause the loosening of the cell wall,56 which may allow more ZnO NPs to access cells. The NPs also increase the permeability of the cell wall and create new pores to further facilitate the uptake of NPs by root cells.57 Lv et al. reported that ZnO NPs internalized into epidermal cells were encapsulated by new cells with active cell division and elongation in the root tip and slowly transferred to other root zones.54 It has also been shown that ZnO NPs rapidly moved into the root crown of Zea mays L. and subsequently extended toward the primary vascular system of the root and the central column transport system of the stem.58 The presence of ZnO NPs was also observed in the intercellular space and cytoplasm of Oryza sativa L. root cells,59 whereas the localized ZnO NPs may cross the root epidermis and cortex via the apoplastic pathway and reach the xylem conduits across the endodermis via the symplastic pathway.60 The cellular internalization and transportation of ZnO NPs also depend on the activation of endocytosis to some extent.59 In particular, FM4-64 uptake and alterations in actin microfilaments were evidently enhanced in root cells treated with ZnO NPs (Fig. 5a),61 with the subsequent potential to control cellular communication and endocytosis. This membrane transport serves as a signal responding to environmental stimuli and can be involved in cellular regulation and receive signaling from targeted receptors.62
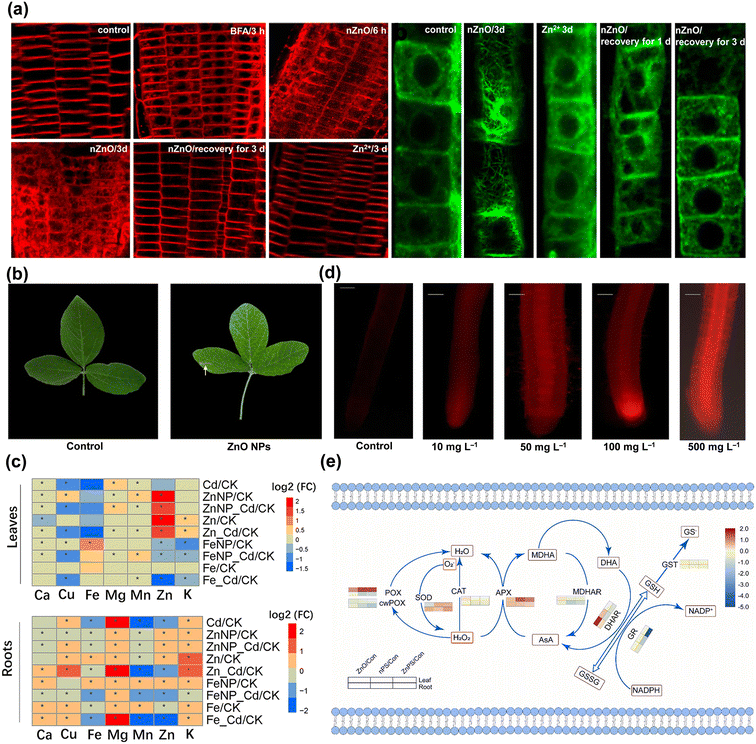 |
| Fig. 5 Root cell endocytosis and root epidermal cell microfilament rearrangement induced by ZnO NPs and Zn2+ (a). Adapted with permission from ref. 61. Copyright (2019) American Chemical Society. Necrotic lesions on Glycine max L. leaves at harvest (b). Adapted with permission from ref. 76. Copyright (2016) Elsevier. Heat map analysis of Fe3O4 and ZnO NPs on nutrient accumulation (c). Adapted with permission from ref. 82. Copyright (2022) Springer Nature. Micrographs of roots exposed to different ZnO NP treatments using propidium iodide (d). Adapted with permission from ref. 46. Copyright (2021) Springer Nature. Heat map of critical antioxidant enzyme activities in Hordeum vulgare L. leaves exposed to ZnO NPs and nanoplastics (e). Adapted with permission from ref. 34. Copyright (2022) Elsevier. | |
The ion channels, carrier proteins, and water channel proteins in plant cells provide the possibility for the migration of NPs.63 Electron micrographs revealed that ZnO NPs invaded and attached to the surfaces of cellular and nuclear membranes as well as cell junctions in Allium cepa L., which confirmed the intracellular transport of ZnO NPs via plasmodesmata channels.64 The transportation of ZnO NPs accumulated in the vacuoles of root cells via vesicles has also been observed,58 further suggesting that ZnO NPs can be internalized into the cytoplasm by endocytosis at the plasma membrane. Transporter carrier proteins or ion channels embedded in the cell membrane will contribute to the entry of NPs across the plasma membrane.57 Specifically, ZnO NPs induced transcription of metal carrier translocators (PvNRAMP3, PvNRAMP4, PvMTP1, and PvIRT3)65 and higher levels of the water channel protein genes TIP3-2 like (Solyc03g019820.3), TIP2-1 like (Solyc12g044330.2), PIP-type pTOM75 (Solyc01g103275.1), and TIP1-1 (Solyc10g083880.2).52 Notably, surface properties may affect the mobility of NPs in the apoplast and symplast and influence the loading and translocation of phloem.66 The small size property augmented the adsorption of ZnO NPs onto the leaf surface and cell walls,49 and also facilitated their entry into roots, leading to the deposition of massive amounts of ZnO NPs.58 The surface charge property also affected the interactions of NPs with biosystems owing to the electrostatic force. In particular, positively charged ZnO NPs were more homogeneously translocated into plant tissues than the negatively charged ones.49 Moreover, metal-based NPs that enter into plant cells can release metal ions and may alter their biochemical activities by reacting with different functional groups of proteins.63 The internalized NPs via endocytosis not only interfere with the expression and localization of plasma membrane proteins, but may also alter the actin microfilament structure in the root transition zone,61 thereby influencing the biological processes and dynamic fate of plant development.
3.2 Physiological inhibition of tissues and cells
The germination and early seedling stages are the most sensitive and critical phases in the plant life cycle to combat stress,67 and their physiological feedbacks are closely related to species.68 It was found that ZnO NPs significantly decreased the germination of Zea mays L. with 53% inhibition69 and also caused a severe reduction in the growth of Panicum maximum.70 Exposure to ZnO NPs curtailed the elongation of primary roots in Oryza sativa L. and led to the partial loss of root gravitropism.46 The internalized ZnO NPs also disrupted cell wall organization and structure in Arabidopsis thaliana via adhesion, physical interactions and genetic coding, thus inducing microfilament rearrangements in epidermal cells of the elongation zone (Fig. 5a) and inhibiting primary root growth.61 Gene ontology (GO) analysis revealed that ZnO NPs stimulated a high degree of gene regulation specifically related to “nitrogen compound metabolism” in Zea mays L. roots and confirmed a significant relationship between enhanced nitrogen absorbing capacity and root shortening.71 The impaired root epidermis and root cap, highly vacuolated and collapsed cortical cells and shrunken vascular columns were observed in Lolium perenne under ZnO NP stress, probably as a direct cause of the inhibition of plant growth.72 The interaction of ZnO NPs with the Allium cepa L. root surface also induced significant morphological changes such as cracks, breaks and spikes, and the mitochondria and nuclei were injured with the cellular internalization and biomolecular intervention of NPs.64 Similarly, after ZnO NP treatment, a bunch of dense particle deposits were found in the cellular matrix of root cells that exhibited obvious disintegration and structural deformation,73 and severely disrupted the physiological structure and state of root tissues.74
Exposure to ZnO NPs equally inhibits the normal growth of stems, leaves and cells, not only by reducing photosynthetic pigments and biomass in seedlings59 but also by perturbing the biochemical processes of growth factors in cells. Different concentrations of ZnO NPs reduced the fresh weight of Oryza sativa L. shoot tissues by 16.9–48.5%,59 and Fagopyrum esculentum biomass significantly by 7.7–26.4%, and incrementally raised the bioaccumulation of Zn with the increase in concentrations.75 It was found that Glycine max L. leaves grown in soil amended with ZnO NPs tended to be yellowed and damaged (Fig. 5b),76 which may be due to the fact that the large surface area of NPs predisposed the plant to uptake of different molecules and inorganic ions from nutrient media or soil, leading to the indirect toxicity symptom of wilting and chlorosis.63 The miR171b-mediated transcriptional up-regulation of SCARECROW 6 could be a determining factor in hindering chlorophyll biosynthesis and contributing to leaf yellowing.53 The high accumulation of ZnO NPs altered the stomatal conductance and the genes of chlorophyll synthesis, along with lowering transpiration rates and shrinking the growth phenotype of leaves.77 Besides, ZnO NPs can be solubilized in environmental media, and phytotoxicity may be related to the release of Zn2+ from NPs.78 The NPs releasing unstable Zn2+ were rapidly adsorbed onto the surface of algal cells and led to the total down-regulation of photosystems, oxidative phosphorylation, and transcriptional/translational mechanisms, which significantly reduced intracellular adenosine triphosphate (ATP) production and inhibited cell growth.79 Released Zn2+ was anchored inside the alga by binding to various metal-binding proteins in the chloroplasts and nucleus, potentially affecting the metabolism and physiology of Eremosphaera viridis by interfering with the flow between green algal cells and the environment.44
There is extensive literature suggesting that the physical and chemical properties of NPs play a crucial role in regulating phytotoxicity. For example, micron-sized ZnO as well as ZnO NPs with diameters smaller than 100 nm or 50 nm inhibited Phragmites australis growth.41 Especially, NPs < 50 nm emitted more Zn2+ and exhibited higher toxicity.41 In some cases, low doses of ZnO NPs promoted seed germination and plant growth, but high doses of NPs diminished this physiology.47 The toxicity of ZnO NPs was also influenced by plant species, and a higher sensitivity to NPs could be found in Solanum lycopersicum than in Triticum aestivum L. under the same treatments.47 Furthermore, the hydrodynamic diameter and surface charge of ZnO NPs can be impacted by the interaction with the surrounding environmental medium and lead to different biological responses in plant cells.36 The subsequent part of oxidative stress and antioxidant defense appears to explain this regulatory difference. The investigation of molecular regulation in physiological inhibition as the basis of phenotypic studies determines the lifeblood of plant growth and development, and more knowledge reserves will be required in establishing molecular-phenotypic theories.
3.3 Imbalance of mineral elements and nutrients
Nutrients play critical roles in plant growth and developmental stages via interacting sensing, signaling and metabolic processes.80 It was revealed that Ca2+ in Salicornia persica cells under ZnO NP stress increased in a concentration-dependent manner and triggered cellular apoptosis, which might be related to the overproduction of ROS and the dysregulation of Zn homeostasis.81 ZnO NPs also affected the synthesis of mineral ions and the balance of nutrients (Fig. 5c) such as Cu, Mg, K, Fe, Mn, Ca, and Zn in seedlings,82 altered the competition between Zn and other minerals for carriers,83 as well as significantly inhibited P uptake and the generation of Fe and Cu in the tissues at high concentrations.84 The imbalance of nutrient elements in plants may be associated with the transcription of related genes. For instance, exposure to ZnO NPs up-regulated the relative abundance of genes encoding nutrient element transporters in Solanum lycopersicum leaves, including heavy metals, ammonium, K, Ca, and S.52 Transcriptomic data also indicated that ZnO NPs triggered high levels of genes participating in Fe homeostasis and transportation in Arabidopsis thaliana, such as gene FERRIC REDUCTION OXIDASE 1 (FRO1)/2/3/5, bHLH38/39/101, IRT1/2, MYB DOMAIN PROTEIN 72 (MYB72), NRAMP4, OLIGOPEPTIDE TRANSPORTER 3 (OPT3), POPEYE (PYE), HMA2, and NAS2/4, whereas the expression of the HIGH AFFINITY K+TRANSPORTER 5 (HAK5) was significantly down-regulated.61 The variation in macronutrients and micronutrients in plants hindered the synthesis and accumulation of proteins and sugars, subsequently affecting normal metabolism and growth. It was also confirmed that ZnO NPs under hydroponic conditions were more toxic than in soil cultivation and apparently reduced the levels of P accumulation and protein formation in Zea mays L. tissues due to the uptake and translocation of metals.85 There was also a linear decrease in proteins and sugars in Oryza sativa L. leaves with the increase in concentrations of ZnO NPs46 and a gradual reduction in total soluble sugars in Arabidopsis thaliana.86 In addition to the typical nutrient elements that are disturbed, the molecular synthesis and transformation of other nutritional substances are indeed affected in varying degrees and are intimately linked to the physiological functions and stress tolerance of plants as discussed subsequently.
3.4 Perturbation of the photosynthesis system
The toxic response of ZnO NPs to plant leaves is largely attributed to reduced photosynthetic pigments and impaired chloroplast structure. For example, it was shown that chlorophyll and carotenoid contents in Vigna angularis leaves were significantly diminished by the ZnO NP treatment,87 thereby inhibiting plant growth and development. Priester et al. observed that chlorophyll a and b of leaves in Glycine max L. at harvest were markedly lower than that of the control under ZnO NP stress,76 presumably owing to blocked chlorophyll biosynthesis and accumulation. The qRT-PCR results indicated an approximately 5-fold reduction in the abundance levels of the chlorophyll synthesis genes CHLOROPHYLL A OXYGENASE (CAO), CHLOROPHYLL SYNTHASE (CHLG), COPPER RESPONSE DEFECT 1 (CRD1), MAGNESIUM-PROTOPORPHYRIN IX METHYLTRANSFERASE (CHLM), and MG-CHELATASE SUBUNIT D (CHLD) in ZnO NP-treated Arabidopsis thaliana,77 confirming the inhibition of photosynthesis process by NPs. In addition, the chloroplast ultrastructure of Hordeum vulgare L. leaves was disrupted by irrigation application of ZnO NPs, such as extended distances between the cell wall and chloroplasts, swollen thylakoids, and loosed grana lamellae.34 After foliar spraying with high concentrations of ZnO NPs, the stomatal density of Phaseolus vulgaris L. leaves decreased and stomatal length increased, making it difficult to maintain the balance between stomatal conductance, net CO2 assimilation, and water use efficiency.88
Photosynthetic efficiency and photochemical systems were directly affected by the reduction of chlorophyll and damage to chloroplast structure induced by ZnO NPs. For example, exposure to ZnO NPs decreased net photosynthesis in Zea mays L. by 12%,89 reduced stomatal conductance, intercellular CO2 concentration, and transpiration rate by over 50% in Arabidopsis thaliana leaves,77 and inhibited the maximum photochemical efficiency of PSII, the quantum yield of photochemistry of PSII, the photochemical quenching, and the apparent electron transfer rate in a concentration-dependent manner.90 The ZnO NP treatment also significantly interfered with the expression levels of photosynthetic structural genes PHOTOSYSTEM I SUBUNIT D-2 (PSAD2), PHOTOSYSTEM I SUBUNIT E-2 (PSAE2), and PHOTOSYSTEM I SUBUNIT K (PSAK) in Arabidopsis thaliana.77 Genes encoding integral membrane proteins including psaA, psbA1, and psbD1 in algal cells were also disturbed by ZnO NPs, and their mRNA transcripts may affect cellular PSI and PSII activities,91 inhibiting the electron transfer and photosynthetic reaction in chloroplasts. Studies have also emphasized the significant enrichment of differentially regulated proteins in the photosynthesis pathway in Hordeum vulgare L. leaves under ZnO NP stress,34 and the upward accumulation of proteins related to the photosystem and electron transport chain in Phaseolus vulgaris L.,88 which influence the photosynthesis pathway in plants. The physiological process of photosynthesis supports the life activities of plants and exhibits extreme sensitivity in the face of external threats; it is not only a source of energy for the transformation of substances, but also a pivotal basis for the maintenance of molecular, cellular, and phenotypic growth.
3.5 Oxidative stress and antioxidant defense
Oxidative stress is considered to be a potential mechanism for NPs inducing phytotoxicity,92 and plants can produce various species of enzymatic and non-enzymatic antioxidants as detoxification mechanisms.93 Excess ROS produced by abiotic stresses lead to membrane lipid breakdown and antioxidant inactivation, ultimately inhibiting cell proliferation and causing cell death.44 To prevent oxidative imbalance, plants have evolved antioxidant-mediated defense systems that control ROS production and scavenging to protect cells from oxidative damage.93 The production of ROS is both a sign of toxicity and a manifestation of tolerance responding to stress, which is a spontaneous active repair and defense mechanism in plants. This in vivo mechanism is a naturally evolving process of life activity that remains undefined at numerous functional sites due to interactive variations of diverse genes and metabolites when resisting abiotic stresses. In contrast, oxidative stress can act as a mediator to explain the biotransformation process of the defense system, especially as metabolism, transcription, and genes are able to fully reveal the signaling linkages of ROS generation, transport, and scavenging at the physiological and molecular levels.
Previous studies have found that ZnO NPs changed mitochondrial membrane potential (Ψm), induced ROS generation in a concentration-dependent manner, and caused mitochondrial swelling at higher exposure doses.64 It has been shown that Zn2+ dissolved from ZnO NPs entering cells may bind to the negatively charged portion of protein biomolecules and generate ROS that may cause cellular damage.94 Sharp imbalance in ROS levels, malondialdehyde (MDA) accumulation and increased electrolyte leakage (EL) were observed in Oryza sativa L., while propidium iodide staining provided further evidence of cellular damage, rupture, and loss of integrity triggered by ZnO NPs (Fig. 5d).46 The expression of miR166l and miR156b regulating ROS homeostasis was significantly decreased in leaves under ZnO NP stress, which may be related to the high transcript abundance of the target genes POLYAMINE OXIDASE 3 and PHOSPHOLIPASE D ALPHA 1 (PLDα1), and subsequently accompanied by severe oxidative burst.53 Furthermore, cell cycle analysis indicated that ZnO NP treatment increased the apoptotic sub-G1 population by 22.4% and decreased the number of G2/M cells compared to the control, implying that ZnO NPs induced Zea mays L. cell death by triggering apoptosis.85 Subsequently, SOD and CAT activities were significantly intensified with increasing exposure concentrations of ZnO NPs, with maximum stimulation rates reported to be 15% and 36%, respectively.46 The ascorbate peroxidase (APX) activity in Vigna angularis87 and Brassica chinensis L.84 was also significantly elevated in response to ZnO NP stress. A low dose of 50 mg L−1 ZnO NPs under soil application increased ascorbic acid (AsA) content in leaves by about 23% compared to the control but then decreased it by around 29% at a high concentration of 250 mg L−1, whereas the opposite result was obtained with leaf spraying of ZnO NPs.95 In brief, the activation of a series of SOD homologous enzymes decomposes excessive superoxide anion radicals (·O2−) to H2O2, immediately followed by the reaction of H2O2 with CAT to produce H2O and O2, or the reaction to form MDA and H2O under the adduction of APX and AsA,34 or the conversion to hydroxyl radicals (·OH) by the Fenton-like reaction.96 The antioxidant process is the standard paradigm for counteracting oxidative stress and scavenging ROS accumulation as a defense system against abiotic disturbances.
More importantly, plants can regulate the activity of antioxidant enzymes to maintain the oxidative balance of cells by modifying gene expression. Studies have shown that high concentrations of ZnO NPs promoted the transcription of APX2 and Fe-SOD genes in Solanum lycopersicum,90 and increased the transcriptional levels of genes encoding CAT (CATa and CATb) by more than 2-fold and antioxidant enzyme genes CSD1, CSD2, FSD1 and MSD1 by 2-4-fold in Oryza sativa L. roots.59 The APXb gene was significantly up-regulated under ZnO NP stress, whereas the transcription of the APXa gene was remarkably down-regulated, implicating that both paralogous homologous APX genes may perform distinct functions to help fine-tune the defense system to sustain homeostasis.59 Transcriptomics and metabolomics analyses also demonstrated that foliar spraying of ZnO NPs elevated the transcription of genes encoding antioxidant enzymes and transporter proteins, and up-regulated the abundance of sugars and amino acids in Solanum lycopersicum (Fig. 6).52 GO bioprocess analyses indicated that pathways involved in ROS metabolism and antioxidant enzyme activities were enriched in ZnO NP treatment, as evidenced by a significant up-regulation of the relative abundance of peroxidase (POD) genes and glutathione S-transferase genes.52 Although the relative levels of glutathione reductase (GR) genes were markedly increased under ZnO NP stress, the transcript abundance of APX-1, Cu/Zn SOD, MDAR, and DHAR participating in the ascorbate–glutathione cycle showed a decreasing trend.53 ZnO NPs also diminished the activities of apoplastic peroxidases (cwPOX), GR, and vacuolar invertase (vacInv) in Hordeum vulgare L. by 26.09%, 32.87%, and 24.40%, respectively (Fig. 5e),34 and the antioxidant glutathione (GSH) content of Fagopyrum esculentum seedlings treated with high-dose NPs was less than that of low-dose ones.75 These findings were largely attributed to the excessive ROS production induced by ZnO NPs that exceeded the scavenging capacity of plants, resulting in the dysregulation of antioxidant and carbohydrate metabolism systems and triggering severe oxidative damage.
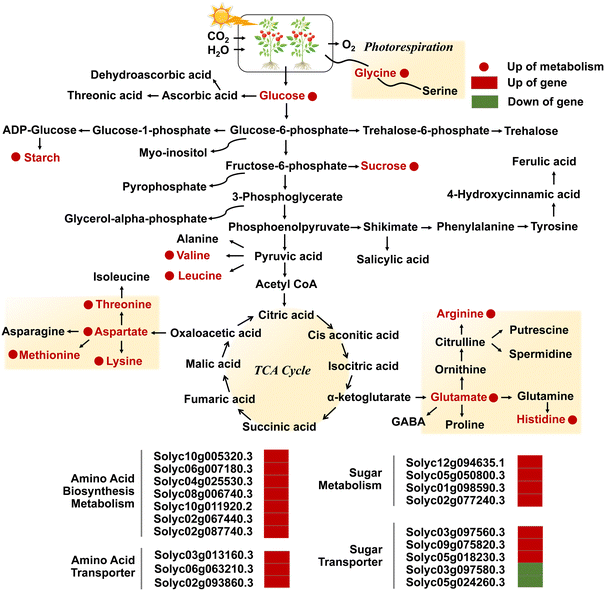 |
| Fig. 6 Diagram of the metabolic pathway in the TCA cycle of the KEGG pathway, and transcriptional regulation of metabolism and transport of amino acids and sugars in leaves exposed to NPs. Adapted with permission from ref. 97. Copyright (2018) American Chemical Society. Red and green colors represent up- and downregulation, and circles and rectangles represent metabolites and genes, respectively. Adapted with permission from ref. 52. Copyright (2020) Royal Society of Chemistry. | |
The component identification of ROS response is closely linked to stress signaling and hormone synthesis,98 and the regulation of this biological process can enhance tolerance against oxidative stress induced by ZnO NPs. The bioaccumulation of melatonin (MT) and its precursors (e.g., serotonin, tryptamine, and tryptophan) in Oryza sativa L.,99 glucosinolates (GSs) in Arabidopsis thaliana,100 proline in Zea mays L.85 and Phaseolus vulgaris L.,88 and AsA in Solanum lycopersicum fruits101 was observed to be increased following ZnO NP treatment, while the mRNA expression of the relevant synthesized genes was also elevated.100 Exposure to ZnO NPs also up-regulated or down-regulated the levels of amino acids, organic acids, sugars, and glycosides, and changed the synthesis of secondary metabolites involved in antioxidant defense, such as flavonols.102 ZnO NPs also accelerated ethylene biosynthesis by up-regulating the transcription of genes in the ethylene metabolism pathway, whereas the ethylene aggravated the toxic responses of ZnO NPs and induced more severe stomatal closure and ultrastructural damage by causing oxidative stress in Oryza sativa L. seedlings.103 Generally, the biochemical response processes involved in ROS can modulate metabolic pathways of primary and secondary metabolic changes, while further enhancing the stress tolerance of plants to maintain the balance between growth and defense/stress responses.
3.6 Disorder of the metabolic pathway
As a crucial mechanism for responding to abiotic stresses, metabolic regulation is essential in resisting the toxicity induced by ZnO NPs. The down-regulation of carbohydrate metabolism and up-regulation of amino acid metabolism represent two crucial metabolic pathways regulated by NPs,104 especially the glycolysis pathway and TCA cycle mediate the generation of energy metabolism.105 Differential gene responses in glutathione metabolism and phytohormone signal transduction pathways may also be able to enhance tolerance in plants.106 The Database for Annotation, Visualization and Integrated Discovery (DAVID) analysis also revealed that ZnO NPs induced severe dysregulation of glycolysis, gluconeogenesis, and fatty acid biosynthesis pathways in algal cells.79 The application of ZnO NPs significantly up-regulated the levels of organic acids (e.g., citric and malic acids) involved in the TCA cycle in Cucumis sativus L. seedlings,102 and dose-dependently altered the metabolomic profiles of Phaseolus vulgaris L.88 The expression levels of genes engaged in sugar transport and metabolism, amino acid transport, biosynthesis and metabolism, as well as phenylpropanoid metabolic pathways in Solanum lycopersicum were regulated by ZnO NPs, such as the Solyc03g097560.3 gene encoding bidirectional sugar transporter SWEET14 and the Solyc02g077240.3 gene encoding pyruvate decarboxylase 1 (Fig. 6).52 The global metabolomic analysis further confirmed that ZnO NPs regulated carbon/nitrogen metabolism and secondary metabolism by augmenting the levels of sucrose, starch, glucose, and amino acids such as glycine, L-histidine, and L-isoleucine.52 Zou et al. also demonstrated that ZnO NPs altered metabolites associated with molecular regulation, signal transduction, and metabolic pathways in Nicotiana tabacum L., consisting of alkaloids, flavonoids, phenylpropanoids, amino acids, nucleotides and their derivatives.82 Flavonoid compounds can bind to metal ions to form flavonoid metal chelates with strong superoxide scavenging activity as an effective defense against the toxic effects of ROS.107 More notably, drastic changes in metabolic levels ultimately lead to a high accumulation of degradation products of phytohormones or stress-related compounds (such as lignin and GSs).88 It can be seen that comprehensive mining of metabolic information in plants is an essential basis for clarifying the toxicity regulation of NPs, and its deeply resolved profiles can probe the metabolites that are indispensable for growth, development and life activities, so as to elucidate the equilibrium of resilience and the molecular mechanism of the KEGG pathway.
3.7 Genotoxicity and DNA damage
Previous studies have emphasized the potential genetic damage and genotoxicity caused by ZnO NPs in plants, which is strong and intuitive evidence for characterizing cytotoxicity at the molecular level. For instance, as the dose and exposure time of green-synthesized ZnO NPs increased, the mitotic index (MI) of Hordeum vulgare L. decreased significantly with the appearance of diverse aberrant chromosomes.108 ZnO NPs also diminished MI of Allium cepa L. bulbs by 23 ± 8.7%,64 augmented the micronuclei index and caused chromosomal aberrations including stickiness, bridges, vagrant chromosomes, multipolarity, and fragment types.73 Moreover, ZnO NPs greatly impeded plant development and inhibited the cell division progression by down-regulating the expression of cell cycle–related genes (CYCA2-1, CYCB1-1, CYCD1-1, CYCD2-1, and E2FB) and decreasing the level of their mRNA transcripts in Arabidopsis thaliana, while ethylene signaling and biosynthesis were involved in the reaction.86 Deep sequencing and GO analysis also confirmed that differentially expressed miRNAs and their potential targets precisely regulated the transcription of stress-responsive genes to participate in the adaptive response of plants against ZnO NP stress.53 The expression of genes encoding ribosomal proteins and histones of Arabidopsis thaliana was significantly down-regulated, strongly repressing the translation process and altering DNA package and transcriptional regulation, which appear to be a stronger role for Zn2+ compared with ZnO NPs.109 The results of the comet assay also indicated that ZnO NPs aggravated DNA damage in Allium cepa L. cells,110 whereas the shifts of the characteristic peaks at 1240 and 1060 cm−1 further revealed the perturbation of the phosphate backbone and the C–O bond between the ribose/deoxyribose of nucleic acids (DNA and RNA).64 A new DNA band was detected in the random amplified polymorphic DNA (RAPD) profiles of Glycine max L. roots treated with ZnO NPs, presumably the disruption of DNA integrity may have a negative impact on the genetic material of plants.111 The prolonged exposure to ZnO NPs also increased polymorphism rates and cytosine methylation in mature Triticum aestivum L. embryos, while diminishing genomic template stability values and inducing genotoxic potential of DNA methylation and genomic instability.112 The damage to chromosomes and DNA in plant cells is quite difficult to repair, and cell death will be triggered once it reaches a completely irreversible state, with this property even carrying genetic properties. Knocking out specific genes to delve deeper into the molecular pathways of toxicity modulation is immensely complicated; nevertheless, the screening of resistance genes is of great value in resisting the nanotoxicity of NPs. Collectively, based on the discussion of the environmental fate of ZnO NPs in plants, the response mechanisms of toxicological effects are summarized and plotted (Fig. 7), and this provides a theoretical reference for the study of the toxicity regulation and potential risks of NPs.
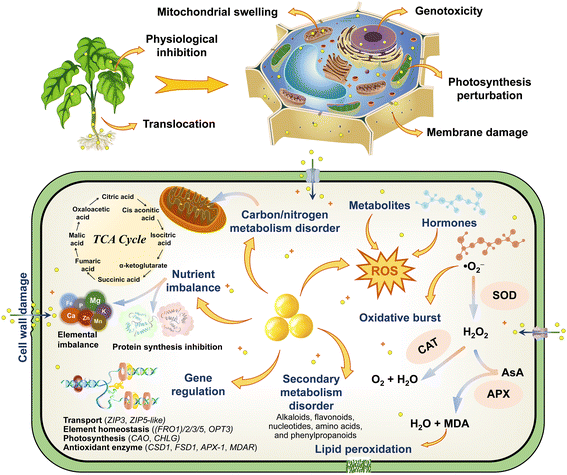 |
| Fig. 7 Schematic illustration of the toxicity responses and regulatory mechanisms of ZnO NPs in plants. | |
4 Uncertain ecological risks of NP composite systems to plants
The released ZnO NPs inevitably undergo adsorption, condensation and complexation effects with other substances in the environment and induce antagonistic or synergistic interactions on phytotoxicity, which pose a new challenge for the uncertain ecological risk of NPs in composite systems. As an example, heavy metals, humic substances, and acetic acid in leachate affect the aggregation, electrostatic adsorption, and ion release of ZnO NPs, especially Cr(III) ions binding to hydroxyl groups on ZnO to generate ZnO–O bonds.113 In addition to the combined exposure of multiple nanomaterials,114 ZnO NPs can also adsorb with microplastics115 but are limited by Zn2+,116 as well as stimulate surface oxidation and degradation of microplastics.117 Considering that ZnO NPs can act as both bioregulator products and exogenous nano-pollutants, the unknown risks to plants induced by exposure to multiple systems may be either toxicity mitigation or toxicity exacerbation (Table 1), implying that the field needs to be supported by more adequate research in the future.
Table 1 Typical examples of mitigative or synergistic effects on plants by composite systems of ZnO NPs and other substances
Plants |
ZnO NP concentration |
Composite substance: concentration |
Mitigative or synergistic effects |
Ref. |
Vigna mungo L. |
50, 100, 150 and 200 mg L−1 |
As: 10 mg L−1 (available as 2.4 mg L−1) |
Elevated seed germination, stabilized physiological morphology, and mitigated metal toxicity of Cd by impeding its migration from roots to shoots |
35
|
Linum usitatissimum L. |
100, 500, and 1000 mg L−1 |
Cd: 100 mg kg−1 |
Reduced the levels of MDA, H2O2 and EL, and increased SOD, POD, CAT, and APX activities to mitigate Cd stress |
139
|
Phytolacca americana L. |
500 mg kg−1 |
Cd: 10 and 100 mg kg−1 |
Stimulated aggregation of ZnO NPs/Cd in the root cytoderm, impeded the root growth, activated the antioxidant system to resist stress, and evoked root cell damage |
135
|
Polyploid and diploid Oryza sativa L. |
25 mg L−1 |
Cd: 100 μmol L−1 |
Enhanced biochemical reaction processes of antioxidant enzymes, altered gene levels of metal transporter proteins and showed greater resistance in polyploidy |
43
|
Fragrant Oryza sativa L. varieties |
25, 50 and 100 mg L−1 |
Cd: 100 mg L−1 |
Increased fresh weight of the whole seedlings, heightened expression of metallothionein and α-amylase, and modulated metabolites of alanine and phenylpropanoid biosynthesis |
140
|
Perilla frutescens
|
100 mg L−1 |
Cd: 5 μmol L−1 |
Accumulated mineral elements, improved photosynthetic ability, and up-regulated the expression of intermediates (amino acids and organic acids) of the TCA cycle |
120
|
Solanum lycopersicum
|
50 mg L−1 |
Cd: 5 μmol L−1 |
Decreased heavy metal accumulation, alleviated the metabolic perturbations induced by Cd, adjusted micronutrient element levels, and stabilized normal growth |
122
|
Spinacia oleracea, Petroselinum sativum, and Coriandrum sativum |
100 mg L−1 |
Cd: 1 mg L−1 |
Diminished uptake and bioavailability of Cd and Pb in roots, affected Cu and Fe generation, and exhibited variability in edible parts of different plants |
141
|
Pb: 100 mg L−1 |
Triticum aestivum L. |
50 and 100 mg kg−1 |
Cd: 3.14 mg kg−1 |
Reduced Cd content in roots, shoots, husks, and grains, and increased plant elongation and photosynthetic pigments with exogenous MT |
121
|
MT: 50 and 100 μmol L−1 |
Nicotiana tabacum
|
50 mg L−1 |
Cd: 5 μmol L−1 |
Promoted the seedling elongation, altered KEGG metabolic pathways, and induced typical amino acid, arginine, and proline metabolism |
82
|
Zea mays L. |
500 mg L−1 |
Co: 300 μmol L−1 |
Facilitated physiological recovery of tissues, including plant growth, ultrastructural status, antioxidant system, and photosynthetic efficiency |
124
|
Oryza sativa L. |
25 μmol L−1 |
Cr(VI): 100 μmol L−1 |
Elevated plant biomass, augmented photosynthesis levels, inhibited ROS burst, and improved the ascorbate-glutathione cycle |
125
|
Sophora alopecuroides L.
|
50 and 100 mg L−1 |
NaCl: 100 mmol L−1 |
Reprogramed the carbon/nitrogen metabolism, fostered glycolysis and the TCA cycle, and enhanced unsaturated fatty acids contents to maintain membrane integrity |
105
|
Chlorella vulgaris
|
10 mg L−1 |
TBBPA: 0.5, 4 and 8 mg L−1 |
Binary NP-TBBPA promoted NP uptake and disrupted cellular ultrastructure, and the addition of HA attenuated lipid peroxidation damage and enhanced tolerance |
36
|
HA: 10 mg L−1 |
Phaseolus vulgaris L.
|
20 and 50 mg kg−1 |
MTZ: 2 and 3 mg kg−1 |
Decreased ammonium oxidase activity, inhibited photosynthetic pigment levels, and affected MTZ accumulation and metabolization in plants |
133
|
Zea mays L. |
50 and 500 mg kg−1 |
HDPE and polylactic acid: 0.1%, 1%, and 10% w/w |
Induced Zn accumulation in root tissues, stimulated biomass variation, influenced plant fitness, and changed the components of the arbuscular mycorrhizal (AM) |
134
|
Hordeum vulgare L. |
500 mg L−1 |
Nanoplastic (PMMA): 1000 mg L−1 |
Altered sites of phosphorylated proteins, interfered with carbohydrate synthesis pathways, and exacerbate photosynthesis injury and metabolic disorder |
34
|
Eremosphaera viridis
|
1, 5, and 10 mg L−1 |
CNCs: 100 mg L−1 |
Intensified bioavailability and toxicity, and evoked intense plasma membrane breakdown, protein folding, and cell damage |
44
|
Pisum sativum L. |
100 and 200 mg L−1 |
Cerium oxide (CeO2) NPs: 100 and 200 mg L−1 |
Inhibited elongation of roots and stems, limited stomata and transpiration of leaves, and affected the balance of mineral elements |
142
|
Oryza sativa L. |
500 mg L−1 |
Ethylene biosynthesis precursor (ACC): 20 μmol L−1 |
Disturbed cellular structure and the plastoglobuli, interfered with antioxidant enzyme synthesis and gene transcription, and intensified the combined toxicity |
103
|
Triticum aestivum L. |
300, 600, 900, and 1200 mg kg−1 |
Gibberellic acid (GA): 100 and 200 mg L−1 |
Alleviated photosynthetic pigment inhibition, promoted nutrient element accumulation, and diminished Zn accumulation and ROS generation |
143
|
Oryza sativa L. |
100, 500, and 1000 mg kg−1 |
MT: 100 μmol L−1 |
Reversed physiological inhibition, limited ROS production, up-regulated synthesis of tetrapyrrole and amino acids, and expanded levels of antioxidant gene response |
99
|
Chlorella sorokiniana
|
0.1 and 1 mmol L−1 |
Phosphate: P/Zn molar ratios of 0.5, 1, and 2 |
Relieved the growth inhibitory effects, improved cell membrane integrity, and up-regulated gene expression for photosynthesis |
91
|
Chenopodium murale L. |
50 mg L−1 |
Salicylic acid (SA): 25, 75, and 150 μmol L−1 |
Repaired the function of the antioxidant enzyme system, increased the expression of phenolic compounds, and decreased the transfer of NPs from roots to shoots |
144
|
Arabidopsis thaliana
|
40 and 50 mg L−1 |
MWCNTs: 100 and 500 mg L−1 |
Inhibited several growth indicators, synergistically or additively regulated the activity of antioxidant enzymes, and deteriorated ROS accumulation in shoots |
136
|
4.1 Toxicity mitigation of other substances induced by ZnO NPs
The industrialized ZnO NPs have the potential to considerably reduce the toxic response of other pollutants as beneficial environmental carriers. Previous studies have demonstrated that ZnO NPs exhibit great potential in mitigating phytotoxicity under heavy metal stress.118,119 For example, ZnO NPs enhanced the photosynthetic capacity of seedlings under Cd stress and elevated the levels of stress-related organic acid metabolites (e.g., citric, malic, and maleic acids) and amino acids (e.g., arginine, glutamate, and phenylalanine),120 while the combination of soil-applied ZnO NPs and foliar-applied MT caused the minimization of Cd in the edible parts and the biofortification of Zn.121 Metabolic fluctuations in cells triggered by Cd and bioaccumulation of heavy metals were mitigated by the addition of ZnO NPs,122 and ZnO NPs–Cd interactions also reduced the bioavailability of NPs by inducing a massive release of root secretions.123 The seed initiation of ZnO NPs also attenuated the toxic effects of the metal by diminishing Co uptake and enhancing the stability of the ultrastructures and photosynthetic apparatus.124 The antioxidant genes OsAPX, OsDHAR, OsMDHAR and OsGR were also significantly up-regulated after ZnO NP treatment, thus inducing a decrease in the levels of ·O2− and H2O2 oxidants and an elevation in the levels of the nonenzymatic antioxidants AsA and GSH. This rendered tolerance to the stress imposed by Cr(VI) exposure in Oryza sativa L. seedlings, activated defense mechanisms, and reversed the inhibition of photosynthetic efficiency and growth.125 Similarly, biogenic ZnO NPs limited As translocation from roots to shoots, reduced ROS and MDA accumulation, and increased osmoregulator content and antioxidant enzyme activities to effectively mitigate As stress.35 Interestingly, the interaction of titanium dioxide (TiO2) NPs and ZnO NPs in the algal-fish food chain inhibited the uptake of elemental Ti and Zn by algae (Asterococcus superbus), and also significantly diminished Ti and Zn contents in fish tissues.18 In addition, field studies found that the combination of foliar spraying of ZnO NPs and seed inoculation with Bacillus subtilis increased the levels of photosynthetic pigments, total soluble sugars, amino acids, and proteins (e.g., grain glutelin and prolamin) to improve metabolism and productivity attributes of Zea mays L.126 The co-application of brassinosteroid and ZnO NPs also maintained cellular redox homeostasis by increasing the ratio of reduced glutathione to oxidized glutathione (GSH/GSSG), relative transcript abundance of antioxidant genes Cu/Zn SOD, CAT1, GSH1, and GR1 and antioxidant enzyme activities in seedlings.127
Dual exposure to environmental factors also needs to be considered. In the face of salinity threats, ZnO NPs modified carbon/nitrogen metabolic processes in Sophora alopecuroides L. and stimulated the expression of unsaturated fatty acids to sustain membrane integrity and resist salt stress, whereas the glycolysis pathway and TCA cycle played a major role in supplying energy.105 ZnO NPs mitigated the damage to the morphophysiology and membrane stability of Medicago sativa L. under heat stress, which might be attributed to the antioxidant system activation and osmolyte accumulation.128 Foliar spraying of ZnO not only attenuated the decline in phenol and mineral nutrient levels in Cucumis sativus L. seedlings under drought stress,129 but also helped improve the chilling effect on photosynthesis in Saccharum officinarum L.130 Exposure of ZnO NPs mitigated oxidative damage, increased levels of metal nutrients, and enhanced enzymes or regulators engaged in carbon/nitrogen and secondary metabolisms in response to Fe deficiency in Solanum lycopersicum.52 From most of the above-mentioned studies, it can be found that ZnO NPs are able to reduce the toxicological effects and risks of heavy metals and environmental stresses on plants and exhibit mitigation strategies, which provides a favorable prospect for the application of nanotechnology in agriculture.
4.2 Combined toxicity caused by ZnO NPs with other substances
Undoubtedly, it is also extremely possible that the interaction of ZnO NPs with environmental mediators exhibits synergistic effects131 and exacerbates the combined toxicity of binary NPs.44 This is closely associated with the surface properties of ZnO NPs and ecological attributes of model plants, giving rise to differential variations in sensitivity and tolerance. Considering the complexity and biochemical reactions of contaminants in the natural environment, binary or even multivariate composite systems of NPs raise more concerns about ecotoxicity and risk.132 The inclusion of ZnO NPs in the soil presenting the herbicide metribuzin (MTZ) impeded the fresh weight of Phaseolus vulgaris L. and altered the metabolism of MTZ in the root tissues.133 Although high-density polyethylene (HDPE) and low-dose polylactic acid (PLA) promoted the growth of Zea mays L. in soil spiked with ZnO NPs, high doses of PLA significantly lowered the shoot and root biomass, enhanced the accumulation of Zn in the roots, and depressed Zn translocation to the aerial parts.134 Individual exposure to ZnO NPs or low-dose Cd failed to interfere with normal plant growth, but combined treatment with ZnO NPs and Cd increased the accumulation of NPs and Cd in cells, induced severe cellular damage and inhibited the growth of Phytolacca americana L. roots by up to 43%.135 The presence of naturally sourced cellulose nanocrystals (CNCs) also augmented the bioavailability and toxicity of NPs to the Eremosphaera viridis by diminishing the aggregation of ZnO NPs, where the toxicity mechanisms exhibited strong oxidative stress, disturbed elemental distribution patterns, disrupted enzyme activities, and catabolic lipid molecules.44
Similarly, the synergistic effect of ZnO NPs and multi-walled carbon nanotubes (MWCNTs) altered genes that maintain cellular ROS homeostasis to regulate the activities of antioxidant enzymes such as SOD, CAT, and GR,136 while the coexistence of ZnO NPs with nanoplastics increased abscisic acid (ABA) and H2O2 concentrations in Hordeum vulgare L. and caused stronger damage to antioxidant and carbohydrate metabolism systems.34 Polystyrene (PS) also aggravated structural damage to the cell wall of Chlorella vulgaris, facilitated the transfer of ZnO NPs through the food chain, and increased the ecological risk of NPs.137 Binary ZnO and tetrabromobisphenol A (TBBPA) in the water bodies produced structural damage and high levels of ROS in algal cells, whereas the addition of humic acid (HA) alleviated cytotoxicity and membrane perturbation.138 The interaction of ZnO NPs with triclosan (TCS) in personal care products also led to the entry of more NPs into algal cells by increasing the permeability of cell membranes, thus enhancing the trophic transfer and combined toxicity of NPs along the food chain.18 In conclusion, in-depth studies on the uptake, transport, and toxicity regulation of NPs can provide new insights into environmental health and ecological safety, and provide a theoretical basis for accurately predicting the uncertain risks triggered by NPs in composite systems to plants.
5 Conclusions and research insights
This review summarized the molecular mechanisms of transportation and toxicity regulation of ZnO NPs and emphasized the mitigating effects and combined toxicity of composite systems of NPs on plants. The results indicated that ZnO NPs were absorbed and transported to plant tissues via the apoplastic and symplastic pathways, especially the up-regulation of metal transporter proteins and gene expression also further illustrated the migration and accumulation of NPs in cells. The reduced seed germination, inhibition of rhizome elongation, decreased biomass and phenotypic structural damage induced by ZnO NPs affected the growth and development. The internalized ZnO NPs interacted with plants at cellular and subcellular levels and triggered nutrient component imbalance, photosynthetic impairment, oxidative stress response, metabolic disorders and genotoxicity, which revealed a crucial mechanism for the toxicological effects of NPs. The generation and scavenging of ROS dominate the toxicity of ZnO NPs and are the focus of exploring detoxification and tolerance mechanisms, while the activation of antioxidant systems plays a key role in maintaining oxidative homeostasis. In addition, composite exposures of ZnO NPs with other substances induce complicated antagonistic or synergistic phytotoxicity, posing uncertain potential risks to the ecological safety of plants. Overall, this review elucidated the fate of ZnO NPs in plants and provided a comprehensive perspective on the environmental behavior and toxicity studies of NPs in natural ecosystems.
Owing to technological advances, NPs are one of the matrices of precisely engineered chemicals and materials that can both improve the growth of plants and threaten their survival. Faced with the challenges of emerging materials, we should initiate studies on nanotoxicity based on previous strategic research goals to gain a comprehensive understanding of the determinants of NP behavior in plants and determine how to manipulate such toxicity, thereby ultimately minimizing the risks and maximizing the benefits. To achieve this objective more efficiently, we propose the following critical research directions and insights:
(1) The understanding of mechanisms by which NPs interact with plants requires sufficient knowledge prior to strategic research and prospective deployment. The essential toxicity mechanisms include (a) the biomolecular behavior and dynamic corona variation in the migration and translocation of NPs in plants, (b) the effects of surface properties and external environmental conditions of NPs on their transport efficiency in cellular internalization, (c) the cellular dynamics and mechanisms of NPs triggering toxicity responses, especially deep-learning models of ROS production and scavenging, and (d) the life cycle responses induced by NPs at the cellular, biochemical, and molecular levels, involving global reprogramming of genomics and metabolomics. The interactive approaches of fluorescence localization, immunohistochemistry, transcriptomics, proteomics, metabolomics, ionomics, and epigenetics are compelling tools to address these issues.
(2) In-depth development for predicting potential hazards and risks is also a critical component. Currently, the research on the prediction and management of the nanotoxicity of NPs is still in the infancy stage. On the one hand, there are still enormous challenges in the exploitation of smart sensors for detecting the exposure hazards and potential risks of NPs to organisms, which involves comprehensive exposure monitoring and potential toxicity assessment. On the other hand, effective models that can predict the behavior and potential effects of NPs in the environment and organisms are competent support for risk assessment. These exploits encompass the release, migration, and transformation of NPs in the environment, as well as the dosage, transport, clearance, accumulation, transformation, and response in organisms. Based on these studies, the combination of the properties and behaviors of NPs may be able to more accurately forecast potential impacts on the environment and organisms.
(3) Currently, the supervision of nanomaterials is very limited, even in countries with the largest production of NPs, such as the United States and China. There is an urgent necessity to find effective regulatory approaches to alleviate the ecological risks that may be caused by nano-pollution. The development of policies and laws for the application and management of NPs is essential to minimize the generation of nano-pollution and can address the problem at the source. The production, application, treatment and disposal of nano-products should be taken into consideration to minimize the introduction of NPs into the environment, e.g., by increasing product utilization and recovery rates and improving device containment. Besides, the exploitation of cost-effective and environmentally friendly nano-products or substitutes is also a feasible approach. In developing new nanomaterials and products, it is crucial to avoid harmful effects and fully utilize the advantages of NPs based on toxicity studies. For example, the “Safe-by-Design” concept,145 in which nanotoxicologists, chemists, and materials scientists collaborate to create safe nano-products, has been adopted. Propaganda and education can equally raise public, social and national awareness of NPs and enhance environmental consciousness from the human mind.
(4) The characterization of NPs in regulating ROS homeostasis could be a prospective strategy for improving the tolerance in plants and crop yield, while nano-stress tolerance responses also represent a rapidly developing research area across plant science and nanomaterials. ROS induced by NPs is a double-edged sword for plants, promoting growth as well as threatening survival. A pivotal factor in addressing this issue is how to manipulate ROS generation and scavenging caused by NPs. Therefore, the combination of generation and scavenging strategies could provide a versatile and effective solution for stress tolerance.
Author contributions
Mengen Kang: conceptualization, formal analysis, writing-original draft, writing-review & editing. Yi Liu: methodology, formal analysis, writing-original draft, writing-review & editing. Yuzhu Weng: conceptualization. Haoke Wang: formal analysis. Xue Bai: project administration, funding acquisition, supervision.
Conflicts of interest
There are no conflicts to declare.
Acknowledgements
The authors gratefully acknowledge the support provided by the National Natural Science Foundation of China (U2243601) and the Priority Academic Program Development of Jiangsu Higher Education Institutions.
References
- M. F. Hochella, D. W. Mogk, J. Ranville, I. C. Allen, G. W. Luther, L. C. Marr, B. P. McGrail, M. Murayama, N. P. Qafoku, K. M. Rosso, N. Sahai, P. A. Schroeder, P. Vikesland, P. Westerhoff and Y. Yang, Natural, incidental, and engineered nanomaterials and their impacts on the Earth system, Science, 2019, 363, eaau8299 CrossRef PubMed.
- B. Peng, F. Zhao, J. Ping and Y. Ying, Recent advances in nanomaterial-enabled wearable sensors: Material synthesis, sensor design, and personal health monitoring, Small, 2020, 16, 2002681 CrossRef CAS.
- S. Hassan, G. Prakash, A. Bal Ozturk, S. Saghazadeh, M. Farhan Sohail, J. Seo, M. Remzi Dokmeci, Y. S. Zhang and A. Khademhosseini, Evolution and clinical translation of drug delivery nanomaterials, Nano Today, 2017, 15, 91–106 CrossRef CAS.
- K. Y. Perera, S. Jaiswal and A. K. Jaiswal, A review on nanomaterials and nanohybrids based bio-nanocomposites for food packaging, Food Chem., 2022, 376, 131912 CrossRef CAS.
- D. Wang, N. B. Saleh, A. Byro, R. Zepp, E. Sahle-Demessie, T. P. Luxton, K. T. Ho, R. M. Burgess, M. Flury, J. C. White and C. Su, Nano-enabled pesticides for sustainable agriculture and global food security, Nat. Nanotechnol., 2022, 17, 347–360 CrossRef CAS.
- P. K. Rai, A. Rai, N. K. Sharma, T. Singh and Y. Kumar, Limitations of biofertilizers and their revitalization through nanotechnology, J. Cleaner Prod., 2023, 418, 138194 CrossRef CAS.
- Y. Ko, C. H. Kwon, S. W. Lee and J. Cho, Nanoparticle-based electrodes with high charge transfer efficiency through ligand exchange layer-by-layer assembly, Adv. Mater., 2020, 32, 2001924 CrossRef CAS.
- J. C. Hsu, Z. Tang, O. E. Eremina, A. M. Sofias, T. Lammers, J. F. Lovell, C. Zavaleta, W. Cai and D. P. Cormode, Nanomaterial-based contrast agents, Nat. Rev. Methods Primers, 2023, 3, 30 CrossRef CAS.
- J. M. V. Makabenta, A. Nabawy, C. H. Li, S. Schmidt-Malan, R. Patel and V. M. Rotello, Nanomaterial-based therapeutics for antibiotic-resistant bacterial infections, Nat. Rev. Microbiol., 2021, 19, 23–36 CrossRef CAS PubMed.
- F. Gottschalk, B. Debray, F. Klaessig, B. Park, J.-M. Lacome, A. Vignes, V. P. Portillo, S. Vázquez-Campos, C. O. Hendren, S. Lofts, S. Harrison, C. Svendsen and R. Kaegi, Predicting accidental release of engineered nanomaterials to the environment, Nat. Nanotechnol., 2023, 18, 412–418 CrossRef CAS.
- C. Svendsen, L. A. Walker, M. Matzke, E. Lahive, S. Harrison, A. Crossley, B. Park, S. Lofts, I. Lynch, S. Vázquez-Campos, R. Kaegi, A. Gogos, C. Asbach, G. Cornelis, F. von der Kammer, N. W. van den Brink, C. Mays and D. J. Spurgeon, Key principles and operational practices for improved nanotechnology environmental exposure assessment, Nat. Nanotechnol., 2020, 15, 731–742 CrossRef CAS.
- M. Murali, H. G. Gowtham, S. B. Singh, N. Shilpa, M. Aiyaz, M. N. Alomary, M. Alshamrani, A. Salawi, Y. Almoshari, M. A. Ansari and K. N. Amruthesh, Fate, bioaccumulation and toxicity of engineered nanomaterials in plants: Current challenges and future prospects, Sci. Total Environ., 2022, 811, 152249 CrossRef CAS.
- H. Singh, A. Sharma, S. K. Bhardwaj, S. K. Arya, N. Bhardwaj and M. Khatri, Recent advances in the applications of nano-agrochemicals for sustainable agricultural development, Environ. Sci.: Processes Impacts, 2021, 23, 213–239 RSC.
- D. M. Mitrano, K. Mehrabi, Y. A. R. Dasilva and B. Nowack, Mobility of metallic (nano)particles in leachates from landfills containing waste incineration residues, Environ. Sci.: Nano, 2017, 4, 480–492 RSC.
- J. Wielinski, A. Gogos, A. Voegelin, C. R. Müller, E. Morgenroth and R. Kaegi, Release of gold (Au), silver (Ag) and cerium dioxide (CeO2) nanoparticles from sewage sludge incineration ash, Environ. Sci.: Nano, 2021, 8, 3220–3232 RSC.
- J. Lv, P. Christie and S. Zhang, Uptake, translocation, and transformation of metal-based nanoparticles in plants: Recent advances and methodological challenges, Environ. Sci.: Nano, 2019, 6, 41–59 RSC.
- B. J. Shaw and R. D. Handy, Physiological effects of nanoparticles on fish: A comparison of nanometals versus metal ions, Environ. Int., 2011, 37, 1083–1097 CrossRef CAS PubMed.
- X. Xin, G. Huang, B. Zhang and Y. Zhou, Trophic transfer potential of nTiO2, nZnO, and triclosan in an algae-algae eating fish food chain, Aquat. Toxicol., 2021, 235, 105824 CrossRef CAS PubMed.
- Y. Ma, Y. Yao, J. Yang, X. He, Y. Ding, P. Zhang, J. Zhang, G. Wang, C. Xie, W. Luo, J. Zhang, L. Zheng, Z. Chai, Y. Zhao and Z. Zhang, Trophic transfer and transformation of CeO2 nanoparticles along a terrestrial food chain: Influence of exposure routes, Environ. Sci. Technol., 2018, 52, 7921–7927 CrossRef CAS PubMed.
- N. K. Geitner, S. M. Marinakos, C. Guo, N. O'Brien and M. R. Wiesner, Nanoparticle surface affinity as a predictor of trophic transfer, Environ. Sci. Technol., 2016, 50, 6663–6669 CrossRef CAS PubMed.
- F. Dang, Y. Huang, Y. Wang, D. Zhou and B. Xing, Transfer and toxicity of silver nanoparticles in the food chain, Environ. Sci.: Nano, 2021, 8, 1519–1535 RSC.
- Y. Huang, X. Bai, C. Li, M. E. Kang, Y. Weng and D. Gong, Modulation mechanism of phytotoxicity on Ipomoea aquatica Forssk. by surface coating-modified copper oxide nanoparticles and its health risk assessment, Environ. Pollut., 2022, 314, 120288 CrossRef CAS.
- Z. Li, X. Liu, M. Zhou, S. Zhang, S. Cao, G. Lei, C. Lou and J. Zhang, Plasma-induced oxygen vacancies enabled ultrathin ZnO films for highly sensitive detection of triethylamine, J. Hazard. Mater., 2021, 415, 125757 CrossRef CAS PubMed.
- A. Singh, N. B. Singh, S. Afzal, T. Singh and I. Hussain, Zinc oxide nanoparticles: A review of their biological synthesis, antimicrobial activity, uptake, translocation and biotransformation in plants, J. Mater. Sci., 2017, 53, 185–201 CrossRef.
- C. Wang, J. Xie, X. Chang, W. Zheng, J. Zhang and X. Liu, ZnO single nanowire gas sensor: A platform to investigate the sensitization of Pt, Chem. Eng. J., 2023, 473, 145481 CrossRef CAS.
- W. Ouyang, J. Chen, Z. Shi and X. Fang, Self-powered UV photodetectors based on ZnO nanomaterials, Appl. Phys. Rev., 2021, 8, 031315 CAS.
- H. Ghaffari, A. Tavakoli, A. Moradi, A. Tabarraei, F. Bokharaei-Salim, M. Zahmatkeshan, M. Farahmand, D. Javanmard, S. J. Kiani, M. Esghaei, V. Pirhajati-Mahabadi, S. H. Monavari and A. Ataei-Pirkooh, Inhibition
of H1N1 influenza virus infection by zinc oxide nanoparticles: Another emerging application of nanomedicine, J. Biomed. Sci., 2019, 26, 70 CrossRef PubMed.
- S. Shamaila, A. Jalil, M. Ishfaq and R. Sharif, Nano-technological aspects of zinc oxide and silver in cosmetics, J. Appl. Phys., 2022, 131, 164306 CrossRef CAS.
- I. Kim, K. Viswanathan, G. Kasi, S. Thanakkasaranee, K. Sadeghi and J. Seo, ZnO nanostructures in active antibacterial food packaging: Preparation methods, antimicrobial mechanisms, safety issues, future prospects, and challenges, Food Rev. Int., 2022, 38, 537–565 CrossRef CAS.
- L. Frechette-Viens, M. Hadioui and K. J. Wilkinson, Quantification of ZnO nanoparticles and other Zn containing colloids in natural waters using a high sensitivity single particle ICP-MS, Talanta, 2019, 200, 156–162 CrossRef CAS PubMed.
- S. Choi, M. Johnston, G. S. Wang and C. P. Huang, A seasonal observation on the distribution of engineered nanoparticles in municipal wastewater treatment systems exemplified by TiO2 and ZnO, Sci. Total Environ., 2018, 625, 1321–1329 CrossRef CAS PubMed.
- X. Zhang, K. Yin, R. Huo, Z. Wang, S. Fan, Q. Ma, L. Wang, S. Zhai and J. Wang, Phytotoxic effects of different concentrations of zinc species on lettuce, Water, Air, Soil Pollut., 2023, 234, 569 CrossRef CAS.
- M. Nekoukhou, S. Fallah, A. Abbasi-Surki, L. R. Pokhrel and A. Rostamnejadi, Improved efficacy of foliar application of zinc oxide nanoparticles on zinc biofortification, primary productivity and secondary metabolite production in dragonhead, J. Cleaner Prod., 2022, 379, 134803 CrossRef CAS.
- J. Guo, S. Li, M. Brestic, N. Li, P. Zhang, L. Liu and X. Li, Modulations in protein phosphorylation explain the physiological responses of barley (Hordeum vulgare) to nanoplastics and ZnO nanoparticles, J. Hazard. Mater., 2023, 443, 130196 CrossRef CAS PubMed.
- S. Banerjee, J. Islam, S. Mondal, A. Saha, B. Saha and A. Sen, Proactive attenuation of arsenic-stress by nano-priming: Zinc oxide nanoparticles in Vigna mungo (L.) Hepper trigger antioxidant defense response and reduce root-shoot arsenic translocation, J. Hazard. Mater., 2023, 446, 130735 CrossRef CAS.
- Y. Liu, M. Kang, Y. Weng, Y. Ding and X. Bai, Toxicity and tolerance mechanism of binary zinc oxide nanoparticles and tetrabromobisphenol A regulated by humic acid in Chlorella vulgaris, Environ. Sci.: Processes Impacts, 2023, 25, 1615–1625 RSC.
- C. M. Rico, S. Majumdar, M. Duarte-Gardea, J. R. Peralta-Videa and J. L. Gardea-Torresdey, Interaction of nanoparticles with edible plants and their possible implications in the food chain, J. Agric. Food Chem., 2011, 59, 3485–3498 CrossRef CAS PubMed.
- V. Rajput, T. Minkina, M. Mazarji, S. Shende, S. Sushkova, S. Mandzhieva, M. Burachevskaya, V. Chaplygin, A. Singh and H. Jatav, Accumulation of nanoparticles in the soil-plant systems and their effects on human health, Ann. Agric. Sci., 2020, 65, 137–143 CrossRef.
- P. Miralles, T. L. Church and A. T. Harris, Toxicity, uptake, and translocation of engineered nanomaterials in vascular plants, Environ. Sci. Technol., 2012, 46, 9224–9239 CrossRef CAS PubMed.
- M. R. Khan, V. Adam, T. F. Rizvi, B. Zhang, F. Ahamad, I. Josko, Y. Zhu, M. Yang and C. Mao, Nanoparticle-plant interactions: Two-way traffic, Small, 2019, 15, e1901794 CrossRef PubMed.
- C. Caldelas, F. Poitrasson, J. Viers and J. L. Araus, Stable Zn isotopes reveal the uptake and toxicity of zinc oxide engineered nanomaterials in Phragmites australis, Environ. Sci.: Nano, 2020, 7, 1927–1941 RSC.
- P. Zoufan, M. Baroonian and B. Zargar, ZnO nanoparticles-induced oxidative stress in Chenopodium murale L, Zn uptake, and accumulation under hydroponic culture, Environ. Sci. Pollut. Res., 2020, 27, 11066–11078 CrossRef CAS PubMed.
- F. Ghouri, M. J. Shahid, J. Liu, M. Lai, L. Sun, J. Wu, X. Liu, S. Ali and M. Q. Shahid, Polyploidy and zinc oxide nanoparticles alleviated Cd toxicity in rice by modulating oxidative stress and expression levels of sucrose and metal-transporter genes, J. Hazard. Mater., 2023, 448, 130991 CrossRef CAS PubMed.
- J. A. Yin, G. H. Huang, C. J. An and R. F. Feng, Nanocellulose enhances the dispersion and toxicity of ZnO NPs to green algae Eremosphaera viridis, Environ. Sci.: Nano, 2022, 9, 393–405 RSC.
- Y. M. Su, V. Ashworth, C. Kim, A. S. Adeleye, P. Rolshausen, C. Roper, J. White and D. Jassby, Delivery, uptake, fate, and transport of engineered nanoparticles in plants: A critical review and data analysis, Environ. Sci.: Nano, 2019, 6, 2311–2331 RSC.
- S. Afzal, T. Aftab and N. K. Singh, Impact of zinc oxide and iron oxide nanoparticles on uptake, translocation, and physiological effects in Oryza sativa L, J. Plant Growth Regul., 2022, 41, 1445–1461 CrossRef CAS.
- R. Amooaghaie, M. Norouzi and M. Saeri, Impact of zinc and zinc oxide nanoparticles on the physiological and biochemical processes in tomato and wheat, Botany, 2017, 95, 441–455 CrossRef CAS.
- J. H. Zhu, J. F. Li, Y. Shen, S. Q. Liu, N. D. Zeng, X. H. Zhan, J. C. White, J. Gardea-Torresdey and B. S. Xing, Mechanism of zinc oxide nanoparticle entry into wheat seedling leaves, Environ. Sci.: Nano, 2020, 7, 3901–3913 RSC.
- J. Zhu, J. Wang, X. Zhan, A. Li, J. C. White, J. L. Gardea-Torresdey and B. Xing, Role of charge and size in the translocation and distribution of zinc oxide particles in wheat cells, ACS Sustainable Chem. Eng., 2021, 9, 11556–11564 CrossRef CAS.
- T. L. Read, C. L. Doolette, N. R. Howell, P. M. Kopittke, T. Cresswell and E. Lombi, Zinc accumulates in the nodes of wheat following the foliar application of 65Zn oxide nano- and microparticles, Environ. Sci. Technol., 2021, 55, 13523–13531 CrossRef CAS PubMed.
- C. L. Doolette, T. L. Read, N. R. Howell, T. Cresswell and E. Lombi, Zinc from foliar-applied nanoparticle fertiliser is translocated to wheat grain: A 65Zn radiolabelled translocation study comparing conventional and novel foliar fertilisers, Sci. Total Environ., 2020, 749, 142369 CrossRef CAS.
- L. L. Sun, Y. B. Wang, R. L. Wang, R. T. Wang, P. Zhang, Q. Ju and J. Xu, Physiological, transcriptomic, and metabolomic analyses reveal zinc oxide nanoparticles modulate plant growth in tomato, Environ. Sci.: Nano, 2020, 7, 3587–3604 RSC.
- S. Adhikari, A. Adhikari, S. Ghosh, D. Roy, I. Azahar, D. Basuli and Z. Hossain, Assessment of ZnO-NPs toxicity in maize: An integrative microRNAomic approach, Chemosphere, 2020, 249, 126197 CrossRef CAS PubMed.
- J. T. Lv, S. Z. Zhang, L. Luo, J. Zhang, K. Yang and P. Christie, Accumulation, speciation and uptake pathway of ZnO nanoparticles in maize, Environ. Sci.: Nano, 2015, 2, 68–77 RSC.
- Z. J. Zhu, H. Wang, B. Yan, H. Zheng, Y. Jiang, O. R. Miranda, V. M. Rotello, B. Xing and R. W. Vachet, Effect of surface charge on the uptake and distribution of gold nanoparticles in four plant species, Environ. Sci. Technol., 2012, 46, 12391–12398 CrossRef CAS.
- R. Tenhaken, Cell wall remodeling under abiotic stress, Front. Plant Sci., 2014, 5, 771 Search PubMed.
- E. Navarro, A. Baun, R. Behra, N. B. Hartmann, J. Filser, A. J. Miao, A. Quigg, P. H. Santschi and L. Sigg, Environmental behavior and ecotoxicity of engineered nanoparticles to algae, plants, and fungi, Ecotoxicology, 2008, 17, 372–386 CrossRef CAS PubMed.
- Z. Lv, H. Sun, W. Du, R. Li, H. Mao and P. M. Kopittke, Interaction of different-sized ZnO nanoparticles with maize (Zea mays): Accumulation, biotransformation and phytotoxicity, Sci. Total Environ., 2021, 796, 148927 CrossRef CAS.
- J. Chen, R. Dou, Z. Yang, T. You, X. Gao and L. Wang, Phytotoxicity and bioaccumulation of zinc oxide nanoparticles in rice (Oryza sativa L.), Plant Physiol. Biochem., 2018, 130, 604–612 CrossRef CAS.
- L. Zhao, J. R. Peralta-Videa, M. Ren, A. Varela-Ramirez, C. Li, J. A. Hernandez-Viezcas, R. J. Aguilera and J. L. Gardea-Torresdey, Transport of Zn in a sandy loam soil treated with ZnO NPs and uptake by corn plants: Electron microprobe and confocal microscopy studies, Chem. Eng. J., 2012, 184, 1–8 CrossRef CAS.
- J. Wan, R. Wang, R. Wang, Q. Ju, Y. Wang and J. Xu, Comparative physiological and transcriptomic analyses reveal the toxic effects of ZnO nanoparticles on plant growth, Environ. Sci. Technol., 2019, 53, 4235–4244 CrossRef CAS PubMed.
- L. A. N. Claus, D. V. Savatin and E. Russinova, The crossroads of receptor-mediated signaling and endocytosis in plants, J. Integr. Plant Biol., 2018, 60, 827–840 CrossRef PubMed.
- A. Dev, A. K. Srivastava and S. Karmakar, Nanomaterial toxicity for plants, Environ. Chem. Lett., 2018, 16, 85–100 CrossRef CAS.
- B. Ahmed, S. Dwivedi, M. Z. Abdin, A. Azam, M. Al-Shaeri, M. S. Khan, Q. Saquib, A. A. Al-Khedhairy and J. Musarrat, Mitochondrial and chromosomal damage induced by oxidative stress in Zn2+ ions, ZnO-Bulk and ZnO-NPs treated Allium cepa roots, Sci. Rep., 2017, 7, 40685 CrossRef CAS PubMed.
- T. N. M. da Cruz, S. M. Savassa, G. S. Montanha, J. K. Ishida, E. de Almeida, S. M. Tsai, J. Lavres Junior and H. W. Pereira de Carvalho, A new glance on root-to-shoot in vivo zinc transport and time-dependent physiological effects of ZnSO4 and ZnO nanoparticles on plants, Sci. Rep., 2019, 9, 10416 CrossRef.
- A. Avellan, J. Yun, B. P. Morais, E. T. Clement, S. M. Rodrigues and G. V. Lowry, Critical review: Role of inorganic nanoparticle properties on their foliar uptake and in Planta translocation, Environ. Sci. Technol., 2021, 55, 13417–13431 CrossRef CAS.
- E. A. Ibrahim, Seed priming to alleviate salinity stress in germinating seeds, J. Plant Physiol., 2016, 192, 38–46 CrossRef CAS PubMed.
- Z. G. Dogaroglu, A. Eren and M. F. Baran, Effects of ZnO nanoparticles and ethylenediamine-N,N'-disuccinic acid on seed germination of four different plants, Global chall., 2019, 3, 1800111 CrossRef.
- M. L. López-Moreno, G. de la Rosa, G. Cruz-Jiménez, L. Castellano, J. R. Peralta-Videa and J. L. Gardea-Torresdey, Effect of ZnO nanoparticles on corn seedlings at different temperatures; X-ray absorption spectroscopy and ICP/OES studies, Microchem. J., 2017, 134, 54–61 CrossRef.
- A. R. Gomes, L. P. de Matos, A. T. B. Guimarães, Í. N. Freitas, T. M. D. Luz, A. M. Silva, S. G. D. Silva Matos, A. S. D. L. Rodrigues, R. D. O. Ferreira, A. R. M. T. Islam, M. M. Rahman, C. Ragavendran, C. Kamaraj, N. M. Mubarak, A. H. Arias, P. C. S. Gomes, F. G. Silva and G. Malafaia, Plant-ZnO nanoparticles interaction: An approach to improve guinea grass (Panicum maximum) productivity and evaluation of the impacts of its ingestion by freshwater teleost fish, J. Hazard. Mater., 2023, 451, 131173 CrossRef CAS.
- H. Xun, X. Ma, J. Chen, Z. Yang, B. Liu, X. Gao, G. Li, J. Yu, L. Wang and J. Pang, Zinc oxide nanoparticle exposure triggers different gene expression patterns in maize shoots and roots, Environ. Pollut., 2017, 229, 479–488 CrossRef CAS.
- D. Lin and B. Xing, Root uptake and phytotoxicity of ZnO nanoparticles, Environ. Sci. Technol., 2008, 42, 5580–5585 CrossRef CAS PubMed.
- M. Kumari, S. S. Khan, S. Pakrashi, A. Mukherjee and N. Chandrasekaran, Cytogenetic and genotoxic effects of zinc oxide nanoparticles on root cells of Allium cepa, J. Hazard. Mater., 2011, 190, 613–621 CrossRef CAS PubMed.
- B. V. C. Oscar, S. P. Melegari, D. S. Vicentini, C. Simioni, L. C. Ouriques, R. C. Puerari and W. G. Matias, Toxicological effects of pure and amine-functionalized ZnO nanorods on Daphnia magna and Lactuca sativa, Environ. Sci.: Nano, 2023, 10, 1190–1207 RSC.
- S. Lee, S. Kim, S. Kim and I. Lee, Assessment of phytotoxicity of ZnO NPs on a medicinal plant, Fagopyrum esculentum, Environ. Sci. Pollut. Res., 2013, 20, 848–854 CrossRef CAS PubMed.
- J. H. Priester, S. C. Moritz, K. Espinosa, Y. Ge, Y. Wang, R. M. Nisbet, J. P. Schimel, A. Susana Goggi, J. L. Gardea-Torresdey and P. A. Holden, Damage assessment for soybean cultivated in soil with either CeO2 or ZnO manufactured nanomaterials, Sci. Total Environ., 2017, 579, 1756–1768 CrossRef CAS.
- X. Wang, X. Yang, S. Chen, Q. Li, W. Wang, C. Hou, X. Gao, L. Wang and S. Wang, Zinc oxide nanoparticles affect biomass accumulation and photosynthesis in Arabidopsis, Front. Plant Sci., 2016, 6, 1243 Search PubMed.
- M. Samei, M. H. Sarrafzadeh and M. A. Faramarzi, The impact of morphology and size of zinc oxide nanoparticles on its toxicity to the freshwater microalga, Raphidocelis subcapitata, Environ. Sci. Pollut. Res., 2019, 26, 2409–2420 CrossRef CAS PubMed.
- A. Vicente, B. Sohm, J. Flayac, P. Rousselle, P. Bauda and C. Pagnout, Toxicity mechanisms of ZnO UV-filters used in sunscreens toward the model cyanobacteria Synechococcus elongatus PCC 7942, Environ. Sci. Pollut. Res., 2019, 26, 22450–22463 CrossRef CAS PubMed.
- L. Li, K. H. Liu and J. Sheen, Dynamic nutrient signaling networks in plants, Annu. Rev. Cell Dev. Biol., 2021, 37, 341–367 CrossRef CAS.
- L. Balazova, P. Babula, M. Balaz, M. Backorova, Z. Bujnakova, J. Briancin, A. Kurmanbayeva and M. Sagi, Zinc oxide nanoparticles phytotoxicity on halophyte from genus Salicornia, Plant Physiol. Biochem., 2018, 130, 30–42 CrossRef CAS PubMed.
- C. Zou, T. Lu, R. Wang, P. Xu, Y. Jing, R. Wang, J. Xu and J. Wan, Comparative physiological and metabolomic analyses reveal that Fe3O4 and ZnO nanoparticles alleviate Cd toxicity in tobacco, J. Nanobiotechnol., 2022, 20, 302 CrossRef CAS PubMed.
- F. A. Farghaly, A. A. Radi, F. A. Al-Kahtany and A. M. Hamada, Impacts of zinc oxide nano and bulk particles on redox-enzymes of the Punica granatum callus, Sci. Rep., 2020, 10, 19722 CrossRef CAS.
- M. Shen, W. Liu, A. Zeb, J. Lian, J. Wu and M. Lin, Bioaccumulation and phytotoxicity of ZnO nanoparticles in soil-grown Brassica chinensis L. and potential risks, J. Environ. Manage., 2022, 306, 114454 CrossRef CAS.
- B. Ahmed, A. Rizvi, A. Syed, A. M. Elgorban, M. S. Khan, H. A. Al-Shwaiman, J. Musarrat and J. Lee, Differential responses of maize (Zea mays) at the physiological, biomolecular, and nutrient levels when cultivated in the presence of nano or bulk ZnO or CuO or Zn2+ or Cu2+ ions, J. Hazard. Mater., 2021, 419, 126493 CrossRef CAS PubMed.
- A. R. Khan, A. Wakeel, N. Muhammad, B. Liu, M. Wu, Y. Liu, I. Ali, S. H. R. Zaidi, W. Azhar, G. Song, J. Wu and Y. Gan, Involvement of ethylene signaling in zinc oxide nanoparticle-mediated biochemical changes in Arabidopsis thaliana leaves, Environ. Sci.: Nano, 2019, 6, 341–355 RSC.
- S. Jahan, Y. B. Alias, A. Bakar and I. B. Yusoff, Toxicity evaluation of ZnO and TiO2 nanomaterials in hydroponic red bean (Vigna angularis) plant: Physiology, biochemistry and kinetic transport, J. Environ. Sci., 2018, 72, 140–152 CrossRef CAS PubMed.
- H. Salehi, N. De Diego, A. Chehregani Rad, J. J. Benjamin, M. Trevisan and L. Lucini, Exogenous application of ZnO nanoparticles and ZnSO4 distinctly influence the metabolic response in Phaseolus vulgaris L, Sci. Total Environ., 2021, 778, 146331 CrossRef CAS PubMed.
- L. Zhao, Y. Sun, J. A. Hernandez-Viezcas, J. Hong, S. Majumdar, G. Niu, M. Duarte-Gardea, J. R. Peralta-Videa and J. L. Gardea-Torresdey, Monitoring the environmental effects of CeO2 and ZnO nanoparticles through the life cycle of corn (Zea mays) plants and in situ mu-XRF mapping of nutrients in kernels, Environ. Sci. Technol., 2015, 49, 2921–2928 CrossRef CAS.
- X. P. Wang, Q. Q. Li, Z. M. Pei and S. C. Wang, Effects of zinc oxide nanoparticles on the growth, photosynthetic traits, and antioxidative enzymes in tomato plants, Biol. Plant., 2018, 62, 801–808 CrossRef CAS.
- H. Zhang, Z. Chen and Q. Huang, Study of the toxicity of ZnO nanoparticles to Chlorella sorokiniana under the influence of phosphate: Spectroscopic quantification, photosynthetic efficiency and gene expression analysis, Environ. Sci.: Nano, 2020, 7, 1431–1443 RSC.
- K. Azarin, A. Usatov, T. Minkina, A. Plotnikov, A. Kasyanova, A. Fedorenko, N. Duplii, E. Vechkanov, V. D. Rajput, S. Mandzhieva and S. Alamri, Effects of ZnO nanoparticles and its bulk form on growth, antioxidant defense system and expression of oxidative stress related genes in Hordeum vulgare L, Chemosphere, 2022, 287, 132167 CrossRef CAS PubMed.
- Z. Zhang, Q. Cui, L. Chen, X. Zhu, S. Zhao, C. Duan, X. Zhang, D. Song and L. Fang, A critical review of microplastics in the soil-plant system: Distribution, uptake, phytotoxicity and prevention, J. Hazard. Mater., 2022, 424, 127750 CrossRef CAS PubMed.
- A. I. Daniel, M. Keyster and A. Klein, Biogenic zinc oxide nanoparticles: A viable agricultural tool to control plant pathogenic fungi and its potential effects on soil and plants, Sci. Total Environ., 2023, 897, 165483 CrossRef CAS PubMed.
- K. Włodarczyk, B. Smolińska and I. Majak, The antioxidant potential of tomato plants (Solanum lycopersicum L.) under nano-ZnO treatment, Int. J. Mol. Sci., 2023, 24, 11833 CrossRef PubMed.
- B. Ahmed, A. Rizvi, K. Ali, J. Lee, A. Zaidi, M. S. Khan and J. Musarrat, Nanoparticles in the soil–plant system: A review, Environ. Chem. Lett., 2021, 19, 1545–1609 CrossRef CAS.
- H. Zhang, W. Du, J. R. Peralta-Videa, J. L. Gardea-Torresdey, J. C. White, A. Keller, H. Guo, R. Ji and L. Zhao, Metabolomics reveals how cucumber (Cucumis sativus) reprograms metabolites to cope with silver ions and silver nanoparticle-induced oxidative stress, Environ. Sci. Technol., 2018, 52, 8016–8026 CrossRef CAS PubMed.
- R. Mittler, S. I. Zandalinas, Y. Fichman and F. Van Breusegem, Reactive oxygen species signalling in plant stress responses, Nat. Rev. Mol. Cell Biol., 2022, 23, 663–679 CrossRef CAS PubMed.
- Y. Song, B. Q. Wang, D. Y. Qiu, Z. M. Xie, S. Dai, C. Li, S. L. Xu, Y. C. Zheng, S. Li and M. Jiang, Melatonin enhances metallic oxide nanoparticle stress tolerance in rice via inducing tetrapyrrole biosynthesis and amino acid metabolism, Environ. Sci.: Nano, 2021, 8, 2310–2323 RSC.
- H. Tao, S. Hu, C. Xia, M. Wang, T. Wang, W. Zeng, Y. Li, H. Chen, J. Zheng and Q. Wang, Involvement of glucosinolates in the resistance to zinc oxide nanoparticle-induced toxicity and growth inhibition in Arabidopsis, Environ. Sci.: Processes Impacts, 2021, 23, 1040–1049 RSC.
- M. A. Akanbi-Gada, C. O. Ogunkunle, V. Vishwakarma, K. Viswanathan and P. O. Fatoba, Phytotoxicity of nano-zinc oxide to tomato plant (Solanum lycopersicum L.): Zn uptake, stress enzymes response and influence on non-enzymatic antioxidants in fruits, Environ. Technol. Innovation, 2019, 14, 100325 CrossRef.
- S. Li, J. Liu, Y. Wang, Y. Gao, Z. Zhang, J. Xu and G. Xing, Comparative physiological and metabolomic analyses revealed that foliar spraying with zinc oxide and silica nanoparticles modulates metabolite profiles in cucumber (Cucumis sativus L.), Food Energy Secur., 2021, 10, e269 CrossRef CAS.
- A. R. Khan, W. Azhar, J. Wu, Z. Ulhassan, A. Salam, S. H. R. Zaidi, S. Yang, G. Song and Y. Gan, Ethylene participates in zinc oxide nanoparticles induced biochemical, molecular and ultrastructural changes in rice seedlings, Ecotoxicol. Environ. Saf., 2021, 226, 112844 CrossRef CAS PubMed.
- X. Li, Z. Ban, F. Yu, W. Hao and X. Hu, Untargeted metabolic pathway analysis as an effective strategy to connect various nanoparticle properties to nanoparticle-induced ecotoxicity, Environ. Sci. Technol., 2020, 54, 3395–3406 CrossRef CAS PubMed.
- J. Wan, R. Wang, H. Bai, Y. Wang and J. Xu, Comparative physiological and metabolomics analysis reveals that single-walled carbon nanohorns and ZnO nanoparticles affect salt tolerance in Sophora alopecuroides, Environ. Sci.: Nano, 2020, 7, 2968–2981 RSC.
- S. Guo, X. Zhang and H. Sun, Transcriptomic mechanism for foliar applied nano-ZnO alleviating phytotoxicity of nanoplastics in corn (Zea mays L.) plants, Sci. Total Environ., 2023, 905, 166818 CrossRef CAS PubMed.
- V. A. Kostyuk, A. I. Potapovich, E. N. Strigunova, T. V. Kostyuk and I. B. Afanas'ev, Experimental evidence that flavonoid metal complexes may act as mimics of superoxide dismutase, Arch. Biochem. Biophys., 2004, 428, 204–208 CrossRef CAS PubMed.
- A. S. Shaban, M. E. Owda, M. M. Basuoni, M. A. Mousa, A. A. Radwan and A. K. Saleh,
Punica granatum peel extract mediated green synthesis of zinc oxide nanoparticles: Structure and evaluation of their biological applications, Biomass Convers. Biorefin., 2022 DOI:10.1007/s13399-022-03185-7.
- P. Landa, S. Prerostova, S. Petrova, V. Knirsch, R. Vankova and T. Vanek, The transcriptomic response of Arabidopsis thaliana to zinc oxide: A comparison of the impact of nanoparticle, bulk, and ionic zinc, Environ. Sci. Technol., 2015, 49, 14537–14545 CrossRef CAS PubMed.
- E. DemİR, N. Kaya and B. Kaya, Genotoxic effects of zinc oxide and titanium dioxide nanoparticles on root meristem cells of Allium cepa by comet assay, Turk. J. Biol., 2014, 38, 31–39 CrossRef.
- M. L. Lopez-Moreno, G. de la Rosa, J. A. Hernandez-Viezcas, H. Castillo-Michel, C. E. Botez, J. R. Peralta-Videa and J. L. Gardea-Torresdey, Evidence of the differential biotransformation and genotoxicity of ZnO and CeO2 nanoparticles on soybean (Glycine max) plants, Environ. Sci. Technol., 2010, 44, 7315–7320 CrossRef CAS PubMed.
- K. Haliloglu, A. Turkoglu, O. Balpinar, H. Nadaroglu, A. Alayli and P. Poczai, Effects of zinc, copper and iron oxide nanoparticles on induced DNA methylation, genomic instability and LTR retrotransposon polymorphism in wheat (Triticum aestivum L.), Plants, 2022, 11, 2193 CrossRef CAS PubMed.
- X. Li, W. Ding, S. Tan and X. Zeng, Stability of Nano-ZnO in simulated landfill leachate containing heavy metal ions, Ecotoxicol. Environ. Saf., 2020, 198, 110641 CrossRef CAS PubMed.
- L. Pagano, F. Pasquali, S. Majumdar, R. De la Torre-Roche, N. Zuverza-Mena, M. Villani, A. Zappettini, R. E. Marra, S. M. Isch, M. Marmiroli, E. Maestri, O. P. Dhankher, J. C. White and N. Marmiroli, Exposure of Cucurbita pepo to binary combinations of engineered nanomaterials: Physiological and molecular response, Environ. Sci.: Nano, 2017, 4, 1579–1590 RSC.
- M. A. Gomez-Gonzalez, T. Da Silva-Ferreira, N. Clark, R. Clough, P. D. Quinn and J. E. Parker, Toward understanding the environmental risks of combined microplastics/nanomaterials exposures: Unveiling ZnO transformations after adsorption onto polystyrene microplastics in environmental solutions, Global chall., 2023, 7, 2300036 CrossRef PubMed.
- U. Rozman, B. Klun, G. Marolt, J. Imperl and G. Kalčíková, A study of the adsorption of titanium dioxide and zinc oxide nanoparticles on polyethylene microplastics and their desorption in aquatic media, Sci. Total Environ., 2023, 888, 164163 CrossRef CAS PubMed.
- A. Sun and W.-X. Wang, Photodegradation of microplastics by ZnO nanoparticles with resulting cellular and subcellular responses, Environ. Sci. Technol., 2023, 57, 8118–8129 CrossRef CAS PubMed.
- X. Ma, H. Sharifan, F. Dou and W. Sun, Simultaneous reduction of arsenic (As) and cadmium (Cd) accumulation in rice by zinc oxide nanoparticles, Chem. Eng. J., 2020, 384, 123802 CrossRef CAS.
- P. Venkatachalam, M. Jayaraj, R. Manikandan, N. Geetha, E. R. Rene, N. C. Sharma and S. V. Sahi, Zinc oxide nanoparticles (ZnONPs) alleviate heavy metal-induced toxicity in Leucaena leucocephala seedlings: A physiochemical analysis, Plant Physiol. Biochem., 2017, 110, 59–69 CrossRef CAS PubMed.
- R. T. Wang, L. L. Sun, P. Zhang, J. P. Wan, Y. B. Wang and J. Xu, Zinc oxide nanoparticles alleviate cadmium stress by modulating plant metabolism and decreasing cadmium accumulation in Perilla frutescents, Plant Growth Regul., 2023, 100, 85–96 CrossRef CAS.
- F. Chen, Y. Li, M. Zia-Ur-Rehman, S. M. Hussain, M. F. Qayyum, M. Rizwan, H. F. Alharby, N. M. Alabdallah, B. M. Alharbi and S. Ali, Combined effects of zinc oxide nanoparticles and melatonin on wheat growth, chlorophyll contents, cadmium (Cd) and zinc uptake under Cd stress, Sci. Total Environ., 2023, 864, 161061 CrossRef CAS PubMed.
- L. Sun, R. Wang, Q. Ju, M. Xing, R. Li, W. Li, W. Li, W. Wang, Y. Deng and J. Xu, Mitigation mechanism of zinc oxide nanoparticles on cadmium toxicity in tomato, Front. Plant Sci., 2023, 14, 1162372 CrossRef PubMed.
- H. Sharifan, X. M. Ma, J. M. Moore, M. R. Habib and C. Eyans, Zinc oxide nanoparticles alleviated the bioavailability of cadmium and lead and changed the uptake of iron in hydroponically grown lettuce (Lactuca sativa L. var. Longifolia), ACS Sustainable Chem. Eng., 2019, 7, 16401–16409 CrossRef CAS.
- A. Salam, A. R. Khan, L. Liu, S. Yang, W. Azhar, Z. Ulhassan, M. Zeeshan, J. Wu, X. Fan and Y. Gan, Seed priming with zinc oxide nanoparticles downplayed ultrastructural damage and improved photosynthetic apparatus in maize under cobalt stress, J. Hazard. Mater., 2022, 423, 127021 CrossRef CAS PubMed.
- V. Prakash, P. Rai, N. C. Sharma, V. P. Singh, D. K. Tripathi, S. Sharma and S. Sahi, Application of zinc oxide nanoparticles as fertilizer boosts growth in rice plant and alleviates chromium stress by regulating genes involved in oxidative stress, Chemosphere, 2022, 303, 134554 CrossRef CAS PubMed.
- A. Jalal, C. Oliveira, A. C. Bastos, G. C. Fernandes, B. H. de Lima, E. Furlani Junior, P. H. G. de Carvalho, F. S. Galindo, I. M. B. Gato and M. C. M. Teixeira Filho, Nanozinc and plant growth-promoting bacteria improve biochemical and metabolic attributes of maize in tropical Cerrado, Front. Plant Sci., 2022, 13, 1046642 CrossRef PubMed.
- M. Li, G. J. Ahammed, C. Li, X. Bao, J. Yu, C. Huang, H. Yin and J. Zhou, Brassinosteroid ameliorates zinc oxide nanoparticles-induced oxidative stress by improving antioxidant potential and redox homeostasis in tomato seedling, Front. Plant Sci., 2016, 7, 615 Search PubMed.
- H. A. Kareem, M. U. Hassan, M. Zain, A. Irshad, N. Shakoor, S. Saleem, J. Niu, M. Skalicky, Z. Chen, Z. Guo and Q. Wang, Nanosized zinc oxide (n-ZnO) particles pretreatment to alfalfa seedlings alleviate heat-induced morpho-physiological and ultrastructural damages, Environ. Pollut., 2022, 303, 119069 CrossRef CAS PubMed.
- M. I. Ghani, S. Saleem, S. A. Rather, M. S. Rehmani, S. Alamri, V. D. Rajput, H. M. Kalaji, N. Saleem, T. A. Sial and M. Liu, Foliar application of zinc oxide nanoparticles: An effective strategy to mitigate drought stress in cucumber seedling by modulating antioxidant defense system and osmolytes accumulation, Chemosphere, 2022, 289, 133202 CrossRef CAS PubMed.
- N. I. Elsheery, V. S. J. Sunoj, Y. Wen, J. J. Zhu, G. Muralidharan and K. F. Cao, Foliar application of nanoparticles mitigates the chilling effect on photosynthesis and photoprotection in sugarcane, Plant Physiol. Biochem., 2020, 149, 50–60 CrossRef CAS PubMed.
- M. Hong, J.-L. Gong, W.-C. Cao, R. Fang, Z. Cai, J. Ye, Z.-P. Chen and W.-W. Tang, The combined toxicity and mechanism of multi-walled carbon nanotubes and nano zinc oxide toward the cabbage, Environ. Sci. Pollut. Res., 2022, 29, 3540–3554 CrossRef CAS PubMed.
- L. Hazeem, Single and combined toxicity effects of zinc oxide nanoparticles: uptake and accumulation in marine microalgae, toxicity mechanisms, and their fate in the marine environment, Water, 2022, 14, 2669 CrossRef CAS.
- C. García-Gómez, R. A. Pérez, B. Albero, A. Obrador, P. Almendros and M. D. Fernández, Interaction of ZnO nanoparticles with metribuzin in a soil-plant system: Ecotoxicological effects and changes in the distribution pattern of Zn and metribuzin, Agronomy, 2023, 13, 2004 CrossRef.
- W. W. Yang, P. Cheng, C. A. Adams, S. W. Zhang, Y. H. Sun, H. W. Yu and F. Y. Wang, Effects of microplastics on plant growth and arbuscular mycorrhizal fungal communities in a soil spiked with ZnO nanoparticles, Soil Biol. Biochem., 2021, 155, 108179 CrossRef CAS.
- Y. Xiao, Y. Li, Y. Shi, Z. Li, X. Zhang, T. Liu, T. H. Farooq, Y. Pan, X. Chen and W. Yan, Combined toxicity of zinc oxide nanoparticles and cadmium inducing root damage in Phytolacca americana L, Sci. Total Environ., 2022, 806, 151211 CrossRef CAS PubMed.
- S. Yang, R. Yin, C. Wang, Y. Yang and J. Wang, Phytotoxicity of zinc oxide nanoparticles and multi-walled carbon nanotubes, alone or in combination, on Arabidopsis thaliana and their mutual effects on oxidative homeostasis, PLoS One, 2023, 18, e0281756 CrossRef CAS PubMed.
- J. Guo, N. Liu, Q. Xie, L. Zhu and F. Ge, Polystyrene microplastics facilitate the biotoxicity and biomagnification of ZnO nanoparticles in the food chain from algae to daphnia, Environ. Pollut., 2023, 324, 121181 CrossRef CAS PubMed.
- Y. Meng, S. Wang, Z. Wang, N. Ye and H. Fang, Algal toxicity of binary mixtures of zinc oxide nanoparticles and tetrabromobisphenol A: Roles of dissolved organic matters, Environ. Toxicol. Pharmacol., 2018, 64, 78–85 CrossRef CAS PubMed.
- M. Ramzan, F. Ayub, A. A. Shah, G. Naz, A. N. Shah, A. Malik, R. Sardar, A. Telesinski, H. M. Kalaji, E. S. Dessoky and H. A. Elgawad, Synergistic effect of zinc oxide nanoparticles and Moringa oleifera leaf extract alleviates cadmium toxicity in Linum usitatissimum: Antioxidants and physiochemical studies, Front. Plant Sci., 2022, 13, 900347 CrossRef PubMed.
- Y. Li, L. Liang, W. Li, U. Ashraf, L. Ma, X. Tang, S. Pan, H. Tian and Z. Mo, ZnO nanoparticle-based seed priming modulates early growth and enhances physio-biochemical and metabolic profiles of fragrant rice against cadmium toxicity, J. Nanobiotechnol., 2021, 19, 75 CrossRef CAS PubMed.
- H. Sharifan, J. Moore and X. Ma, Zinc oxide (ZnO) nanoparticles elevated iron and copper contents and mitigated the bioavailability of lead and cadmium in different leafy greens, Ecotoxicol. Environ. Saf., 2020, 191, 110177 CrossRef CAS PubMed.
- E. Skiba, M. Pietrzak, S. Glinska and W. M. Wolf, The combined effect of ZnO and CeO2 nanoparticles on Pisum sativum L.: A photosynthesis and nutrients uptake study, Cells, 2021, 10, 3105 CrossRef CAS PubMed.
- A. Iftikhar, S. Ali, T. Yasmeen, M. S. Arif, M. Zubair, M. Rizwan, H. A. S. Alhaithloul, A. A. M. Alayafi and M. H. Soliman, Effect of gibberellic acid on growth, photosynthesis and antioxidant defense system of wheat under zinc oxide nanoparticle stress, Environ. Pollut., 2019, 254, 113109 CrossRef CAS PubMed.
- S. Taherbahrani, P. Zoufan and B. Zargar, Modulation of the toxic effects of zinc oxide nanoparticles by exogenous salicylic acid pretreatment in Chenopodium murale L, Environ. Sci. Pollut. Res., 2021, 28, 65644–65654 CrossRef CAS PubMed.
- S. S. Ali, R. Al-Tohamy, E. Koutra, M. S. Moawad, M. Kornaros, A. M. Mustafa, Y. A. Mahmoud, A. Badr, M. E. H. Osman, T. Elsamahy, H. Jiao and J. Sun, Nanobiotechnological advancements in agriculture and food industry: Applications, nanotoxicity, and future perspectives, Sci. Total Environ., 2021, 792, 148359 CrossRef CAS PubMed.
|
This journal is © The Royal Society of Chemistry 2024 |