Speeding it up: dual effects of biostimulants and iron on the biodegradation of poly(lactic acid) at mesophilic conditions†
Received
30th November 2023
, Accepted 2nd February 2024
First published on 2nd February 2024
Abstract
Plastic pollution presents a growing concern, and various solutions have been proposed to address it. One such solution involves the development of new plastics that match the properties of traditional polymers while exhibiting enhanced biodegradability when disposed of in a suitable environment. Poly(lactic acid) (PLA) is a biobased, compostable polymer known for its low environmental impact and ability to break down into harmless components within a specified timeframe. However, its degradation in industrial composting facilities poses challenges, and it cannot degrade in home composting. In this study, we investigated the biodegradability of PLA within a biostimulated compost matrix at mesophilic conditions (37 °C) over 180 days. The compost environment was enhanced with Fe3O4 nanopowder, skim milk, gelatin, and ethyl lactate, individually and in combination, to target different stages of the PLA biodegradation process. We monitored key indicators, CO2 evolution, number average molecular weight, and crystallinity, to assess the impact of the various biostimulants and iron. The results demonstrated that the most effective treatment for degrading PLA at mesophilic conditions was adding gelatin and Fe3O4. Gelatin accelerated PLA biodegradation by 25%, Fe3O4 by 17%, and a combination of gelatin and Fe3O4 by 30%. The effect of skim milk and ethyl lactate is also reported. This research introduces novel pathways to enhance PLA biodegradation in home composting scenarios, offering promising solutions to address the plastic pollution challenge.
Environmental significance
Plastics are omnipresent in daily life, particularly in food packaging applications, and are often released into the environment due to poorly managed waste disposal practices. It is crucial to consider alternative disposal options for biobased and compostable polymers like poly(lactic acid) (PLA), even on a household scale. Given the low temperatures typically encountered in home or backyard compost settings, the microorganisms present in compost or soil require an additional stimulus for effective decomposition. This study investigates the incorporation of various nutrients, including gelatin, skim milk, ethyl lactate, and iron nanopowder, to enhance the compost environment. The findings reveal that adding these compounds serve to biostimulate the compost environment, facilitating accelerated biodegradation of PLA that would otherwise take an extended period (over two years) to break down naturally. These results open opportunities for considering home composting as a viable alternative to industrial composting to dispose of PLA.
|
1. Introduction
Poly(lactic acid) – PLA – is a biobased, biodegradable polymer that is an eco-friendly substitute for fossil-based polymers for a circular and sustainable economy. PLA is derived from natural resources and quickly degrades and breaks down in suitable waste management environments, such as industrial composting. PLA is a versatile biobased polymer because it has properties comparable to conventional polymers, is cost-effective, and can provide an additional disposal scenario, namely composting, at the end of a contaminated package life cycle.1 These benefits, combined with the growing consumer awareness of plastic littering and white pollution, have propelled PLA to the forefront as the face of the green, biobased plastic movement.2
Although PLA is industrially compostable, its practical and rapid biodegradation depends on reaching temperatures in the thermophilic range, 45 to 60 °C, to undergo chemical hydrolysis and significantly reduce its molecular weight in a shorter period so that microorganisms present in the compost can use PLA oligomers as a food source. This constraint makes it difficult to degrade PLA at lower and ambient temperatures (i.e., mesophilic range, 20 to 45 °C).3 Chemical hydrolysis is a crucial step in the PLA degradation mechanism, involving the breakdown of high into low molecular-weight polymer chains, such as oligomers, dimers, and monomers, which are easily assimilated by microorganisms.4 However, at the lower temperatures commonly encountered in the home or backyard composting environments (herein referred to as backyard composting), chemical hydrolysis proceeds at a prolonged pace.5 Boosting the hydrolytic degradation of PLA, particularly in backyard composting, is extremely difficult due to the limitation of high temperature essential to activate the biodegradation process.3
Several research studies have included nanocomposites and metal compounds within the PLA matrix at lower concentrations to enhance its depolymerization and degradation.6–8 Including these metal oxides and nanoparticles significantly alters physical properties, such as the number average molecular weight (Mn), melting temperature (Tm), and glass transition temperature (Tg)9–11 of the resulting PLA or PLA blends, and may not be practical for food contact or single-use PLA discarded packages.
Enzymatic hydrolysis involving the release of extracellular enzymes is also essential for PLA degradation when low molecular weight PLA chains are available. PLA's degrading enzymes include the hydrolase class of enzymes, primarily proteases.3 The serine proteases released by the microorganisms in response to the presence of amino acid compounds in compost have been shown to cleave the ester bonds in PLA.12,13 So, the introduction of enzymes to compost may assist in the biodegradation of PLA.
Adding various components besides enzymes, such as nutrients, electron donor/acceptor compounds, or compounds essential to trigger the biochemical processes of microorganisms in the given environment, is termed biostimulation.14–16 Biostimulation techniques to enhance PLA biodegradation have been reported.17 However, most of these studies were conducted in restricted settings where PLA was the only carbon source, in liquid media with specific microbial strains, and usually at higher temperatures, which do not replicate the real conditions encountered during backyard composting.18,19
In a previous study, we assessed the change in PLA biodegradation at a mesophilic temperature of 37 °C in a solid composting matrix by adding biostimulants to the compost. The compounds selected as biostimulants in that study were screened through multiple criteria: anticipated to have no toxicity towards the microorganisms present, be able to degrade, be biodegradable in a suitable timeframe, be consumed, be cost-effective, and be readily available. The main goal was to introduce compounds enhancing the biotic degradation stage. Skim milk and gelatin were selected to trigger proteolytic activity. Ethyl lactate, belonging to the lactate esters family, was used to stimulate the lactate-utilizing microbial species in backyard compost. All these compounds effectively enhanced the biodegradation of PLA in simulated backyard composting by at least 15%, as determined by the accelerated reduction of Mn.20
In this study, we focused on evaluating the effect of adding a metal compound to catalyze the chemical hydrolysis of PLA and combine that with our previously work demonstrated biotic enhancement. Metals can be added externally to the compost media rather than within PLA for humification purposes, as previously reported21,22 and screening through the earlier criteria. Table 1 presents the permissible limits of metal compounds as derived from regulatory standards for heavy metals in agricultural soils (mg kg−1),23 since the resulting amended compost may be applied to agricultural land, lawns, or home gardens. The primary standards include EEA 2007,24 TMS 2007,25 BPI 2021,26 GB 15168-2018,27 OMOE 2011,28 and NZME 2012.29
Table 1 Critical limits of heavy metals in agricultural soils (mg kg−1), adapted from ref. 23
Country |
As |
Cd |
Cr |
Cu |
Hg |
Ni |
Pb |
Zn |
Australia |
20 |
3 |
50 |
100 |
1 |
60 |
300 |
200 |
Canada |
20 |
3 |
250 |
150 |
0.8 |
100 |
200 |
500 |
China |
20–40 |
0.3–0.6 |
150–300 |
50–200 |
0.5–3.4 |
60–190 |
70–240 |
200–300 |
Germany |
50 |
5 |
500 |
200 |
5 |
200 |
1000 |
600 |
Tanzania |
1 |
1 |
100 |
200 |
2 |
100 |
200 |
150 |
Netherlands |
76 |
13 |
180 |
190 |
36 |
100 |
530 |
720 |
New Zealand |
17 |
3 |
290 |
>104 |
200 |
N/A |
160 |
N/A |
UK |
43 |
1.8 |
N/A |
N/A |
26 |
230 |
N/A |
N/A |
US |
6.5 |
1.5 |
105 |
50 |
0.4 |
31 |
75 |
250 |
Considering the criteria mentioned before and the information from Table 1 only a few metals could be selected for further consideration due to limitations placed by permissible limits. The selected compounds were further scrutinized (Table 2) for their antibacterial properties, as reported in the literature, to evaluate their use directly in compost.
Table 2 List of elements screened for their antimicrobial properties
Element |
Antibacterial nature |
References |
Zn |
Yes |
30 and 31 |
Fe |
No |
30, 32 and 33 |
Cu |
Yes |
30
|
Ni |
Yes |
30
|
Ti |
Yes |
34 and 35 |
Cl |
Yes |
30 and 36 |
Ag |
Yes |
36 and 37 |
Table 2 indicates that iron was an acceptable compound that could be used to target chemical hydrolysis. It was demonstrated that introduction of a Lewis acid, FeCl3, can speed up the hydrolysis of PLA in an alkali solution.38 However, due to chlorine's antimicrobial behavior, it was impossible to introduce FeCl3 into compost. Alternatively, iron oxides, such as FeO, FeO2, Fe3O4, or Fe2O3, could be the primary option and are in a form that is not toxic to the microbes in the compost.32,33 Several of these iron forms are present in soil worldwide.39
Thus, this study aimed to investigate the effectiveness of biostimulating the compost environment with compounds that may be able to enhance the chemical hydrolysis (Fe3O4), the enzymatic degradation (skim milk and gelatin), and electroconductivity (ethyl lactate as electron donor compound) during the aerobic biodegradation of PLA in compost at mesophilic conditions. Differences in CO2 evolution, changes in Mn, and the crystallinity (Xc) of PLA degradation with and without biostimulants were monitored to account for the activity of biostimulants in compost.
2. Materials & methods
2.1. Materials
PLA Ingeo™ 2003D resin, with L-lactic acid content of 96%, was obtained from NatureWorks LLC (Minnetonka, MN, USA). Iron oxide nano-powder (Fe3O4) was obtained from US Research Nanomaterials, Inc. (Houston, TX, USA). Skim milk powder was procured from a local store (Walmart, Lansing, MI, USA). Gelatin of the brand McCormick & Co. (Hunt Valley, MD, USA) was purchased on Amazon LLC. Ethyl lactate was procured from Sigma Aldrich™ (St. Louis, MO, USA).
2.2. Characterization of PLA and the biostimulants
The carbon, hydrogen, and nitrogen compositions of PLA resin, skim milk, gelatin, and ethyl lactate were determined using elemental analysis, (CHNS/O Elemental Analyzer, PerkinElmer 2400 Series II) (Shelton, CT, USA), and are presented in Table 3.
Table 3 Carbon, hydrogen, and nitrogen content (percentage by weight) of the tested materials
Material |
% carbon |
% hydrogen |
% nitrogen |
PLA |
49.7 ± 0.2 |
5.7 ± 0.0 |
0.0 ± 0.0 |
Skim milk |
41.3 ± 0.1 |
6.3 ± 0.0 |
5.7 ± 0.1 |
Gelatin |
44.8 ± 0.3 |
7.0 ± 0.1 |
16.4 ± 0.1 |
Ethyl lactate |
31.9 ± 1.0 |
5.5 ± 0.3 |
0.0 ± 0.0 |
2.3. Biodegradation test in compost
The biodegradation of PLA and the effectiveness of introducing biostimulants in compost on the degradation of PLA were evaluated under aerobic mesophilic conditions using a direct measurement respirometric (DMR) system.40–42 The system included a non-dispersive infrared gas analyzer (NDIR) (Li-COR® LI-820, Lincoln, NE, USA) which measures the CO2 concentration. The DMR system chamber was maintained at temperature of 37 ± 2 °C and relative humidity (RH) of 50% ± 5%. A flow rate of CO2-free air (concentration <30 ppm to establish a low baseline) was controlled at 40 ± 2 cm3 min−1. Detailed information about the DMR equipment can be found in other source.43
The mature compost obtained from MSU composting facility was sifted using a 10 mm screen to get rid of any huge debris or chunks present, and then conditioned at 37 °C until use. Deionized water was used to adjust the moisture content of compost to 50%. Saturated vermiculite (Sun Gro Horticulture Distribution Inc., Bellevue, WA, USA), was mixed with compost in 1
:
4 parts (dry weight). Samples of the resulting compost-vermiculite mixture were later sent to the Soil and Plant Laboratory at MSU for determining the physicochemical parameters. Data regarding the nutrient analysis is presented in Table S1 in the ESI.†44 The bioreactors were packed with 400 g of compost, 8 g of PLA sample and the selected biostimulant, and all the samples were tested in triplicate. Blank (only compost) and positive control (cellulose) were also tested.
2.3.1 Biostimulation.
Fe3O4 nanopowder at 17 g was mixed with the compost matrix in the bioreactor containing PLA to target the hydrolysis step. Ethyl lactate, skim milk, and gelatin were added at 8 g individually per bioreactor and mixed with the compost matrix thoroughly with the PLA pellets in it to ensure uniform distribution.
2.4. Size exclusion chromatography (SEC)
The Mn and molecular weight distribution (MWD) of PLA for the control and each treatment with the biostimulants were measured using SEC (Waters Corp., Milford, MA, USA) as described elsewhere.4 PLA samples weighing approximately 10 mg were retrieved at predetermined time intervals and dissolved in 5 mL of tetrahydrofuran (THF) solvent. A temperature of 35 °C and a 1 mL min−1 flow rate were maintained during testing. The Mark–Houwink constants of K = 0.000174 dL g−1 and α = 0.736 were used to determine Mn, Mw, and MWD of the PLA samples. Data analysis was carried out using Waters Breeze™2 software.
2.5. Differential scanning calorimetry (DSC)
A DSC model Q100 (TA Instruments, New Castle, DE, USA), was used to determine the Tg, Tm, crystallization temperature (Tc), and crystallinity (Xc) for the PLA samples retrieved from the regular and biostimulated compost. PLA samples weighing between 5–10 mg were packed in aluminum pans and cooled down to −5 °C and then subjected to a heating cycle to reach 210 °C at a ramp rate of 10 °C min−1. This helped to evaluate the evolution of Xc. The cooling was achieved using a nitrogen cooling system that maintained the purge flow rate at 70 mL min−1. The degree of crystallinity was estimated using equation:1 | 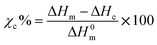 | (1) |
where ΔHm is the heat of fusion, ΔHc is cold crystallization enthalpy, and ΔH0m is the heat of fusion for 100% crystalline pure PLA (93 J g−1).45
3. Results & discussion
The CO2 evolution of PLA samples in compost and biostimulated with Fe3O4 nanopowder and the combination of gelatin, skim milk, and ethyl lactate without and with Fe3O4 nanopowder was tracked over a test duration of 180 days at mesophilic conditions (37 °C). Samples were retrieved at specific times to evaluate the Mn and Xc evolution and determine the kinetic degradation rate.
3.1. Effect of Fe3O4 on cellulose and PLA degradation
Fig. 1a and b show the CO2 evolution and mineralization, respectively, of cellulose, cellulose in compost biostimulated with Fe3O4 nanopowder (hereafter referred to as cellulose + Fe), PLA, and PLA in compost biostimulated with Fe3O4 nanopowder (hereafter referred to as PLA + Fe) at 37 °C. Control compost (blank) evolved around 26.1 g of CO2, and compost biostimulated with Fe3O4 nanopowder (hereafter referred to as blank + Fe) evolved around 27.2 g of CO2. The minor difference can be attributed to the difference in weight of the compost introduced in the bioreactor, and the levels are not significantly different (p > 0.05). Cellulose in compost evolved around 36.8 g of CO2, and reached a mineralization of 87.7%, whereas cellulose + Fe evolved around 44.6 g of CO2, depicting a mineralization of 137.7%. The primary reason for the priming effect (>100% mineralization) observed in the case of cellulose + Fe may be attributed to the over-deterioration of the endemic carbon present in the compost.4 PLA in compost showed around 22.5 g of CO2 evolution, whereas blank produced around 26.1 g of CO2, implying that no carbon from PLA was degraded. The negative mineralization values indicate more CO2 production in the blank bioreactors than in the PLA bioreactors. PLA offers a physical hydrophobic barrier to water, making it difficult for the microorganisms to utilize it as a carbon source at the beginning of the test and until day 180 due to the low contribution of chemical hydrolysis at mesophilic temperatures. Overall, we did not see any mineralization in PLA due to the low temperature of 37 °C, which is insufficient to activate the biotic stage. These values are very low compared to the degradation of PLA at thermophilic temperatures4,44 but are similar to earlier reported values.5,46
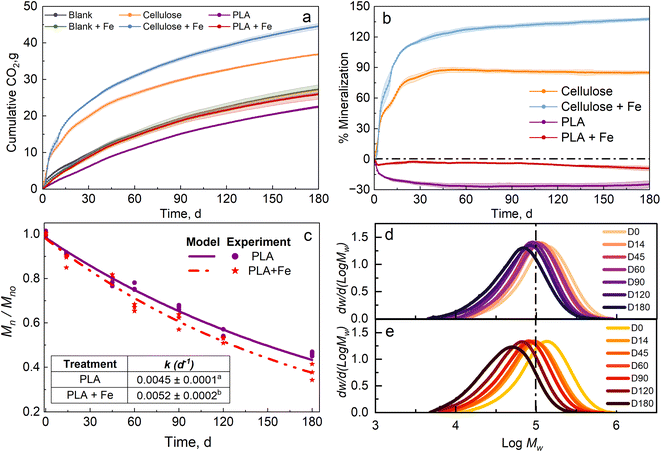 |
| Fig. 1 Cumulative CO2 evolution (a) and mineralization (b) of blank, cellulose, PLA, blank + Fe3O4 (blank + Fe), cellulose + Fe3O4 (cellulose + Fe), PLA + Fe3O4 (PLA + Fe) in compost at 37 °C. (c) Represents the normalized Mn reduction as a function of time for PLA in control compost and compost biostimulated with Fe3O4. The experimental data was fitted using a first-order reaction of the form Mn/Mno = e(−kt), where Mno is the initial Mn, k is the rate constant, and t is the time. The inset shows the k-fitted values; values with different lowercase letters are statistically different (α = 0.05, Tukey–Kramer test). (d) and (e) show the MWD of PLA in compost and compost biostimulated with Fe3O4, respectively. | |
In the case of PLA and PLA + Fe, we observed the difference in the CO2 evolution right from the start of the test. PLA + Fe evolved around 25.9 g of CO2 compared with 22.5 g of CO2 evolution for PLA. Similar to the case of cellulose + Fe, the reason for the difference in the CO2 evolution for PLA can be attributed to the presence of Fe3O4. Fe3O4 promotes microbial activity in the soil and enhances the nitrification potential.47 Fe3O4 is also known to induce changes by enhancing enzymatic activity and microbial growth.32 This characteristic can be corroborated by the kinetic degradation rate (k), as seen in Fig. 1c inset (PLA k = 0.0045 ± 0.0001 d−1 and PLA + Fe k = 0.0052 ± 0.0002 d−1). The significant difference can be credited to the presence of Fe3O4.Fig. 1d and e present the molecular weight distribution (MWD) for PLA in compost and compost biostimulated with Fe3O4. The peak amplitude for PLA in Fig. 1d remained approximately the same throughout the test duration. No broadening of the peak and negligible shift indicates that the chemical hydrolysis proceeded slowly at a mesophilic temperature of 37 °C. In contrast, for PLA + Fe, a significant shift to low Mw and broadening of the peak are observed in Fig. 1e, depicting the reduction in Mn as shown in Fig. 1c.
3.2.
M
n reduction for PLA with biostimulants in compost
PLA samples were retrieved separately from the control compost and compost biostimulated with skim milk, gelatin, and ethyl lactate. Fig. 2 shows the reduction in Mn of PLA and biostimulated PLA tracked until the end of the test (180 days). A significant difference was observed in the kinetic reduction rates of PLA with biostimulant treatment compared with no biostimulation treatment. The CO2 evolution values for PLA, PLA + skim milk, PLA + gelatin, and PLA + ethyl lactate are provided in Section S1 of the ESI.†
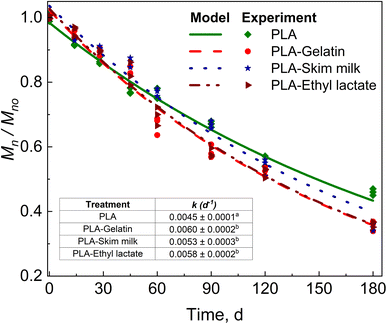 |
| Fig. 2 Normalized Mn reduction as a function of time for PLA in control compost and compost biostimulated with skim milk, gelatin, and ethyl lactate. The experimental data was fitted using a first-order reaction of the form Mn/Mno = e(−kt), where Mno is the initial Mn, k is the rate constant, and t is the time. The inset shows the k-fitted values; values with different lowercase letters are statistically different (α = 0.05, Tukey–Kramer test). | |
Skim milk was added to the compost to induce protease activity by the microbes.48–50 Serine protease (3.4.21.112) belongs to peptidases and is the class of extracellular enzymes able to hydrolyze the peptide bonds linked to amino acids in the protein structure. Skim milk is composed of different proteins, such as lactose, casein, and whey protein, making it a good precursor for enzymatic activity, as mentioned earlier. Other researchers have previously used skim milk to demonstrate extracellular protease synthesis. The microorganisms present in compost secrete protease to hydrolyze the milk protein. This protease is used by microorganisms capable of PLA degradation to depolymerize PLA.51 This increase in k can be deduced as a final reduction of around 75 days on the biodegradation time when PLA in compost biostimulated with skim milk reaches Mn ≲ 10 kDa at 420 days (Fig. 2). Microorganisms assimilate the PLA n-mers at this stage, accelerating the biodegradation stage.44 On the other hand, PLA needs at least 494 days to reach the same Mn – an effective 15% reduction of time.
Gelatin is composed of protein and amino acids, and is a precursor for protease activity.19,52–54 The addition of gelatin to compost produced an acceleration of PLA with an enhancement of k translated to a final reduction of around 124 days when PLA is biostimulated with gelatin, reaching an Mn ≲ 10 kDa at 371 days compared with at least 494 days for PLA alone – an effective 25% reduction of time (Fig. 2).
Ethyl lactate, on the other hand, was used to stimulate the lactate utilizing microbes in the compost. Ethyl lactate undergoes hydrolysis to produce ethanol and lactate, where both can act as a constant long-term supply of hydrogen sources as electron donor compounds for reductive degradation and microbial redox process. Lactate has been shown to act as an electron donor compound in the case of anaerobic degradation.55,56 Lactate has previously been used for anaerobic degradation, trichloroethane dichlorination, and sulfate reduction.57,58 PLA + ethyl lactate resulted in a change in k, which can be translated to around 111 days when PLA is biostimulated with ethyl lactate, reaching an Mn ≲ 10 kDa at 384 days compared to PLA alone – an effective 22% reduction of time (Fig. 2).
A detailed discussion of the effect of gelatin, skim milk, and ethyl lactate on the biodegradation of PLA is provided in our previous work.20
3.3. Effect of Fe3O4 on cellulose and PLA degradation with gelatin as a biostimulant
Since gelatin resulted in the most significant Mn reduction for PLA, it was selected to discuss the effect of a combination of biostimulants (i.e., Fe3O4 nanopowder and gelatin). The data for Fe3O4 nanopowder, skim milk, and ethyl lactate are provided in Section S3 of the ESI.† When Fe3O4 nanopowder was introduced in compost amended with gelatin, the gelatin acted as a precursor for the protease enzyme secretion by the microbes present in the compost and the Fe3O4 nanopowder provided the metal to catalyze the hydrolysis.
Fig. 3a and b show the CO2 evolution and mineralization of cellulose + Fe, gelatin + Fe, PLA + Fe, cellulose + gelatin + Fe (hereafter referred to as cell + gel + Fe), and PLA + gel + Fe in compost at 37 °C. Gelatin + Fe resulted in around 60.1 g of CO2 evolution and mineralization of 247.8% in 180 days. Gelatin was combined with Fe to target the chemical hydrolysis and enzymatic degradation steps. The CO2 evolution in this case (Cell + gel + Fe 1) was 64.6 g, which is as expected and higher compared with the individual values for cellulose + Fe and gelatin + Fe and a mineralization of 144.9%. To better understand the interaction and to account for the degradation behavior of cellulose in the presence of gelatin (Cell + gel + Fe 2), the mineralization value was estimated at 97.7% by subtracting the background signal from the bioreactor containing gelatin + Fe. This higher value indicates that cellulose degradation was not affected by gelatin, and both are used up by the microorganisms as carbon sources.
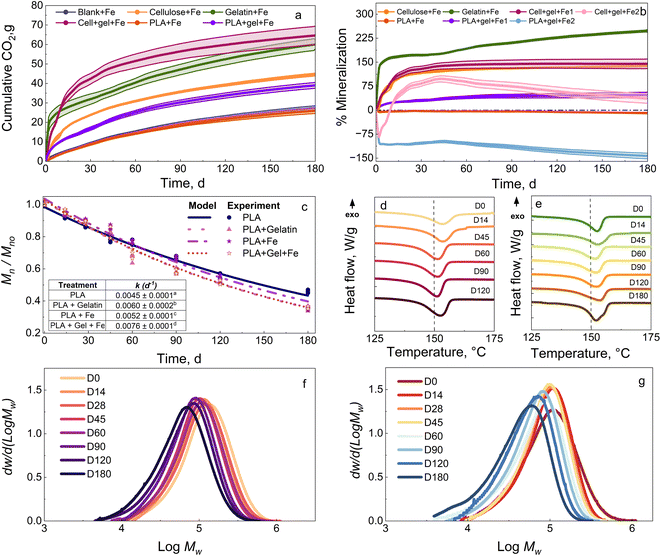 |
| Fig. 3 Cumulative CO2 evolution (a) and mineralization (b) of blank + Fe, cellulose + Fe, PLA + Fe, gelatin + Fe, cellulose + gelatin + Fe (Cell + gel + Fe), PLA + gelatin + Fe (PLA + gel + Fe) in compost at 37 °C. (c) Represents the normalized Mn reduction as a function of time for PLA in control compost and compost biostimulated with gelatin, and gelatin + Fe. The experimental data was fitted using a first-order reaction of the form Mn/Mno = e(−kt), where Mno is the initial Mn, k is the rate constant, and t is the time. The inset shows the k-fitted values; values with different lowercase letters are statistically different (α = 0.05, Tukey–Kramer test). (d) and (e) depict DSC thermograms for PLA + Fe and PLA + Fe in compost biostimulated with gelatin. (f) and (g) show the MWD of PLA + Fe in compost and compost biostimulated with gelatin. | |
To understand the influence of gelatin + Fe on PLA degradation, PLA was introduced in the compost amended with gelatin + Fe. The bioreactor containing both PLA and gelatin + Fe (PLA + gel + Fe 1) generated CO2 evolution of around 40.5 g and maximum mineralization of 56.5% by the end of the test. Improved mineralization was observed as opposed to no CO2 evolution for PLA alone without any biostimulation of the compost. The effect of gelatin + Fe on PLA degradation is calculated by plotting the mineralization of PLA + gel + Fe 2 (subtracting gelatin + Fe), as mentioned earlier. Negative mineralization does not necessarily indicate the absence of gelatin's protease activity in PLA's enzymatic degradation. This finding is validated by the significant difference observed in the kinetic rate of degradation for PLA in compost, with and without any biostimulation with gelatin, as seen in Fig. 3c inset (PLA + Fe k = 0.0052 ± 0.0001 and PLA + Gel + Fe k = 0.0076 ± 0.0002). The significant difference in the evolution of Xc from 28.3% to 31.4% for PLA, and from 28.3% to 40.9% for PLA biostimulated with gelatin + Fe, as seen in Fig. 3d and e, respectively, further shows the improvement in the enzymatic degradation of PLA due to the presence of gelatin + Fe. Gelatin acts as a precursor for the microbes to release protease enzyme, aiding in the enzymatic degradation of PLA. The broadening and change in the amplitude of the MWD peaks through the test duration of 180 days, as seen in Fig. 3g for PLA in compost biostimulated with gelatin + Fe compared to PLA + Fe alone in Fig. 3f, show compelling evidence for enhanced degradation for PLA in the presence of gelatin and Fe. Iron is an essential micronutrient, necessary for life-sustaining processes, and plays a critical role in cell growth of microbes.59 Iron also functions as a cofactor, promoting and increasing enzymatic activity. In addition, Iron plays an important role in various biological processes such as respiration, oxido-reduction mechanism, nitrogen fixation, tricarboxylic acid cycle, and electron transport.33 S. He et al. demonstrated that a soil matrix amended by Fe nanoparticles shifted the microbial community composition and stimulated the metabolic activity of the bacterial community present by enhancing their growth rate.33 The soil nitrification potential of the Fe-amended soil was improved from 10% to 19% compared to control soil, indicating that adding Fe aided in the increase of biomass capacity and eventually enhanced and boosted carbon cycling.
Zhang et al. further showed that the addition of Fe nanoparticles promoted the degradation of organic matter and amplified the dehydrogenase and urease activities, significantly improving the overall microbial activity and nitrogen mineralization.47 Thus, adding Fe3O4 nanopowder improves microbial metabolic activity, nitrification potential, and microbial population. When supplemented with the enzymatic activity associated with gelatin, these changes improve the degradation of PLA in compost compared with that of control PLA with no biostimulants present. Y. He et al. showed enhanced enzymatic and nitrification activity for organic matter degradation in a food-waste composting system due to compost amendment with Fe-carbon particles.60 In addition, the bacterial and fungal communities exhibited significant improvement in the composting process due to the presence of iron, which can explain the improved PLA degradation found in the presence of Fe3O4 nanopowder.
Overall, the changes obtained in k indicate a final reduction of around 148 days when PLA stimulated with gelatin reaches an Mn ≲ 10 kDa at 346 days compared to at last 494 days for PLA – an effective 25% reduction of time. Similarly, time reductions of 17% or 30% were observed when PLA + Fe3O4 or gelatin and Fe3O4 nanopowder, respectively, when included in the compost.
As organic waste disposal is becoming more stringent worldwide and landfill disposal bans are increasing, several food industries are being impacted and need to find alternative end-of-life scenarios. The gelatin industry produces a large amount of sludge, resulting in a tremendous amount of waste generated, which includes collagen fibers, bone residues, and other inorganic materials. This gelatin sludge usually ends up in landfill or waste management treatment without any pretreatment and creates several problems such as water contamination, greenhouse gas emission, and health risks for local habitats.61 So, this waste could be diverted from landfills and used to produce mature compost along with the organic fraction of municipal solid waste. Gelatin sludge is high in nitrogen and organic matter content and can act as a valuable plant nutrient.61 The nutritional value of the compost generated from treating gelatin waste and Fe3O4 nanopowder, considering the benefits mentioned earlier, complement each other to improve soil fertility once the compost is applied to agricultural land. The selection and combination of specific compounds can open a new route to accelerate the degradation of compostable polymers in industrial and home composting operations.
4. Conclusion
We investigated the role of different compounds—skim milk, gelatin, and ethyl lactate in combination with Fe3O4 nanopowder—on PLA degradation at 37 °C by biostimulating the compost media. The different compounds were selected to target different stages of the biodegradation process. Without any biostimulant compounds, PLA continued to undergo a long abiotic lag phase affirming a slow hydrolysis phase, which was seen as a negative mineralization for the test duration of 180 days, whereas a boost in CO2 evolution for PLA was observed in compost amended by Fe3O4 nanopowder in combination with gelatin, skim milk, or ethyl lactate. This observation was verified by the molecular weight change and crystallinity evolution. PLA biodegradation was accelerated by 30% to reach the biotic phase of Mn ≲ 10 kDa by the addition of gelatin and Fe3O4 nanopowder. The addition of biostimulants opens new avenues to improve PLA biodegradation in home composting conditions.
Author contributions
P. M. and R. A. conceived and designed the experiments; P. M. performed the experiments, analyzed the data, and drafted the manuscript. The authors thank Anibal Bher for helping with the test, data acquisition, and discussion about biodegradation. R. A. provided mentoring and financial support. All authors have read, reviewed, and agreed to the published version of the manuscript.
Conflicts of interest
The authors declare no conflict of interest.
Acknowledgements
P. M. acknowledges the School of Packaging at Michigan State University; R. A. acknowledges the USDA National Institute of Food and Agriculture and Michigan State University AgBioResearch, Hatch project number MICL02665, for partial study support.
References
-
R. Auras, L.-T. Lim, S. E. M. Selke and H. Tsuji, Poly(lactic Acid) : Synthesis, Structures, Properties, Processing, Applications, and End of Life, 2022 Search PubMed.
- K. Ghosh and B. H. Jones, Roadmap to Biodegradable Plastics—Current State and Research Needs, ACS Sustain. Chem. Eng., 2021, 9(18), 6170–6187 CrossRef CAS . Available from: https://pubs.acs.org/doi/10.1021/acssuschemeng.1c00801.
- A. Bher, P. C. Mayekar, R. A. Auras and C. E. Schvezov, Biodegradation of Biodegradable Polymers in Mesophilic Aerobic Environments, Int. J. Mol. Sci., 2022, 23(20), 12165 CrossRef CAS PubMed.
- P. C. Mayekar, E. Castro-Aguirre, R. Auras, S. Selke and R. Narayan, Effect of nano-clay and surfactant on the biodegradation of poly(lactic acid) films, Polymers, 2020, 12(2), 1–16 CrossRef PubMed.
- P. C. Mayekar, W. Limsukon, A. Bher and R. Auras, Breaking It Down: How Thermoplastic Starch Enhances Poly(lactic acid) Biodegradation in Compost – A Comparative Analysis of Reactive Blends, ACS Sustain. Chem. Eng., 2023, 11(26), 9729–9737 CrossRef CAS.
- V. Botvin, S. Karaseva, V. Khasanov and A. Filimoshkin, Kinetic study of depolymerization of lactic and glycolic acid oligomers in the presence of oxide catalysts, Polymers, 2020, 12(10), 1–19 CrossRef PubMed.
- M. Qu, H. Tu, M. Amarante, Y. Q. Song and S. S. Zhu, Zinc oxide nanoparticles catalyze rapid hydrolysis of poly(lactic acid) at low temperatures, J. Appl. Polym. Sci., 2014, 131(11), 1–7 CrossRef.
- Y. Luo, Z. Lin and G. Guo, Biodegradation Assessment of Poly (Lactic Acid) Filled with Functionalized Titania Nanoparticles (PLA/TiO2) under Compost Conditions, Nanoscale Res. Lett., 2019, 14(56), 1–10 Search PubMed.
- A. Anžlovar, A. Kržan and E. Žagar, Degradation of PLA/ZnO and PHBV/ZnO composites prepared by melt processing, Arabian J. Chem., 2018, 11(3), 343–352 CrossRef.
- Y. B. Luo, X. L. Wang and Y. Z. Wang, Effect of TiO2 nanoparticles on the long-term hydrolytic degradation behavior of PLA, Polym. Degrad. Stab., 2012, 97(5), 721–728 CrossRef CAS.
- E. Lizundia, L. Ruiz-Rubio, J. L. Vilas and L. M. León, Towards the development of eco-friendly disposable polymers: ZnO-initiated thermal and hydrolytic degradation in poly(l-lactide)/ZnO nanocomposites, RSC Adv., 2016, 6(19), 15660–15669 RSC.
- H.-A. Lim, T. Raku and Y. Tokiwa, Hydrolysis of polyesters by serine proteases, Biotechnol. Lett., 2005, 27(7), 459–464 CrossRef CAS PubMed . Available from: http://link.springer.com/10.1007/s10529-005-2217-8.
- H. Pranamuda, A. Tsuchii and Y. Tokiwa, Poly (L-lactide)-Degrading Enzyme Produced byAmycolatopsis sp, Macromol. Biosci., 2001, 1(1), 25–29 CrossRef CAS . Available from: https://onlinelibrary.wiley.com/doi/10.1002/1616-5195(200101)1:1%<?pdb_no 3C25?>3C25<?pdb END?>::AID-MABI25%3E3.0.CO;2-3.
- Y. Tokiwa and B. P. Calabia, Biodegradability and biodegradation of poly(lactide), Appl. Microbiol. Biotechnol., 2006, 72(2), 244–251 CrossRef CAS PubMed.
- D. B. Watson, W.-M. Wu, T. Mehlhorn, G. Tang, J. Earles and K. Lowe,
et al., In Situ Bioremediation of Uranium with Emulsified Vegetable Oil as the Electron Donor, Environ. Sci. Technol., 2013, 47(12), 6440–6448 CrossRef CAS PubMed . Available from: https://pubs.acs.org/sharingguidelines.
- L. Cosgrove, P. L. McGeechan, P. S. Handley and G. D. Robson, Effect of biostimulation and bioaugmentation on degradation of polyurethane buried in soil, Appl. Environ. Microbiol., 2010, 76(3), 810–819 CrossRef CAS PubMed . Available from: http://ddsdx.uthscsa.edu/dig/itdesc.html.
- Y. Boonluksiri, B. Prapagdee and N. Sombatsompop, Promotion of polylactic acid biodegradation by a combined addition of PLA-degrading bacterium and nitrogen source under submerged and soil burial conditions, Polym. Degrad. Stab., 2021, 188 Search PubMed.
- K.
S. Bonifer, X. Wen, S. Hasim, E. K. Phillips, R. N. Dunlap and E. R. Gann,
et al., Bacillus pumilus B12 Degrades Polylactic Acid and Degradation Is Affected by Changing Nutrient Conditions, Front. Microbiol., 2019, 10, 1–13 CrossRef PubMed . Available from: https://www.frontiersin.org/article/10.3389/fmicb.2019.02548/full.
- T. Apinya, N. Sombatsompop and B. Prapagdee, Selection of a Pseudonocardia sp. RM423 that accelerates the biodegradation of poly(lactic) acid in submerged cultures and in soil microcosms, Int. Biodeterior. Biodegrad., 2015, 99, 23–30 CrossRef CAS . Available from: https://linkinghub.elsevier.com/retrieve/pii/S0964830515000037.
- P. C. Mayekar and R. Auras, Accelerating Biodegradation : Enhancing Poly(lactic acid) Breakdown at Mesophilic Environmental Conditions with Biostimulants, Macromol. Rapid Commun., 2023, 2300641, 1–10 Search PubMed.
- S. Zhang, Z. Wei, M. Zhao, X. Chen, J. Wu and K. Kang,
et al., Influence of malonic acid and manganese dioxide on humic substance formation and inhibition of CO2 release during composting, Bioresour. Technol., 2020, 318, 124075, DOI:10.1016/j.biortech.2020.124075.
- J. Mei, K. Ji, L. Su, M. Wu, X. Zhou and E. Duan, Effects of FeSO4 dosage on nitrogen loss and humification during the composting of cow dung and corn straw, Bioresour. Technol., 2021, 341, 125867, DOI:10.1016/j.biortech.2021.125867.
- Z. He, J. Shentu, X. Yang, V. C. Baligar, T. Zhang and P. J. Stoffella, Heavy Metal Contamination of Soils: Sources, Indicators, and Assessment, Journal of Environmental Indicators, 2015, 9, 17–18 Search PubMed.
-
European Environmental Agency, Progress in Management of Contaminated Sites (CSI 015/LSI 003), 2007, https://www.eea.europa.eu/data-and-maps/indicators/progress-in-management-of-contaminated-sites-3/assessment Search PubMed.
-
Tanzania Minister of State (TMS), The Environmental Management (Soil Quality Standards) Regulations, 2007 Search PubMed.
-
Biodegradable Products Institute, BPI – Heavy Metals, 2021 Search PubMed.
-
Ministry of Environment Protection of the People’s Republic of China, Soil Environmental Quality Risk Control Standard for Soil Contamination of Agricultural Land, 2018 Search PubMed.
-
Ontario Ministry of the Environment (OMOE), Soil, Ground Water and Sediment Standards for Use under Part XV.1 of the Ontario Protection Act, 2011 Search PubMed.
-
New Zealand Ministry for the Environment (NZME), Users' Guide National Environmental Standard for Assessing and Managing Contaminants in Soil to Protect Human Health, 2012 Search PubMed.
- M. Yasuyuki, K. Kunihiro, S. Kurissery, N. Kanavillil, Y. Sato and Y. Kikuchi, Antibacterial properties of nine pure metals: a laboratory study using Staphylococcus aureus and Escherichia coli, Biofouling, 2010, 26(7), 851–858 CrossRef CAS PubMed . Available from: https://www.tandfonline.com/action/journalInformation?journalCode=gbif20.
- E. Hernández-García, M. Vargas, C. González-Martínez and A. Chiralt, Biodegradable antimicrobial films for food packaging: Effect of antimicrobials on degradation, Foods, 2021, 10(6), 1256 CrossRef PubMed.
- L. Zhang, H. Dong, Y. Zhu, J. Zhang, G. Zeng and Y. Yuan,
et al., Evolutions of different microbial populations and the relationships with matrix properties during agricultural waste composting with amendment of iron (hydr)oxide nanoparticles, Bioresour. Technol., 2019, 289, 121697 CrossRef CAS PubMed.
- S. He, Y. Feng, J. Ni, Y. Sun, L. Xue and Y. Feng,
et al., Different responses of soil microbial metabolic activity to silver and iron oxide nanoparticles, Chemosphere, 2016, 147, 195–202 CrossRef CAS PubMed.
- V. L. Pachapur, A. Dalila Larios, M. Cledón, S. K. Brar, M. Verma and R. Y. Surampalli, Behavior and characterization of titanium dioxide and silver nanoparticles in soils, Sci. Total Environ., 2016, 563–564, 933–943, DOI:10.1016/j.scitotenv.2015.11.090.
- M. Kaseem, K. Hamad and Z. U. Rehman, Review of Recent Advances in Polylactic Acid/TiO2 Composites, Materials, 2019, 12, 1–16 Search PubMed.
- J. Kim, B. Pitts, P. S. Stewart, A. Camper and J. Yoon, Comparison of the Antimicrobial Effects of Chlorine, Silver Ion, and Tobramycin on Biofilm, Antimicrob. Agents Chemother., 2008, 52(4), 1446 CrossRef CAS PubMed . Available from:/pmc/articles/PMC2292569/.
- G. Gorrasi, A. Sorrentino and R. Pantani, Modulation of Biodegradation Rate of Poly(lactic acid) by Silver Nanoparticles, J. Polym. Environ., 2015, 23, 316–320 CrossRef CAS.
- X. Li, S. Gong, L. Yang, F. Zhang, L. Xie and Z. Luo,
et al., Study on the degradation behavior and mechanism of Poly(lactic acid) modification by ferric chloride, Polymer, 2020, 188, 121991 CrossRef CAS.
-
EPA, Ecological Soil Screening Level for Iron Interim Final, US Environ Prot Agency – Off Solid Waste Emerg, 2005, p. 211, https://rais.ornl.gov/documents/eco-ssl_iron.pdf Search PubMed.
-
ASTM International, ASTM D5338-15(2021): Standard Test Method for Determining Aerobic Biodegradation of Plastic Materials Under Controlled Composting Conditions, Incorporating Thermophilic Temperatures, in ASTM Standards, 2021, pp. 1–7 Search PubMed.
-
International Standard ISO/FDIS 14855-1:2012, Determination of the Ultimate Aerobic Biodegradability of Plastic Materials under Controlled Composting Conditions - Method by Analysis of Evolved Carbon Dioxide, Part 1: General Method, 2012, p. 20 Search PubMed.
- T. Kijchavengkul, R. Auras, M. Rubino, M. Ngouajio and R. Thomas Fernandez, Development of an automatic laboratory-scale respirometric system to measure polymer biodegradability, Polym. Test., 2006, 25(8), 1006–1016 CrossRef CAS . Available from: www.elsevier.com/locate/polytest.
-
E. Castro-Aguirre, Design and Construction of A Medium-Scale Automated Direct Measurement Respirometric System to Assess Aerobic Biodegradation of Polymers, MSc Thesis, Michigan State University, East Lansing, MI, 2013.
- E. Castro-Aguirre, R. Auras, S. Selke, M. Rubino and T. Marsh, Insights on the aerobic biodegradation of polymers by analysis of evolved carbon dioxide in simulated composting conditions, Polym. Degrad. Stab., 2017, 137, 251–271, DOI:10.1016/j.polymdegradstab.2017.01.017.
- P. C. Mayekar, E. Castro-Aguirre, R. Auras, S. Selke and R. Narayan, Effect of Nano-Clay and Surfactant on the Biodegradation of Poly(Lactic Acid) Films, Polymers, 2020, 12(2), 311–2020 CrossRef CAS PubMed ):311. Available from: https://www.mdpi.com/2073-4360/12/2/311/htm.
- S. M. Satti, A. A. Shah, T. L. Marsh and R. Auras, Biodegradation of Poly(lactic acid) in Soil Microcosms at Ambient Temperature: Evaluation of Natural Attenuation, Bio-augmentation and Bio-stimulation, J. Polym. Environ., 2018, 26(9), 3848–3857 CrossRef CAS . Available from: http://link.springer.com/10.1007/s10924-018-1264-x.
- L. Zhang, Y. Zhu, J. Zhang, G. Zeng, H. Dong and W. Cao,
et al., Impacts of iron oxide nanoparticles on organic matter degradation and microbial enzyme activities during agricultural waste composting, Waste Manage., 2019, 95, 289–297, DOI:10.1016/j.wasman.2019.06.025.
- A. N. Mistry, B. Kachenchart, A. Wongthanaroj, A. Somwangthanaroj and E. Luepromchai, Rapid biodegradation of high molecular weight semi-crystalline polylactic acid at ambient temperature via enzymatic and alkaline hydrolysis by a defined bacterial consortium, Polym. Degrad. Stab., 2022, 202 Search PubMed.
- D. Rupali, Screening and Isolation of Protease Producing Bacteria from Soil Collected from Different Areas of Burhanpur Region (MP) India, Int. J. Curr. Microbiol. Appl. Sci., 2015, 4(8), 597–606 CAS . Available from: http://www.ijcmas.com.
- W. Penkhrue, C. Khanongnuch, K. Masaki, W. Pathom-aree, W. Punyodom and S. Lumyong, Isolation and screening of biopolymer-degrading microorganisms from northern Thailand, World J. Microbiol. Biotechnol., 2015, 31(9), 1431–1442 CrossRef CAS PubMed . Available from: http://link.springer.com/10.1007/s11274-015-1895-1.
- F. Decorosi, M. L. Exana, F. Pini, A. Adessi, A. Messini and L. Giovannetti,
et al., The Degradative Capabilities of New Amycolatopsis Isolates on Polylactic Acid, Microorganisms, 2019, 7(12), 590 CrossRef CAS PubMed . Available from: https://www.mdpi.com/2076-2607/7/12/590.
- A. Jarerat, Y. Tokiwa and H. Tanaka, Poly(l-lactide) degradation by Kibdelosporangium aridum, Biotechnol. Lett., 2003, 25(23), 2035–2038 CrossRef CAS PubMed . Available from: http://link.springer.com/10.1023/B:BILE.0000004398.38799.29.
- A. Jarerat and Y. Tokiwa, Poly(L-lactide) degradation by Saccharothrix waywayandensis, Biotechnol. Lett., 2003, 25(5), 401–404 CrossRef CAS PubMed . Available from: http://link.springer.com/10.1023/A:1022450431193.
- A. Jarerat and Y. Tokiwa, Degradation of Poly(L-lactide) by a Fungus, Macromol. Biosci., 2001, 1(4), 136–140 CrossRef CAS . Available from: https://onlinelibrary.wiley.com/doi/10.1002/1616-5195(20010601)1:4%3C136::AID-MABI136%3E3.0.CO;2-3.
- T. Jiang, C. Gao, C. Ma and P. Xu, Microbial lactate utilization: Enzymes, pathogenesis, and regulation, Trends Microbiol., 2014, 22(10), 589–599 CrossRef CAS PubMed.
-
A. Farhana and S. L. Lappin, Biochemistry, Lactate Dehydrogenase, StatPearls, 2021, pp. 1–11, https://www.ncbi.nlm.nih.gov/books/NBK557536/ Search PubMed.
- J. Li, A. Hu, S. Bai, X. Yang, Q. Sun and X. Liao,
et al., Characterization and performance of lactate-feeding consortia for reductive dechlorination of trichloroethene, Microorganisms, 2021, 9(4), 1–18 Search PubMed.
- A. M. dos Santos, J. M. Costa, J. K. Braga, T. M. Flynn, G. Brucha and G. P. Sancinetti,
et al., Lactate as an effective electron donor in the sulfate reduction: impacts on the microbial diversity, Environ. Technol., 2022, 43(20), 3149–3160, DOI:10.1080/09593330.2021.1916092.
- C. Colombo, G. Palumbo, J.-Z. He, R. Pinton and S. Cesco, Review on iron availability in soil: interaction of Fe minerals, plants, and microbes, J. Soils Sediments, 2014, 14, 538–548 CrossRef CAS.
- Y. He, X. Huang, H. Zhang, H. Li, Y. Zhang and X. Zheng,
et al., Insights into the effect of iron-carbon particle amendment on food waste composting: Physicochemical properties and the microbial community, Bioresour. Technol., 2022, 351, 126939, DOI:10.1016/j.biortech.2022.126939.
- M. K. Awasthi, A. K. Pandey, P. S. Bundela, J. W. C. Wong, R. Li and Z. Zhang, Co-composting of gelatin industry sludge combined with organic fraction of municipal solid waste and poultry waste employing zeolite mixed with enriched nitrifying bacterial consortium, Bioresour. Technol., 2016, 213, 181–189, DOI:10.1016/j.biortech.2016.02.026.
|
This journal is © The Royal Society of Chemistry 2024 |
Click here to see how this site uses Cookies. View our privacy policy here.