Quantitative thermodynamic exposure assessment of PCBs available to sandworms (Alitta virens) in activated carbon remediated sediment during ongoing sediment deposition†
Received
12th September 2023
, Accepted 7th January 2024
First published on 12th February 2024
Abstract
Marine mesoscale studies with sandworms (Alitta virens) were conducted to isolate important processes governing the exposure and bioaccumulation of polychlorinated biphenyls (PCBs) at contaminated sediment sites. Ex situ equilibrium sampling with silicone-coated jars, and in situ passive sampling with low-density polyethylene (LDPE) were used to determine the performance of an activated carbon (AC) amendment remedy applied to the bed sediment. A quantitative thermodynamic exposure assessment (‘QTEA’) was performed, showing that PCB concentrations in polymers at equilibrium with the surficial sediment were suited to measure and assess the remedy effectiveness with regard to PCB bioaccumulation in worms. In practice, monitoring the performance of sediment remedies should utilize a consistent and predictive form of polymeric sampling of the sediment. The present study found that ex situ equilibrium sampling of the surficial sediment was the most useful for understanding changes in bioaccumulation potential as a result of the applied remedy, during bioturbation and ongoing sediment and contaminant influx processes. The ultrathin silicone coatings of the ex situ sampling provided fast equilibration of PCBs between the sediment interstitial water and the polymer, and the multiple coating thicknesses were applied to confirm equilibrium and the absence of surface sorption artifacts. Overall, ex situ equilibrium sampling of surficial sediment could fit into existing frameworks as a robust and cost-effective tool for contaminated sediment site assessment.
Environmental significance
Critical processes governing the exposure of polychlorinated biphenyls (PCBs) at contaminated sediment sites were isolated in the laboratory. Processes affecting bioaccumulation of PCBs in sediment dwelling worms could be simplified, for remedy effectiveness monitoring, to the PCB concentration in polymers at equilibrium with the surficial sediment. Ex situ equilibrium sampling of the sediment will be a robust tool for determining the bioaccumulation potential at field sites experiencing ongoing influx of sediment, following application of AC amendment as a remedial treatment. Overall, ex situ equilibrium sampling of surficial sediment could provide better input data for Biota-Sediment Accumulation Factor (BSAF) models, compared to organic carbon normalized sediment concentrations.
|
Introduction
Contaminated sediment sites require dedicated environmental monitoring before, during, and after remedial activities to assess remedial efficacy. Of the remedial approaches available, mixing or amending contaminated sediments with activated carbon (AC) is increasingly used for sorbing hydrophobic organic contaminants (HOCs) and thereby lowering their freely dissolved concentrations (Cfree) and, consequently, their bioavailability in the contaminated sediment.1–5 Meanwhile, ongoing influx of fresh sediment and associated HOCs is a common occurrence at contaminated sediment sites, which are often located in protected net depositional areas.6 Ongoing input sources, both dissolved and particulate associated, have challenged remediation and monitoring efforts at many contaminated sediment sites.7–13 Natural and anthropogenic sediment processes at field sites, include: bioturbation, ongoing sediment influx/deposition, groundwater discharge, propellor wash from ship traffic, nearby maintenance dredging activity, sediment erosion and scour from flooding, and tidal and seasonal effects. Because of the complexity created by these processes, field studies of AC amendment remediation are often unable to isolate bioturbation and ongoing influx as the only relevant processes.
Existing frameworks available for monitoring contaminated sediment sites often utilize a combination of measurements in bulk phase sediment and in the water column (i.e., exhaustive extractions to quantify total chemical concentrations), determination of bioaccumulation, and increasingly passive sampling. Passive sampling using various polymers has gained recognition as a useful tool for determining the Cfree of HOCs in sediment interstitial water at sites undergoing remediation.14–16 In addition to Cfree, directly measuring and monitoring polymer concentrations is highly informative, as equilibrium concentrations of HOCs in polymers can be directly related to the “thermodynamic potential for bioaccumulation”.17–20 In addition, polymers are being explored as a tool to predict bioaccumulation, as HOC concentrations in polymer and HOC concentrations in biota lipid are highly correlated.21–23 Consequently, the most immediate implementation of the polymeric sampling techniques, into existing risk assessment and monitoring frameworks, might be to replace organic carbon normalized bulk-phase sediment measurements in the Biota-Sediment Accumulation Factor (BSAF)24–31 with polymeric sampling of the sediment.17,18,20–22 While the need for this type of assessment has been known for two decades,32,33 the practical implementation of polymers and BSAFs at contaminated sediment sites, undergoing remediation with AC, is still an area of scientific inquiry and debate. In this study, two polymeric sampling approaches were explored for establishing thermodynamic reference levels of polychlorinated biphenyls (PCBs) in sediment. The approaches were evaluated for understanding bioaccumulation potential during AC remediation and ongoing influx in a series of mesocosm experiments. The study and results presented in this article are part of a larger investigation (ESI, Fig. S1†).34,35
The present study compared PCB polymeric sampling to sandworm bioaccumulation in 90 days mesocosm experiments containing New Bedford Harbor sediment, which can be considered highly contaminated.22 The New Bedford Harbor site and sediment have been a focus of past and present contaminated sediment research.1,2,21,34–38 The mesocosms included ongoing influx of ‘fresh’ clean or contaminated sediment and bioturbation as processes driving contaminant transport between AC remediated bed sediments and the water column. The objectives of this research were to perform a quantitative thermodynamic exposure assessment (‘QTEA’) of native bedded PCBs in mesocosms and sandworms (Alitta virens), with regard to: (1) the presence or absence of AC mixed into the bed sediment, (2) the presence or absence of spiked PCBs on the input sediment (i.e., sediment introduced periodically to simulate ongoing deposition), and (3) the polymeric sampling method: in situ passive (pre-equilibrium) sampling with low density polyethylene (LDPE) (passive sampling devices, PSDs) or ex situ equilibrium sampling with silicone coated jars (equilibrium sampling devices, ESDs). Equilibrium concentrations in polymers were converted to concentrations in lipid at equilibrium with the sediment, which then served as a thermodynamic reference level to assess and compare with lipid-normalized tissue concentrations determined in worms.
The type(s) of biota used in mesocosm studies are an important feature allowing experiments to resemble critical aspects of the natural environment.6,39 The use of sandworms (A. virens; formerly Nereis virens), has implications on the bioturbation, fate, and transport of HOCs like PCBs.6,36,40,41 Large marine sandworms make U-shaped galleries or burrows39,41 approximately 8–10 cm in depth,27,28,40 with maximum depths of 45 cm in the field.42 The sandworms irrigate the burrows, and the burrow walls provide for more contaminant transport than uncolonized bed sediment.40,43 The walls remain well oxidized,40,41,43 and the burrows effectively increase the surface area of the sediment–water interface.44 Along with their role in enhancing geochemical processes, sandworms are ecologically significant, forming the base of the food chain in some habitats, as prey for lobsters, fish, and birds.26,42
Sandworms have a variety of behaviors influencing their exposure to PCBs; for example, sandworms feed by extending a portion of their bodies from their burrow to deposit feed.29,40 In addition, they have been reported to process large volumes of sediment or settled detritus, and sediment ingestion is considered a major route of contaminant uptake.29,45,46 Uptake via direct exposure to sediment interstitial water is also a portion of total uptake,45 and sandworms can take up dissolved organic matter through absorption mechanisms.40 Further, PCBs in surface water contribute to sandworm bioaccumulation, as sandworms irrigate their burrows with the overlying water.39,45
Sandworms have a long history of use in bioaccumulation testing of dredged material and contaminated sediment.24,25,27,28,31,45,47 The time necessary for PCBs to reach steady-state in sandworms depends on many factors (e.g., PCB concentrations, organism lipid content, sediment organic carbon), but under routine testing conditions, steady-state should be reached within 90 days.24,26,27,29,45,48 Klosterhaus et al.29 reported that HOCs with log
KOW > 6.7 would require >28 days to reach steady-state. This would apply to the more hydrophobic PCBs included in the present study.
While bioaccumulation is often regarded as the “true” indicator of bioavailability – biology is highly complex. The complex biology of the sandworm, and other potential living surrogates for bioaccumulation, make polymers a desirable alternative to assess the bioavailability of HOCs in sediment systems. The use of polymeric sampling to monitor the progress of sediment remedial activities is of great interest, not only in research, but also in practice by environmental managers.14,16
Methods and materials
Experimental design
The general layout of the 90 day marine mesocosm experiments have been described previously.1,2,37 and is presented in the ESI (Fig. S1†). This study examined two different polymeric sampling approaches for a ‘QTEA’ of PCBs in mesocosms containing sandworms, A. virens (hereafter simply denoted “worm” or “worms”). The mesocosms also included bivalves (Mercenaria mercenaria, hereafter, “clams”) and fish (Cyprinodon variegatus) – however, only the worms will be discussed in detail in this article (Fig. S1†). Animal testing was conducted using methods and protocols approved by the US Army, Engineer Research and Development Center's Institutional Animal Care and Use Committee (IACUC Protocol # EL-6009-2014-6). The mesocosms were comprised of 52 L glass aquaria, measuring 51 × 25 × 41 cm3 (length × width × height), with bed sediment (∼6 cm depth) that was AC amended or a control with no amendment, a surface water layer (∼32 cm depth), and biota (“E2” and “E3” in Fig. S1†). A control with no amendment or biota (“E1” in Fig. S1†) was also monitored by polymeric sampling, but was not the primary focus of the present study. Results from this no biota control treatment (E1) are presented later in this article. The AC was a coal-based Taste Odor Grade (TOG) type from Calgon Carbon Corporation (80 × 325 mesh, ∼32 to 184 μm) applied at a dose of 4.3% by dry weight (∼native total organic carbon (TOC) level of the sediment). The AC was pre-mixed into the bed sediment for 1 month of active mixing to advance the sediment-to-AC mass transfer of PCBs. In addition to the AC amendment treatment, E3 (denoted “AC mix”), ongoing sediment influxes were added three days a week to every mesocosm to produce a well-dispersed plume, which settled on the bed sediment as a thin accumulating layer. This study focused on the PCB congeners originating from field-collected bed sediment (referred to as “native bedded congeners” or “bedded PCBs”). However, of the six replicate mesocosms used for the controls and AC mix (a total of 18 mesocosms for E1, E2, and E3, Fig. S1†), three replicate mesocosms for each experimental set-up were used to introduce sediment spiked with PCB marker congeners 13, 54, and 173 (for a total of 9 mesocosms from E1, E2, and E3, Fig. S1†). PCB 54 and 173 were not detected in the bed sediment, but PCB 13 was present at lower concentrations in the bed sediment. The remaining nine mesocosms received clean sediment inputs without marker PCBs. The present study focused only on the uptake of native bedded PCB congeners. Uptake of the three ongoing input marker congeners have been examined previously.1,2,37
Polymeric sampling approaches
In one approach, LDPE was used as in situ (in the mesocosm) PSDs with performance reference compounds (PRCs) to predict PCB concentrations in polymer in equilibrium with the sediment1 or surface water.37 In another approach, silicone was used to coat the inner vertical walls of glass jars, which were filled with the study sediment and used as ex situ ESDs, where PCB equilibrium was confirmed between the sediment and the polymer after extraction and analysis. Equilibrium was confirmed by using three silicone coating thicknesses.1 A previous cross validation study demonstrated good agreement between these two polymeric sampling approaches of the surficial sediment on the basis of Cfree.1 For this investigation, the focus was on comparing the two polymeric sampling approaches to the lipid-normalized native bedded PCB concentrations in worms. Conversions from polymer to lipid utilized eqn (1): | 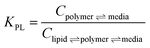 | (1) |
where KPL is the polymer-lipid partition coefficient for the specific PCB congener49,50 (Table S1†), Cpolymer⇌media is the concentration in the polymer at equilibrium (confirmed or calculated) with some media (e.g., sediment, water, etc.), and Clipid⇌polymer⇌media is the concentration in lipid at equilibrium with that media (often notation is simplified to Clipid⇌media). The conversion used in the present study is the same as the “third approach” applied in Burgess et al.,38 and is the approach of Jahnke et al.18 and Schäfer et al.20
Chemical analyses
The chemical analyses are described in detail in Schmidt et al.1 and Gidley et al.2,37 In brief, sediments were extracted by pressurized fluid extraction using hexane/acetone (USEPA SW-846 Method 3545A) and the extracts were treated with sulfuric acid following a modified EPA Method 3665A to remove interfering organic compounds. Tissues were extracted with hexane in a sonic bath overnight (modified EPA Method 3550) and lipids and other interfering compounds were removed by sulfuric acid based on a modified EPA method 3665. LDPE and silicone were extracted with hexane. Extracts were analyzed by dual column gas chromatography with electron capture detection (GC-ECD) following EPA method 8082A. A total of 133 PCB congeners were analyzed in the sediments, tissues and polymeric samplers, with 34 congeners being the focus of the present study (Table S1†). A microcolorimetric method51 was used to measure lipids from a separate aliquot of sample. The TOC of the sediment was measured using the Lloyd Kahn method.
Statistical analyses
JMP® 7.0 (SAS® Institute Inc.) was used to perform nonparametric Wilcoxon Signed-Rank tests. Here, the data was paired by congener and treatment, and a p-value less than 0.05 was considered statistically significant (α for 95% confidence). If the data were not paired, the nonparametric Wilcoxon test (also known as the Mann–Whitney U-statistic) was applied, where “Prob > |Z|” ≤ 0.05 was considered evidence that the means of the two treatments being compared were significantly different.
Results and discussion
Bed sediments had bulk-phase total PCB concentrations of approximately 30 mg kg−1 dry weight (excluding input marker congeners) and a TOC of 4.5%. Table 1 provides basic measurements of tissue mass and lipid content for the worms in the experimental treatments. There were no major differences in biomass across treatments, unlike other studies where the presence of AC may have affected worm nutrition and mass.39 Though no effect of AC on biomass was detected, AC may have influenced the activity of the worms and potentially reduced bioturbation (this processes has been documented by Koelmans and Jonker52). Sandworms of this general size range have been used in previous studies.25,27,29,53,54 The lipid content of the organisms was also similar to previous studies of sandworms.27,29,39,48,54,55 The worms were added to the mesocosms as adults, so significant growth was not expected during the 3 month experiments. Sandworms have been reported to grow slowly.56 Janssen and Beckingham57 reviewed the “secondary effects” of AC amendment on benthic organisms, such as changes in: growth, lipid content, behavior, and survival. Secondary effects on growth, lipid content, and survival were minimal in the present study. The present study did not include detailed monitoring of worm activity or bioturbation.
Table 1 Mean and standard deviation of sandworm (Alitta virens) individual mass and lipid content. Individual mass at experiment initiation (actual) and experiment end (calculated from total tissue and number of individuals from each mesocosm)a
|
Control |
AC mix |
Clean influx |
Spiked influx |
Clean influx |
Spiked influx |
Error = one standard deviation of the mean.
|
Whole mass (initial), g, n = 15 |
2.8 ± 0.8 |
3.1 ± 0.9 |
3.8 ± 0.8 |
4.4 ± 1.7 |
Whole mass calculated (end), g, n = 3 |
3.1 ± 0.1 |
3.3 ± 0.4 |
4.1 ± 0.2 |
4.1 ± 0.4 |
Lipid content, %, n = 3 |
1.8 ± 0.2 |
1.9 ± 0.3 |
1.8 ± 0.2 |
1.7 ± 0.2 |
Fig. 1 can be considered a ‘QTEA’ of PCBs in the surficial bedded sediment relative to the worms. Following eqn (1), equilibrium concentrations in polymer were converted to concentrations in lipid at equilibrium with the sediment (i.e., the thermodynamic potential for bioaccumulation, Clipid⇌sediment) by using congener specific polymer-lipid partition coefficients49,50 (Table S1†). The Clipid⇌sediment values then served as a basis to assess worm tissue concentrations measured and then lipid-normalized (i.e., “actual measured” in worm lipid by solvent extraction, Clipid(worms)). In-tissue polymer sampling17 was not conducted in the present study. The data was paired by each mesocosm, so three replicates appear as three separate data points in Fig. 1. A plot of PCB congeners measured in worm lipid and “predicted” in lipids based on ESDs, all versus log
KOW, is provided in the ESI (Fig. S2†).
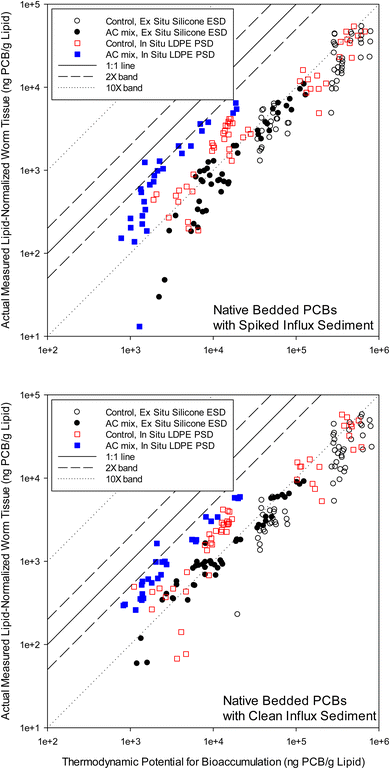 |
| Fig. 1 Measured lipid-normalized PCB concentrations in worm tissue (Clipid(worm), ng PCB/g lipid) versus the predicted thermodynamic potential for bioaccumulation (Clipid⇌sediment, ng PCB/g lipid) on a PCB congener basis. Circles show ex situ silicone equilibrium sampling device (ESD) results for Clipid⇌sediment, with hollow circles showing the control, and black filled circles showing the activated carbon (AC) mix treatment. Squares show in situ low density polyethylene (LDPE) passive sampling device (PSD) results for Clipid⇌sediment, with hollow red squares showing the control, and blue filled squares showing the AC mix treatment. The 1 : 1 line, 2× bands (1 : 1 ± a factor of 2), and 10× bands (1 : 1 ± a factor of 10) are shown. | |
The worms were exposed to PCBs primarily via the sediment and had sufficient time to reach steady-state with their environment.24,26,27,29,45,48 However, lipid normalized concentrations were generally below the equilibrium partitioning level, as indicated by data points below the 1
:
1 line in Fig. 1. The off-set ratio, of Clipid(worms) to Clipid⇌sediment, was calculated for each data point in Fig. 1, where Clipid⇌sediment is the thermodynamic potential for bioaccumulation (or ‘QTEA’) from the sediment determined using polymers. For ESDs the average (±standard deviation) ratio was 0.08 ± 0.04 for clean influx, and 0.07 ± 0.03 for spiked influx. For the PSDs, the ratios were 0.26 ± 0.13 and 0.21 ± 0.15 for the clean and spiked influx mesocosms, respectively. Both ratios are utilizing the same Clipid(worms), and therefore, the smaller ratios for ESDs indicates higher Clipid⇌sediment values relative to PSDs. This is consistent with the findings of Apell and Gschwend58 and Yan et al.,3 who observed ex situ Cfree measurements to be greater than in situ measurements. For most congeners with both ESDs and PSDs, the data were well below the 1
:
1 line (ratio < 1), indicating PCB concentrations in the worms below the thermodynamic potential for bioaccumulation from the surficial bed sediment. This assumes sufficient accuracy of the partition coefficients49,50 (Table S1†). Previous researchers have also found biota to be under-equilibrated relative to sediments.17–20 Recently, Burgess et al.38 performed a similar analysis at New Bedford Harbor under field conditions. In that study, bioaccumulation of PCBs by mussels (Mytilus edulis) deployed in the water column was compared to accumulation by co-deployed LDPE passive samplers. They observed the same type and magnitude off-set from the 1
:
1 line when actual bioaccumulation was compared with polymer predicted accumulation. The off-set presented in Jahnke et al.17,18 were also similar for fish, where polymer was equilibrated with sediment (as in Fig. 1). The degree of under-equilibration, in the present study, was also in the same range as other studies19,20 depending primarily on the species and system, but perhaps also on the polymer methods and approaches.
In the mesocosm systems of the present study, worms were exposed to influx sediment depositional layers (0.15 cm total theoretical accumulation in 90 days), which also included clean fish feed (unconsumed by fish) and fish feces. The introduction of clean feed and deposits likely reduced the concentration of PCBs in the surficial sediment and reduced the worm's exposure and tissue concentrations in terms of native bedded PCBs. Polymers and biota may have responded to this layering differently in terms of exposure and accumulation. However, relative to clams and fish (not the focus of the present study), the worms were expected to be the experimental species least impacted by the clean feed. The food was sinking through the water column, likely with time to be contaminated with PCBs before the worms consumed these deposits. Also, the worms likely fed on both bed sediment and input sediment. In contrast, caged fish fed exclusively in the water column, and clams fed primarily in the water column of the aquaria.
Risk assessments at contaminated sites are often driven by fish and shellfish data because of concerns with human consumption of seafood. In the field, many fish species will have a sediment linkage, either food web related or by direct sediment interaction.6,20,59 Supporting this, Schmidt and Burgess23 found ex situ sediment ESDs predicted fish and shellfish bioaccumulation with greater strength and accuracy than in situ water column PSDs. Among the species in the mesocosms, the worm tissue concentrations may be the most similar to fish tissue concentrations at field sites, when considering the addition of clean feed. The fish in the mesocosms were confined to the surface water in cages (to reduce sediment resuspension by swimming activity) – disrupting the sediment linkage. Applying clean feed to the mesocosms is a departure from field conditions.35 Compared to biota in these mesocosm experiments, the resident biota in the field are expected to consume material fully equilibrated with PCBs from their environment. Bridges et al.35 applied Cfree values and a deconvoluted food web model60 to these mesocosms. They found that the food web model could represent exposure conditions in these experiments assuming the worm diet consisted of 50% sediment and 50% clean feed, and worm ventilation consisted of 100% overlying water. The strong influence of the surface water is consistent with worms irrigating their burrows.39,45
Polymer samplers measured the depositional layer, but this would be in proportion to the depth of sediment measured (i.e., spatial vertical scale sampled) by the polymers. The depth of the depositional layers was theoretically 0.15 cm accumulation in 90 days, or only 5–8% of the sampler depth (sampler depth was approximately the top 2–3 cm). The worms were exposed to depositional layers and sediment at depth, but the proportions could have been different than the vertical scale sampled by the polymers. In addition, the worms have a surface water exposure component. Preferential feeding by the worms around the depositional layer is presumed to have occurred, as it would have been enriched in feed and feces from the fish. Sandworms may metabolize some PCBs, which does not occur in polymers, but the PCBs that metabolize are generally known.21,25–27,40,54,61 There were no outliers in Fig. 1 that could be explained by PCB metabolism in the worms and not in the polymer. Furthermore, the interaction of physical/chemical processes in the sediment versus metabolic alteration in the worms may never have been properly delineated, previously, in systems including sediment.25,26
Fig. 2 presents decreases in Clipid(worms) (Y-axis) versus decreases in Clipid⇌polymer⇌sediment (X-axis, from Cpolymer⇌sediment) in AC treated systems relative to the control system without AC, following eqn (2):
|  | (2) |
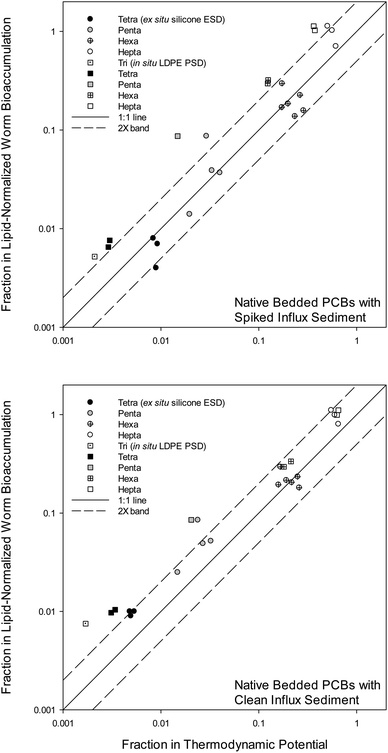 |
| Fig. 2 Fraction remaining in lipid-normalized worm bioaccumulation by the addition of activated carbon (AC) (Clipid(worm),AC mix/Clipid(worm),contol) versus fraction remaining in the “thermodynamic potential for bioaccumulation” from sediment by the addition of AC (Clipid⇌sediment,AC mix/Clipid⇌sediment,control). Circles show ex situ silicone equilibrium sampling device (ESD) results for Clipid⇌sediment separated by PCB chlorination/homolog. Squares show in situ low density polyethylene (LDPE) passive sampling device (PSD) results for Clipid⇌sediment, also separated by PCB chlorination. The 1 : 1 line and 2× bands (1 : 1 ± a factor of 2) are shown. | |
The “fraction remaining” is similar to the “fraction of initial condition” described by Grundy et al.16 Overall, there was good agreement between decline in thermodynamic potential (Clipid⇌polymer⇌sediment) and decline in actual bioaccumulation (Clipid(worms)). In terms of total PCBs analyzed, the AC provided 90% reductions in worms, 93% reductions in silicone ESDs, and 97% reductions in LDPE PSDs (not all data shown). Of the congeners presented in Fig. 2, three replicates for each condition were averaged before the fraction remaining was calculated. The fraction of PCB bioaccumulation remaining in the worms resulting from the presence of AC ranged from 1.1 (10% increase) to 0.004 (99.6% reduction) depending on the chlorination of the PCB congeners. The fraction of PCBs remaining in polymers caused by the AC ranged from 0.65 (35% reduction) to zero (non-detect, 100% reduction). Tri-chlorinated congeners were non-detect in the AC mix with the additional mixing of the ESDs, so tri-chlorinated congeners were only shown for the PSDs. In Fig. 2, the differences in the remedy effectiveness based on KOW, reflected by the PCB homologs, has been previously observed and reported for the bioaccumulation data of all mesocosm species.2 In the current study, highly chlorinated PCBs showed little-to-no exposure reductions.
For the tri-, tetra-, and penta-chlorinated congeners in the clean influx mesocosms, both polymeric sampling methods showed Clipid⇌sediment reductions greater than actual determined reductions in Clipid(worms) (Fig. 2). It is possible that this is primarily related to the introduction of clean feed (as described above). The signal (i.e., change in PCB uptake due to the addition of AC) from the worm tissue was dampened (or reduced) by the clean feed relative to the signal in the polymers. In other words, it is expected that polymers do not respond as much to the clean feed as do the biota.
Sediment ingesting organisms are expected to align better with ex situ passive sampling than in situ passive sampling,3 and the results of the present study appear consistent with this. There is a minor to moderate difference between PSD and ESD derived measurements, which can be seen in both Fig. 1 and 2. ESD measurements seem generally closer to the 1
:
1 line in Fig. 2, thus having higher predictive value of the bioaccumulation response to the remedy, compared to PSD measurements. In Fig. 1 this is seen as better alignment of the ESD data, for both the AC mix and the control, relative to PSDs. For the spiked influx mesocosms (Fig. 2), this difference is significant (p = 0.02, paired test; prob > |Z| = 0.0005) between the PSDs and the ESDs across all PCB homologs. This result was not necessarily expected at the start of this study, as ex situ sampling involves two weeks of additional mixing, which advances the sediment-to-AC mass transfer of PCBs. The 1 month pre-mixing at the start of the mesocosm studies helped the ESDs in this respect.
Additional data is included in Fig. 3 (not shown in Fig. 1 and 2), which shows: fractions of the thermodynamic level from PSDs used to measure the sediment (“LDPE strips”), PSDs used to measure the surface water (“LDPE discs”), and lipid-normalized worm bioaccumulation; all relative to the ESDs used to measure the sediment. The fraction of the thermodynamic potential as defined by silicone ESDs was determined following eqn (3):
|  | (3) |
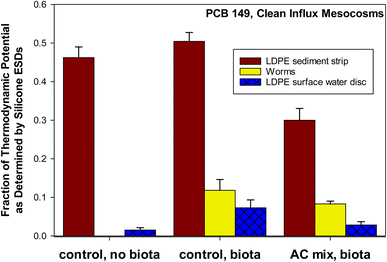 |
| Fig. 3 Fraction of thermodynamic potential as defined by silicone equilibrium sampling devices (ESDs). Silicone ESDs sampled surficial bed sediment. In situ low density polyethylene (LDPE) strips sampled surficial bed sediment (0 to 90 days). In situ LDPE discs sampled surface water (overlying water) from 45 to 90 days. Controls (with and without biota) and activated carbon (AC) mix treatments are shown. A mean LDPE-lipid partition coefficient of 0.0938 for PCBs was used to convert concentrations of PCB 149 in LDPE to a lipid basis.50 A congener specific silicone-lipid partition coefficient of 0.0945 was used to convert concentrations of PCB 149 in silicone to a lipid basis.49 Error bars show one standard deviation of the mean (n = 3). | |
Additionally, a set of control mesocosms containing no biota was included in Fig. 3. PCB 149 was selected because it was the only non-coeluting peak on chromatograms that was consistently detected in all replicates above detection limits in the surface water of the AC mix treatment. PCB 149, a hexa-chlorinated congener with a log
KOW of 6.67,62 can be considered highly hydrophobic. In order to emphasize the relationship within each mesocosm, rather than the average across mesocosms (as in Fig. 2), the fraction of the ESD measurement was taken within each mesocosm (as in Fig. 1) prior to averaging across three replicate mesocosms receiving clean sediment influx (Fig. 3). PCB 149 shows that for clean influx mesocosms, the worms were at a thermodynamic level closer to the surface water (LDPE disc) than the sediment (LDPE strip) for both the AC mix and control treatments. The sediment (measured by polymer) was at a higher thermodynamic level than the worms and surface water. PCB 149 was the congener in organisms closest to the thermodynamic potential for bioaccumulation from the sediment (in worms at 12.4% of the potential). In the control (no AC), other congeners in the worms were at a level even more comparable to the surface water. Field studies have found polymers equilibrated with surface waters to be at higher thermodynamic levels than biota.19,38 The spiked influx mesocosms may agree more with field studies in this respect, but this data was not shown (Fig. S3†), as PCB 149 was below detection in the spiked influx AC mix treatment by the surface water PSDs (LDPE discs). In the clean influx mesocosms (Fig. 3), the surface water levels were close to detection limits in the AC mix. If the fish feed (flakes) and clam feed (algae) had been pre-equilibrated with the system (contaminated), the worms may have been at a level closer to the sediment.
In Fig. 3, it can be seen that the relative thermodynamic levels between sediment, worms, and surface water are maintained in the control and AC mix treatments. In other words, the worms remain at about 5 to 10% of the thermodynamic potential of the sediment regardless of whether or not the sediment contains AC. Rather than the traditional BSAF model, Sormunen et al.63 discuss a “refined BSAF” – a term utilized here (eqn (4)), where:
|  | (4) |
The ratio of Clipid(bio) to Clipid⇌sediment has been utilized previously.18–20 The “activity ratio”,20 “partitioning status”64 or “refined BSAF” between biota and sediment would still vary based on the species-specific traits within a system and perhaps KOW, but not vary based on partitioning to different types of organic matter30,33 or from AC amendment and associated aging. Fig. S2c† shows the refined BSAFs did not vary much based on KOW in the present study, likely due to the ingestion of sediment by the worms. Diepens et al.30 found traditional BSAFs for similar congeners to range between 7.4 and 19.2 for worms exposed to spiked sediment. Applying these refined BSAFs would not likely narrow this range of BSAFs much, relative to their overall magnitude (these researchers were not investigating different types of organic carbon). However, the refined BSAFs may have removed the confounding factors of organic matter quality changes and sediment-PCB aging over time.
Conclusions
These mesocosm studies were complex relative to routine bioaccumulation tests, but successfully isolated some important processes occurring in the experimental system, which could be representative of processes occurring in the field. This work contributes to a growing number of studies on the use of polymeric samplers to monitor sediment remedy performance (particularly during AC amendment). In the present study, a ‘QTEA’ was accomplished, showing that complex processes affecting bioaccumulation in worms might be monitored relatively simply by polymeric sampling. Specifically, for remedy effectiveness monitoring, this could be accomplished by measuring the concentration of HOCs in a polymer at equilibrium with the surficial sediment.
In practice, monitoring the performance of remedies in the field should utilize a consistent and predictive form of polymeric sampling of the sediment. Previous researchers have concluded that measuring Cfree in the interstitial water,15,58 or polymer concentrations from surface water37,38 would be best for monitoring remedies. Researchers have also found the in situ interstitial Cfree (from PSDs) may be most useful for understanding sediment-to-water column fluxes.65 PSDs may be more responsive to conditions in the surficial sediment than the worms and ex situ ESDs, but the latter two tracked better through the AC remedy. The present study found that ex situ sampling of the surficial sediment may be most useful for tracking the performance of an AC remedy applied to the sediment with ongoing influx. Apell and Gschwend58 and Yan et al.3 recognized that ex situ Cfree determination might be preferred to in situ Cfree, if ex situ shows the highest possible interstitial water concentrations. This was not obvious to the authors of the present study, with regard to AC amended sediments, as ex situ methods involve additional mixing. Surficial sediment is often dynamic, being resuspended and redeposited. It is likely that sampling suspended particulate matter,64 or dredged material in a maintenance dredging operation, could yield similar useful measurements depending on the questions being asked.
Ex situ equilibrium sampling was, in the present study, conducted using silicone coated jars with multiple coating thickness. The ultrathin silicone coatings provided fast equilibration of PCBs in sediment interstitial water, and the multiple silicone coating thicknesses were applied to confirm equilibrium and the absence of surface sorption artifacts. Commercialization of the coated jars would promote uniformity of samplers and incentivize the adoption of this method.66 Jonker et al.15 recently provided practical guidance on other ex situ equilibrium sampling techniques that are simpler to operate and can yield similar concentrations in polymer. Overall, ex situ equilibrium sampling of surficial sediment (or dredged material during maintenance dredging activities) will be a more accurate alternative to conventional, organic carbon normalized, sediment measurements used in the BSAF model17,18,20–22 and existing risk assessment and monitoring frameworks.
Conflicts of interest
The authors declare no conflict of interest associated with this study. Citation of trade names utilized in this study does not constitute an official endorsement or approval of the use of such commercial products. Any opinions, findings, conclusions, or recommendations expressed in this article are those of the authors, and not necessarily the views of the US Army Corps of Engineers, or the US Environmental Protection Agency.
Acknowledgements
We gratefully acknowledge team members: Alice P. Wang, Loretta A. Fernandez, Alan J. Kennedy, Nicolas L. Melby, Allyson H. Wooley, Natalie S. Rogers, Carlos E. Ruiz, Todd S. Bridges and Upal Ghosh for their contributions. This work was funded by the US Strategic Environmental Research and Development Program (SERDP, 14 ER03-035/ER-2431) and the US Army Corps of Engineers' Dredging Operations and Environmental Research program (DOER, project RT 23-10).
References
- S. N. Schmidt, A. P. Wang, P. T. Gidley, A. H. Wooley, G. R. Lotufo, R. M. Burgess, U. Ghosh, L. A. Fernandez and P. Mayer, Cross validation of two partitioning-based sampling approaches in mesocosms containing PCB contaminated field sediment, biota, and activated carbon amendment, Environ. Sci. Technol., 2017, 51, 9996–10004 CrossRef CAS PubMed.
- P. T. Gidley, A. J. Kennedy, G. R. Lotufo, A. H. Wooley, N. L. Melby, U. Ghosh, R. M. Burgess, P. Mayer, L. A. Fernandez, S. N. Schmidt, A. P. Wang, T. S. Bridges and C. E. Ruiz, Bioaccumulation in functionally different species: ongoing input of PCBs with sediment deposition to activated carbon remediated bed sediments, Environ. Toxicol. Chem., 2019, 38, 2326–2336 CrossRef CAS PubMed.
- S. Yan, M. Rakowska, X. Shen, T. Himmer, C. Irvine, R. Zajac-Fay, J. Eby, D. Janda, S. Ohannessian and D. D. Reible, Bioavailability assessment in activated carbon treated coastal sediment with in situ and ex situ porewater measurements, Water Res., 2020, 185, 116259 CrossRef CAS PubMed.
- M. T. Schaanning, B. Beylich, J. S. Gunnarsson and E. Eek, Long-term effects of thin layer capping in the Grenland fjords, Norway: Reduced uptake of dioxins in passive samplers and sediment-dwelling organisms, Chemosphere, 2021, 264, 128544 CrossRef CAS PubMed.
- A. P. Wang, J. Conder, B. Chadwick and G. Rosen, Long-Term Monitoring of an In Situ Activated Carbon Treatment to Reduce Polychlorinated Biphenyl Availability in an Active Harbor, Environ. Toxicol. Chem., 2022, 41, 1568–1574 CrossRef CAS PubMed.
- L. J. Thibodeaux and V. J. Bierman, The bioturbation-driven chemical release process, Environ. Sci. Technol., 2003, 37, 252A–258A CrossRef CAS PubMed.
- D. P. Weston, W. M. Jarman, G. Cabana, C. E. Bacon and L. A. Jacobson, An evaluation of the success of dredging as remediation at a DDT-contaminated site in San Francisco Bay, California, USA, Environ. Toxicol. Chem., 2002, 21, 2216–2224 CAS.
-
S. C. Nadeau and M. M. Skaggs Jr, Analysis of recontamination of completed sediment remedial projects, in Proceedings of the Fourth International Conference on Remediation of Contaminated Sediments, Savannah, GA, USA, 2007 Search PubMed.
- L. A. Rodenburg, S. Du, B. Xiao and D. E. Fennell, Source apportionment of polychlorinated biphenyls in the New York/New Jersey Harbor, Chemosphere, 2011, 83, 792–798 CrossRef CAS PubMed.
- T. P. Needham and U. Ghosh, Four decades since the ban, old urban wastewater treatment plant remains a dominant source of PCBs to the environment, Environ. Pollut., 2019, 246, 390–397 CrossRef CAS PubMed.
- G. Schertzinger, F. Itzel, J. Kerstein, J. Tuerk, T. C. Schmidt and B. Sures, Accumulation pattern and possible adverse effects of organic pollutants in sediments downstream of combined sewer overflows, Sci. Total Environ., 2019, 675, 295–304 CrossRef CAS PubMed.
- N. J. Lombard, M. Bokare, R. Harrison, L. Yonkos, A. Pinkney, D. Murali and U. Ghosh, Codeployment of Passive Samplers and Mussels Reveals Major Source of Ongoing PCB Inputs to the Anacostia River in Washington, DC, Environ. Sci. Technol., 2023, 57, 1320–1331 CrossRef CAS PubMed.
- T. Hussain, D. Athanasiou, B. Rao, M. Bejar, M. Rakowska, I. Drygiannaki, D. B. Chadwick, M. A. Colvin, N. T. Hayman, G. H. Rosen, M. Otto, B. Streets, R. Pitt and D. D. Reible, Sediment recontamination potential and biological impacts of hydrophobic organics from stormwater in a mixed-use watershed, Sci. Total Environ., 2024, 906, 167444 CrossRef CAS PubMed.
- K. Booij, C. D. Robinson, R. M. Burgess, P. Mayer, C. A. Roberts, L. Ahrens, I. J. Allan, J. Brant, L. Jones, U. R. Kraus and M. M. Larsen, Passive sampling in regulatory chemical monitoring of nonpolar organic compounds in the aquatic environment, Environ. Sci. Technol., 2016, 50, 3–17 CrossRef CAS PubMed.
- M. T. Jonker, R. M. Burgess, U. Ghosh, P. M. Gschwend, S. E. Hale, R. Lohmann, M. J. Lydy, K. A. Maruya, D. Reible and F. Smedes,
Ex situ determination of freely dissolved concentrations of hydrophobic organic chemicals in sediments and soils: basis for interpreting toxicity and assessing bioavailability, risks and remediation necessity, Nat. Protoc., 2020, 15, 1800–1828 CrossRef CAS PubMed.
- J. S. Grundy, M. K. Lambert and R. M. Burgess, Passive Sampling-Based versus Conventional-Based Metrics for Evaluating Remediation Efficacy at Contaminated Sediment Sites: A Review, Environ. Sci. Technol., 2023, 57, 10151–10172 CrossRef CAS PubMed.
- A. Jahnke, P. Mayer, M. S. McLachlan, H. Wickström, D. Gilbert and M. MacLeod, Silicone passive equilibrium samplers as ‘chemometers’ in eels and sediments of a Swedish lake, Environ. Sci.: Processes Impacts, 2014, 16, 464–472 RSC.
- A. Jahnke, M. MacLeod, H. Wickström and P. Mayer, Equilibrium sampling to determine the thermodynamic potential for bioaccumulation of persistent organic pollutants from sediment, Environ. Sci. Technol., 2014, 48, 11352–11359 CrossRef CAS PubMed.
- K. Mäenpää, M. T. Leppänen, K. Figueiredo, P. Mayer, D. Gilbert, A. Jahnke, C. Gil-Allué, J. Akkonen, I. Nybom and S. Herve, Fate of polychlorinated biphenyls in a contaminated lake ecosystem: combining equilibrium passive sampling of sediment and water with total concentration measurements of biota, Environ. Toxicol. Chem., 2015, 34, 2463–2474 CrossRef PubMed.
- S. Schäfer, C. Antoni, C. Möhlenkamp, E. Claus, G. Reifferscheid, P. Heininger and P. Mayer, Equilibrium sampling of polychlorinated biphenyls in River Elbe sediments–linking bioaccumulation in fish to sediment contamination, Chemosphere, 2015, 138, 856–862 CrossRef PubMed.
- C. L. Friedman, R. M. Burgess, M. M. Perron, M. G. Cantwell, K. T. Ho and R. Lohmann, Comparing polychaete and polyethylene uptake to assess sediment resuspension effects on PCB bioavailability, Environ. Sci. Technol., 2009, 43, 2865–2870 CrossRef CAS PubMed.
- A. S. Joyce, L. M. Portis, A. N. Parks and R. M. Burgess, Evaluating the relationship between equilibrium passive sampler uptake and aquatic organism bioaccumulation, Environ. Sci. Technol., 2016, 50, 11437–11451 CrossRef CAS PubMed.
- S. N. Schmidt and R. M. Burgess, Evaluating polymeric sampling as a tool for predicting the bioaccumulation of polychlorinated biphenyls by fish and shellfish, Environ. Sci. Technol., 2020, 54, 9729–9741 CrossRef CAS PubMed.
-
R. J. Pruell, N. I. Rubinstein, B. K. Taplin, J. A. LiVolsi and C. B. Norwood, 2,3,7,8-TCDD, 2,3,7,8-TCDF and PCBs in marine sediments and biota: laboratory and field studies, Final report (no. PB-90-266735/XAB; EPA-600/8-90/068), Environmental Protection Agency, Narragansett, RI (USA), Environmental Research Lab, 1990 Search PubMed.
- R. J. Pruell, N. I. Rubinstein, B. K. Taplin, J. A. LiVolsi and R. D. Bowen, Accumulation of polychlorinated organic contaminants from sediment by three benthic marine species, Arch. Environ. Contam. Toxicol., 1993, 24, 290–297 CrossRef CAS.
- R. J. Pruell, B. K. Taplin, D. G. McGovern, R. McKinney and S. B. Norton, Organic contaminant distributions in sediments, polychaetes (Nereis virens) and American lobster (Homarus americanus) from a laboratory food chain experiment, Mar. Environ. Res., 2000, 49, 19–36 CrossRef CAS PubMed.
-
A. J. Kennedy, G. R. Lotufo, J. A. Steevens and T. S. Bridges, Determining Steady-State Tissue Residues for Invertebrates in Contaminated Sediment, ERDC/EL TR-10-2, U.S. Army Engineer Research and Development Center, Vicksburg, MS, USA, 2010 Search PubMed.
- E. R. Bennett, J. A. Steevens, G. R. Lotufo, G. Paterson and K. G. Drouillard, Novel control and steady-state correction method for standard 28-day bioaccumulation tests using Nereis virens, Environ. Toxicol. Chem., 2011, 30, 1366–1375 CrossRef CAS PubMed.
- S. L. Klosterhaus, E. Dreis and J. E. Baker, Bioaccumulation kinetics of polybrominated diphenyl ethers from estuarine sediments to the marine polychaete, Nereis virens, Environ. Toxicol. Chem., 2011, 30, 1204–1212 CrossRef CAS PubMed.
- N. J. Diepens, M. J. Van den Heuvel-Greve and A. A. Koelmans, Modeling of bioaccumulation in marine benthic invertebrates using a multispecies experimental approach, Environ. Sci. Technol., 2015, 49, 13575–13585 CrossRef CAS PubMed.
- G. R. Lotufo, J. M. Biedenbach, J. D. Farrar, M. K. Chanov, B. W. Hester, C. R. Warbritton, J. A. Steevens, J. M. Netchaev, A. J. Bednar and D. W. Moore, Interlaboratory Comparison of Three Sediment Bioaccumulation Tests, Environ. Toxicol. Chem., 2022, 41, 1260–1275 CrossRef CAS PubMed.
- G. Cornelissen and Ö. Gustafsson, Prediction of large variation in biota to sediment accumulation factors due to concentration-dependent black carbon adsorption of planar hydrophobic organic compounds, Environ. Toxicol. Chem., 2005, 24, 495–498 CrossRef CAS PubMed.
- G. Cornelissen, Ö. Gustafsson, T. D. Bucheli, M. T. Jonker, A. A. Koelmans and P. C. Van Noort, Extensive sorption of organic compounds to black carbon, coal, and kerogen in sediments and soils: mechanisms and consequences for distribution, bioaccumulation, and biodegradation, Environ. Sci. Technol., 2005, 39, 6881–6895 CrossRef CAS PubMed.
-
A. P. Wang, Application of low density polyethylene passive sampling to assess the efficacy of contaminated sediment remedies in the presence of on-going polychlorinated biphenyl inputs, PhD thesis, Northeastern University, Boston, MA, USA, 2019, available from: https://repository.library.northeastern.edu/files/neu:m044hf31v/fulltext.pdf.
-
T. S. Bridges, P. T. Gidley, G. R. Lotufo, A. J. Kennedy, C. E. Ruiz, N. L. Melby, M. L. Ballentine, A. Wooley, C. Laber, U. Ghosh, R. M. Burgess, L. A. Fernandez, A. Wang, P. Mayer and S. Schmidt, Quantitative Thermodynamic Exposure Assessment (QTEA) Supporting Resilient Contaminated Sediment Site Restoration; Final Report for ER-2431, SERDP, Alexandria, VA(USA), 2022 Search PubMed.
- W. S. Bosworth and L. J. Thibodeaux, Bioturbation: a facilitator of contaminant transport in bed sediment, Environ. Prog., 1990, 9, 211–217 CrossRef CAS.
- P. T. Gidley, G. R. Lotufo, A. J. Kennedy, N. L. Melby, A. H. Wooley, C. H. Laber, R. M. Burgess, C. E. Ruiz and T. S. Bridges, Effect of Activated Carbon in Thin Sand Caps Challenged with Ongoing PCB Inputs from Sediment Deposition: PCB Uptake in Clams (Mercenaria mercenaria) and Passive Samplers, Arch. Environ. Contam. Toxicol., 2022, 82, 95–104 CrossRef CAS PubMed.
- R. M. Burgess, M. G. Cantwell, Z. Dong, J. S. Grundy and A. S. Joyce, Comparing Equilibrium Concentrations of Polychlorinated Biphenyls Based on Passive Sampling and Bioaccumulation in Water Column Deployments, Environ. Toxicol. Chem., 2023, 42, 317–332 CrossRef CAS PubMed.
- S. Josefsson, M. Schaanning, G. S. Samuelsson, J. S. Gunnarsson, I. Olofsson, E. Eek and K. Wiberg, Capping Efficiency of Various Carbonaceous and Mineral Materials for In Situ Remediation of Polychlorinated Dibenzo-p-dioxin and Dibenzofuran Contaminated Marine Sediments: Sediment-to-Water Fluxes and Bioaccumulation in Boxcosm Tests, Environ. Sci. Technol., 2012, 46, 3343–3351 CrossRef CAS PubMed.
-
W. H. Wilson Jr and R. E. Ruff, Species Profiles: Life Histories and Environmental Requirements of Coastal Fisheries and Invertebrates (North Atlantic): Sandworm and Bloodworm, U.S. Fish and Wildlife Service Biological Report 82(11.80), U.S. Army Corps of Engineers, Washington, DC, TR EL-82-4, 1988 Search PubMed.
- L. Pischedda, J. C. Poggiale, P. Cuny and F. Gilbert, Imaging oxygen distribution in marine sediments. The importance of bioturbation and sediment heterogeneity, Acta Biotheor., 2008, 56, 123–135 CrossRef CAS PubMed.
- G. Y. Miron and G. L. Desrosiers, Distributions and population structures of two intertidal estuarine polychaetes in the lower St. Lawrence Estuary, with special reference to environmental factors, Mar. Biol., 1990, 105, 297–306 CrossRef.
- E. Kristensen, M. Hjorth Jensen and R. C. Aller, Direct measurement of dissolved inorganic nitrogen exchange and denitrification in individual polychaete (Nereis virens) burrows, J. Mar. Res., 1991, 49, 355–377 CrossRef CAS.
-
K. T. Du Clos, Polychaete Burrowing Behavior in Sand and Mud, MS thesis, MS thesis, The University of Maine, Orono, ME, USA, 2012, available from: https://digitalcommons.library.umaine.edu/etd/1872.
- N. I. Rubinstein, E. Lores and N. R. Gregory, Accumulation of PCBs, mercury and cadmium by Nereis virens, Mercenaria mercenaria and Palaemonetes pugio from contaminated harbor sediments, Aquat. Toxicol., 1983, 3, 249–260 CrossRef CAS.
- A. M. Nielsen, N. T. Eriksen, J. L. Iversen and H. U. Riisgård, Feeding, growth and respiration in the polychaetes Nereis diversicolor (facultative filter-feeder) and N. virens (omnivorous)-a comparative study, Mar. Ecol.: Prog. Ser., 1995, 125, 149–158 CrossRef.
- N. I. Rubinstein, R. J. Pruell, B. K. Taplin, J. A. LiVolsi and C. B. Norwood, Bioavailability of 2,3,7,8-TCDD, 2,3,7,8-TCDF and PCBs to marine benthos from Passaic River sediments, Chemosphere, 1990, 20, 1097–1102 CrossRef CAS.
- D. W. McLeese, C. D. Metcalfe and D. S. Pezzack, Uptake of PCBs from sediment by Nereis virens and Crangon septemspinosa, Arch. Environ. Contam. Toxicol., 1980, 9, 507–518 CrossRef CAS PubMed.
- D. Gilbert, G. Witt, F. Smedes and P. Mayer, Polymers as Reference Partitioning Phase: Polymer Calibration for an Analytically Operational Approach To Quantify Multimedia Phase Partitioning, Anal. Chem., 2016, 88, 5818–5826 CrossRef CAS PubMed.
- F. Smedes, T. P. Rusina, H. Beeltje and P. Mayer, Partitioning of hydrophobic organic contaminants between polymer and lipids for two silicones and low density polyethylene, Chemosphere, 2017, 186, 948–957 CrossRef CAS PubMed.
- E. Van Handel, Rapid determination of total lipids in mosquitoes, J. Am. Mosq. Control Assoc., 1985, 1, 302–304 CAS.
- A. A. Koelmans and M. T. Jonker, Effects of black carbon on bioturbation-induced benthic fluxes of polychlorinated biphenyls, Chemosphere, 2011, 84, 1150–1157 CrossRef CAS PubMed.
- H. Goerke, Temperature-dependent elimination of 2,4,6,2′,4′-pentachloro[U-14C]biphenyl in Nereis virens (Polychaeta), Arch. Environ. Contam. Toxicol., 1986, 13, 347–355 CrossRef.
- H. Goerke and W. Ernst, Elimination of pentachlorobiphenyls by Nereis virens (Polychaeta) in the laboratory and the marine environment, Chem. Ecol., 1986, 2, 263–285 CrossRef CAS.
- H. Goerke and W. Ernst, Fate of 14C-labelled di-, tri- and pentachlorobiphenyl in the marine annelid Nereis virens I. Accumulation and elimination after oral administration, Chemosphere, 1977, 6, 551–558 CrossRef CAS.
- V. E. Herwati, T. Elfitasari, N. Rismaningsih, P. H. Riyadi, W. Tarangkoon, O. Radjasa and S. Windarto, Analysis of growth and nutritional quality of sea worms (Nereis virens) as a mass cultured natural feed on different substrate media thicknesses, Biodiversitas, 2021, 22, 3299–3305 Search PubMed.
- E. M. L. Janssen and B. A. Beckingham, Biological responses to activated carbon amendments in sediment remediation, Environ. Sci. Technol., 2013, 47, 7595–7607 CrossRef CAS PubMed.
- J. N. Apell and P. M. Gschwend,
In situ passive sampling of sediments in the Lower Duwamish Waterway Superfund site: Replicability, comparison with ex situ measurements, and use of data, Environ. Pollut., 2016, 218, 95–101 CrossRef CAS PubMed.
-
B. Huser and P. Bartels, Feeding ecology of carp, in Biology and Ecology of Carp, ed. C. Pietsch and P. Hirsch, CRC Press, Boca Raton, FL, USA, 2015, pp. 217–243 Search PubMed.
- U. Ghosh, M. Bokare and F. A. P. C. Gobas, Deconvoluting Thermodynamics from Biology in the Aquatic Food Web Model, Environ. Toxicol. Chem., 2021, 40, 2145–2155 CrossRef CAS PubMed.
- W. Ernst, H. Goerke and K. Weber, Fate of 14C-labelled di-, tri- and pentachlorobiphenyl in the marine annelid Nereis virens II. Degradation and faecal elimination, Chemosphere, 1977, 6, 559–568 CrossRef CAS.
- D. W. Hawker and D. W. Connell, Octanol-Water Partition Coefficients of Polychlorinated Biphenyl Congeners, Environ. Sci. Technol., 1988, 22, 382–387 CrossRef CAS.
- A. J. Sormunen, M. T. Leppänen and J. V. K. Kukkonen, Influence of sediment ingestion and exposure concentration on the bioavailable fraction of sediment-associated tetrachlorobiphenyl in oligochaetes, Environ. Toxicol. Chem., 2008, 27, 854–863 CrossRef CAS PubMed.
- T. Wernicke, S. Abel, B. I. Escher, J. Koschorreck, H. Rüdel and A. Jahnke, Equilibrium sampling of suspended particulate matter as a universal proxy for fish and mussel monitoring, Ecotox. Environ. Safe., 2022, 232, 113285 CrossRef CAS PubMed.
- J. N. Apell, D. H. Shull, A. M. Hoyt and P. M. Gschwend, Investigating the effect of bioirrigation on in situ porewater concentrations and fluxes of polychlorinated biphenyls using passive samplers, Environ. Sci. Technol., 2018, 52, 4565–4573 CrossRef CAS PubMed.
- X. Cui, P. Mayer and J. Gan, Methods to assess bioavailability of hydrophobic organic contaminants: principles, operations, and limitations, Environ. Pollut., 2013, 172, 223–234 CrossRef CAS PubMed.
|
This journal is © The Royal Society of Chemistry 2024 |