DOI:
10.1039/D4EE01252F
(Communication)
Energy Environ. Sci., 2024,
17, 6204-6214
Aeroelectrolyte for atmospheric open electrochemical cells†
Received
19th March 2024
, Accepted 16th May 2024
First published on 17th May 2024
Abstract
Expediting electrochemical reactions that involve gaseous components is crucial for the development of diverse energy and environmental applications (batteries, electrochemical conversion, fuel cells, and gas sensors). Unfortunately, the intrinsically closed structure of conventional electrochemical cell systems, in which a liquid (or solid) electrolyte exists between the electrodes, does not allow the facile and direct diffusion of gaseous reactants or products to/from the electrode surface. Our design, an electrochemical cell system with an open-structured form factor, involves an “aeroelectrolyte” that floats in the air and directly approaches the electrode surfaces. The electrochemical system contains a nonwoven nanofiber salt bridge to ensure continuous and stable electrochemical reactions in the aeroelectrolyte-based cells. We confirmed the feasibility of our design in a modified Daniell cell by varying the factors that affect the aeroelectrolyte and electrode components. Our open-structure electrochemical system with the aeroelectrolyte enables the realization of ideal three-phase boundaries for atmospheric electrochemical reactions.
Broader context
Accelerating electrochemical reactions involving gaseous components is vital for advancing various energy and environmental applications such as batteries, electrochemical conversion, fuel cells, and gas sensors. Unfortunately, conventional electrochemical cell systems, characterized by their closed structure with a liquid or solid electrolyte between electrodes, hinder the easy and direct diffusion of gaseous reactants or products to and from the electrode surface. Our innovative design addresses this limitation by introducing an open-structured electrochemical cell system featuring an “aeroelectrolyte” that floats in the air and directly engages with the electrode surfaces. To ensure continuous and stable electrochemical reactions in these aeroelectrolyte-based cells, we incorporate a nonwoven nanofiber salt bridge. Validating the feasibility of our approach, we conducted experiments on a modified Daniell cell, adjusting factors influencing both the aeroelectrolyte and electrode components. Our open-structure electrochemical system with the aeroelectrolyte facilitates the establishment of ideal three-phase boundaries for atmospheric electrochemical reactions.
|
1. Introduction
Electrochemical cells are utilized in modern energy and environmental applications owing to their reaction versatility, simplicity, two-way (spontaneous and non-spontaneous) operation, and current/voltage-based controllable reaction characteristics.1–5 For decades, studies on diverse electrochemical devices (batteries, electrochemical conversion, fuel cells, gas sensors, etc.) have mainly focused on regulating the type and composition of cell components comprising solid electrodes (cathodes and anodes) and a liquid electrolyte.6–10 Since then, electrochemistry has evolved to adopt advanced electrochemical cell mechanisms that utilize atmospheric agents as reactants or products for gas-related energy and environmental applications, such as generating useful gases (e.g., water electrolysis and CH4 oxidation) and controlling gaseous pollutants (e.g., the reduction of CO2 or N2).9,11–16 However, to fundamentally adapt to changes in the state of matter and respond to the increasing randomness of gaseous reactants, we need to develop a customized cell design for atmospheric electrochemical reaction.17–20
Conventional electrochemical conversion of gaseous reactants requires these reactants, which are randomly scattered in the atmosphere, to dissolve in or penetrate the liquid electrolyte and then adhere to the electrode/electrolyte interface.21,22 Moreover, the diffusion rate and solubility of gaseous reactants in liquid electrolytes are typically poor, and are responsible for sluggish mass transport during the electrochemical reaction.21,23 Traditionally, coercive gas purging has been used to enhance the dissolution of gas-phase reactants. This method enables the number of three-phase reaction sites, where the reactant, electrolyte, and electrode are in contact simultaneously, to be significantly increased, which alleviates the rate-determining step of the mass transport of the gas reactants. Nevertheless, mechanical purging requires additional energy input with substantial purging pressure in liquid electrolytes,24–27 neither would this be a fundamental solution if the gas reactants randomly dispersed in the atmosphere were hazardous to capture. Similarly, in the case of an electrochemical reaction involving gaseous products, the rate properties for gas evolution are often hindered by the insufficient elimination of gas bubbles on the electrode surface and the consequent instantaneous deactivation of the electrochemical reaction sites.28–30
To address the inherent limitation of gas diffusion in an enclosed electrolyte space, a cell configuration with an open-type space between the electrodes could be considered as a possible solution for gas-containing electrochemical cells. An open electrochemical system allows the gaseous reactant in the atmosphere to be directly and quickly supplied to the electrode surface, thereby paradoxically indicating that the liquid electrolyte portion, in terms of ion transport during the electrochemical reaction, should be omitted, which would result in an unavailable electrochemical reaction.31,32 This motivated our work, which led us to propose an electrochemical cell system with a new form factor, which employs an “aeroelectrolyte” that simultaneously enables (i) the direct access of gaseous reactants (or the facile release of gaseous products without bubble formation) via an open space between the cathode and anode and (ii) the supply of electrolyte species for electrochemical reaction (Fig. 1). Aeroelectrolytes can be defined as an electrolyte that exists as an aerosol phase consisting of small microdroplets floating in air. The aeroelectrolyte, which is sprayed from an aerosol generator, supplements the ionic species and the formation of an electrode/electrolyte interface in that it is randomly adsorbed on both the anode and cathode.33–35 With this novel phase, the interface boundary between the electrolyte droplets and the electrode inherently forms a large number of three-phase reaction sites (gas reactant/liquid electrolyte/solid electrode) (Fig. S1, ESI†). The aeroelectrolyte system (liquid droplets in a gas atmosphere), as a new form factor, has a structure completely inverse to that of conventional electrochemical cells (gas bubbles in a liquid electrolyte).
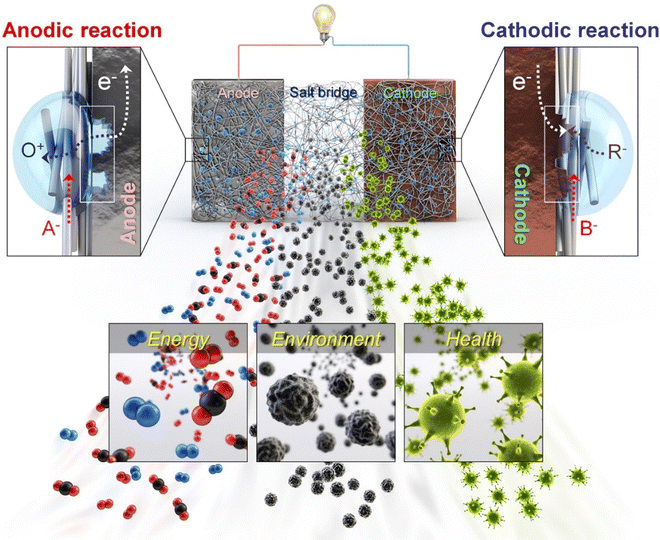 |
| Fig. 1 Schematic illustration of the aeroelectrolyte system. | |
We validated the operation of the aeroelectrolyte system by modifying the configuration of a Daniell cell, which is one of the most basic and simple electrochemical cells. First, the size of the electrolyte droplets was reduced from the bulk scale to ultrafine microparticles by evaluating different spray methods. We introduced solid-state nanofiber-based salt bridges between the electrodes to release the charge stress accumulated in the electrolyte droplets to allow continuous electrochemical reactions. We additionally investigated the effect of the generation pressure and concentration of the aeroelectrolyte on the cell properties. The diffusion behavior of the aeroelectrolyte in the reactor was directly observed in real time using a high-speed camera. The introduction of a new open-type electrochemical cell structure with an aeroelectrolyte system suggests the possibility of further breakthroughs with respect to tailoring atmospheric electrochemical cells.
2. Results and discussion
2.1. Stabilization of droplet electrolyte system and effect of the salt bridge
We tested our hypothesis that an electrochemical reaction with an aeroelectrolyte would be realizable by comparing the electrochemical properties of cells with liquid bath and sprayed electrolytes (Fig. 2a and b). Although conventional bath electrolytes provide a means for ionic species to form interconnection pathways between the cathode and anode, an open space between the electrodes does not provide a transport pathway for the ions generated or consumed during the reaction; in other words, the electrochemical circuit is disconnected. In an open-cell configuration with an aeroelectrolyte, charges accumulate inside the electrolyte droplets during the electrochemical reaction, which obstructs continuous reaction. In addition to the open-cell system, we therefore implemented a solid-state nanofiber-based salt-bridge system, which we applied to a second sprayed electrolyte system for comparison purposes (Fig. 2c). In the case of the Daniell cell reaction (overall reaction: Zn + Cu2+ → Zn2+ + Cu), the positive charges accumulate inside the electrolyte droplets on the Zn anode due to the dissolution of Zn2+ (Zn → Zn2+ + 2e−); likewise, the negative charges gather inside the electrolyte droplets on the Cu cathode because of the consumption of Cu2+ (Cu2+ + 2e− → Cu) (Fig. 2d). This problem can be solved by introducing a porous nanofiber-based salt-bridge system that connects the electrolyte droplets on both electrodes as electrochemical reaction sites (Fig. 2e). This arrangement would enable a cell reaction that occurs inside an electrolyte droplet that is simultaneously in contact with both the electrode and salt bridge to sufficiently release the charge stress phenomena by facilitating ion (either reactant or product) movement between the droplets and the salt bridge. The aforementioned charge stress issue related to the accumulation of Zn2+ at the anode and consumption of Cu2+ at the cathode could therefore be solved by introducing a salt bridge system.36,37 In our work, the salt bridges for the aeroelectrolyte-based electrochemical cells were constructed by using a facile and versatile electrospinning method to form a 1-D nanofiber structure to bridge the space between the Zn anode and Cu cathode (Fig. 2f and Fig. S2, ESI†). This was accomplished by attaching solid-state nanofibers containing polyacrylonitrile (PAN) and polyethylene oxide (PEO) polymers to both electrodes to intentionally face the same direction.38,39
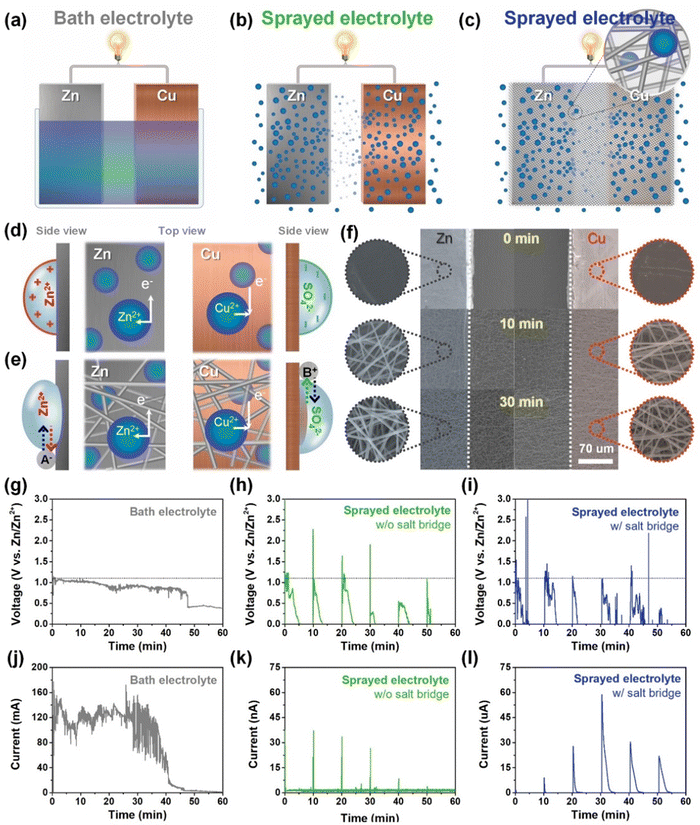 |
| Fig. 2 Introduction of the salt bridge system and sprayed electrolyte. Schematics of the (a) bath electrolyte cell, (b) sprayed electrolyte cell without the salt bridge, and (c) sprayed electrolyte cell with the salt bridge in the Daniell cell system. Schematic representation of the (d) charge stress issue and (e) salt bridge effect in the Daniell cell system. (f) Scanning electron microscopy (SEM) image of the electrodes with a salt bridge, taken with electrospinning times of 0, 10, and 30 min from top to bottom. Cell voltage test of the (g) bath electrolyte cell, and sprayed electrolyte cell (h) without and (i) with the salt bridge. Current test of the (j) bath electrolyte cell, and sprayed electrolyte cell (k) without and (l) with the salt bridge. | |
We compared the electrochemical properties of the liquid bath and sprayed electrolytes to verify the feasibility of the new form factor of electrochemical cells that employ aeroelectrolytes (Fig. 2g–i). In the conventional electrochemical cell configuration with the liquid bath electrolyte, the Zn and Cu electrodes facing each other continuously remained immersed in the same electrolyte bath. For the cell modified with the sprayed electrolyte, the electrolyte solution was sprayed on both electrodes facing the same direction in intervals of 10 min during the measurement. The electrolytes sprayed with and without the salt bridge system were tested simultaneously. In the cell voltage test, the bath electrolyte initially registered an operating voltage of ∼1.1 V and this gradually decreased for ∼45 min (Fig. 2g). Because the chemical oxidizing power of Zn is stronger than that of Cu, Cu was chemically coated onto the surface of the Zn anode to completely cover the surface, which resulted in a rapid voltage drop after 45 min.36,37 The voltage of the cell with the sprayed electrolyte both with and without the salt bridge measured ∼1.1 V while the electrolyte was being sprayed (Fig. 2h and i). This indicates that an electrochemical reaction occurred by spraying a droplet of the electrolyte onto both electrodes, as evidenced from the measured cell voltage of 1.1 V. After full dissolution or consumption of the ionic species from both electrodes, the voltage returned to 0 V. In terms of the current measurement, the cell with the bath electrolyte produced a strong current on the milliampere scale, and the operating voltage gradually decreased with large fluctuations (Fig. 2j). After approximately 42 min, the generated current declined sharply and converged to almost zero. This degradation in the cell reactivity was attributed to the chemical deposition of Cu on the Zn electrode, and corresponds to the results for the bath electrolyte in Fig. 2g. The sprayed electrolyte cell without a salt bridge exhibited a range of low-intensity current pulses in the nanoampere range while the electrolyte was being sprayed, and ultimately converged to almost zero (Fig. 2k) for the duration of the measurement. In contrast, for the sprayed electrolyte cell with the salt bridge, the current increased by more than three orders of magnitude to reach the milliampere scale while the electrolyte was being sprayed, indicating a reaction rate of more than 1000 times higher than that for the cell without the salt bridge (Fig. 2l). In addition, the current remained at levels higher than 0 μA for a much longer time than for the cell without the nanofiber salt bridge. These promising results demonstrated the effectiveness of a salt bridge in an open cell structure using an aeroelectrolyte.
The effects of the density of the nanofibers in the salt-bridge on the electrodes were investigated by controlling the electrospinning time (Fig. S3–S5, ESI†). The SEM images confirmed the proportional relationship between the electrospinning time and dendrite density. Moreover, the cell with the salt bridge that was electrospun for 10 min showed the best reaction stability and highest reactivity compared to the others. An overly low salt bridge density decreases the adsorption probability of the aeroelectrolyte droplets on the salt bridge, which limits the charge stress relief. Conversely, an excessively high salt-bridge density makes it difficult for the aeroelectrolyte to pass through the salt-bridge layer, with the resulting adsorption of the electrolyte onto the electrode. The optimal electrochemical activity therefore requires an appropriate salt-bridge density.
2.2. Introduction of the aeroelectrolyte and its electrochemical properties
The realization of ideal electrochemical cells at atmospheric pressure requires the electrolyte droplets to be much smaller and lighter than the sprayed particles such that the droplets are able to freely float in the air. Using an aerosol generator, we succeeded in considerably diminishing the electrolyte particle size, and named this new electrolyte system with the aerosol phase an “aeroelectrolyte” system (Fig. 3a and Fig. S6, ESI†). The electrochemical properties of the aeroelectrolyte cell were evaluated with and without the nanofiber salt bridge by supplying a continuous stream of electrolyte in the form of an aerosol to the electrodes, (Fig. 3b–e and Fig. S7, ESI†). In the cell voltage tests, the operating voltage of the cell without the salt bridge was much lower (approximately 0.3 V) than the theoretical value of 1.1 V, and the voltage remained consistently unstable (Fig. 3b). On the other hand, in the case of the cell with the aeroelectrolyte and the salt bridge, the cell voltage reached 1.1 V and the electrochemical reaction was stably maintained without degradation (Fig. 3c). In the current test, which reflects the degree of the electrochemical reaction, current generation without the salt bridge was negligible during aeroelectrolyte input (Fig. 3d). In contrast, the supply of aeroelectrolyte to the cell with the salt bridge produced a meaningful current in excess of 1 μA (Fig. 3e). The current detected with the aeroelectrolyte was weaker than that with the sprayed electrolyte because the size of the electrochemical reaction surfaces between the electrolyte droplets and the electrode became smaller by minimizing the droplet size. However, the continuous supply of aeroelectrolyte resulted in a gradual and steady increase in the measured current during the reaction. These results demonstrate that the reactivity of the cell with an aeroelectrolyte is highly sensitive to the presence of a salt bridge. In addition, for an electrochemical reaction that occurs to the same extent or for an identical quantity of ionic species electrified on the surface between the electrode and electrolyte droplets, the charge stress inside the droplets becomes more sensitive as the particle size decreases. Nevertheless, the accumulated charge stress can be successfully relieved by ion exchange in the salt bridge. Moreover, the adsorption of the aeroelectrolyte on the salt bridge can further aid the removal of charge stress to increase the reactivity.
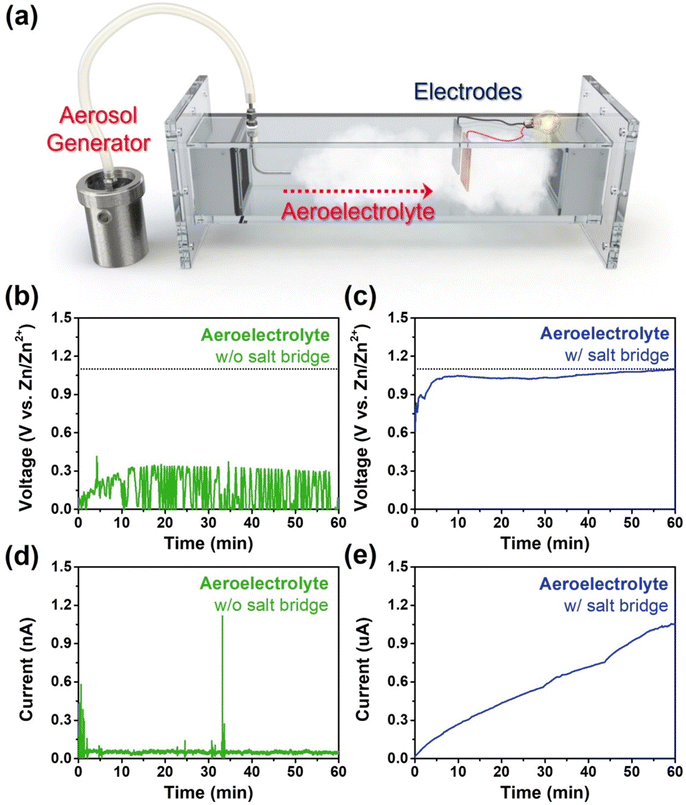 |
| Fig. 3 Introduction of the aeroelectrolyte system and its electrochemical performance. (a) Schematic of the aeroelectrolyte experimental system. Voltage test of the cell supplied with aeroelectrolyte (b) without and (c) with the salt bridge, respectively. Current test of the cell supplied with aeroelectrolyte (d) without and (e) with the salt bridge, respectively. | |
The initial reaction kinetics and ion diffusivity in the aeroelectrolyte cells with and without the nanofiber salt bridge were evaluated by conducting a chronoamperometry (CA) test by applying 1.1 V to each cell (Fig. S7, ESI†). Without the salt bridge, no initial current was detected, and it remained at 0 A, which indicated that the electrochemical reaction did not occur on the electrical double layer (EDL).36,40 In contrast, the aeroelectrolyte cell with the salt bridge generated a strong initial current that reached equilibrium within 30 s. This is because the salt bridge facilitates ion transfer and the reactant is continuously supplied to the EDL.
2.3. Factors controlling the diffusion of the aeroelectrolyte
The physical properties of aeroelectrolytes, such as the droplet size, amount of generated electrolyte, and adsorption power, are important for determining the electrochemical properties of the reaction in a Daniell cell with an open structure.33–35 The diffusion characteristics of the aeroelectrolyte in the cell reactor were examined by adjusting the aerosol generation pressure and concentration (Fig. 4, Fig. S8–S10 and Video S1, ESI†). A high-speed camera was used to observe the diffusion of the aeroelectrolyte toward the electrodes in the reactor. In our experimental system, the particle size distribution of the aeroelectrolyte was largely independent of the aforementioned parameters, with an average size of approximately 300 nm (Fig. S9 and S10, ESI†). However, the amount of aeroelectrolyte generated and the injection power varied significantly with changes in the concentration and pressure of the aeroelectrolyte. An increase in the pressure from 1.5 to 2.5 MPa at the same concentration led to the generation of a greater amount of aeroelectrolyte (Fig. 4a–f). Additionally, the acceleration of the aeroelectrolyte increased with increasing pressure, which, in turn, increased the injection power and turbulence of the aeroelectrolyte. At higher speeds, the aeroelectrolyte continuously circulates in the reactor, thereby increasing the probability of non-adsorbed electrolyte approaching the electrode surface. Variation of the concentration of the generated electrolyte at constant pressure increased the injection power and turbulence of the aeroelectrolyte in the reactor as the molar concentration increased (Fig. 4g–l). This change was more pronounced than the variation resulting from adjustment of the pressure. As for particle propulsion, changes in the concentration below 0.5 M do not significantly affect the speed of individual particles. However, at concentrations of 0.5 M and above, where more particles are generated, the propulsion speed tends to increase due to the influence of the airflow generated by the larger number of particles.
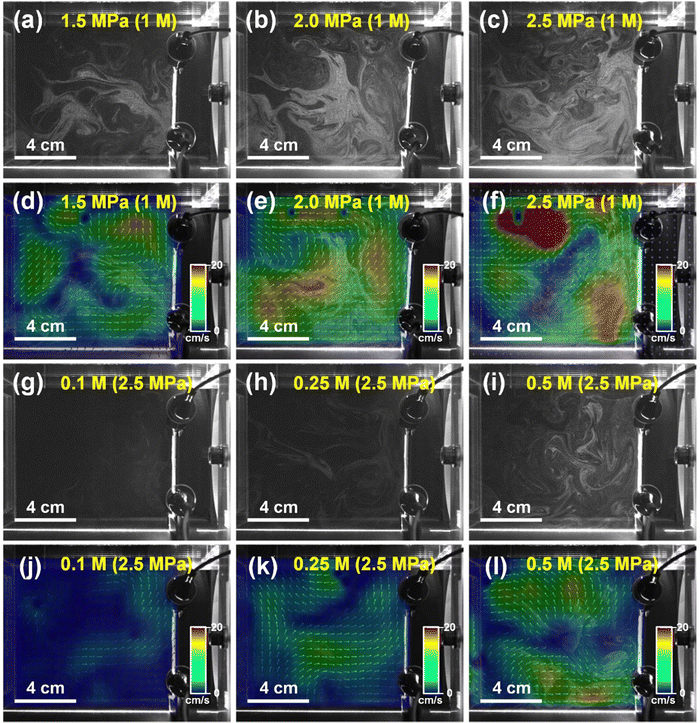 |
| Fig. 4 Effect of the aerosol generation pressure and concentration of the CuSO4 solution on the diffusion characteristics of the aeroelectrolyte. High-speed camera images of the aeroelectrolyte (1 M) generated at pressures of (a) 1.5, (b) 2.0, and (c) 2.5 MPa. Flow rate data of the aeroelectrolyte (1 M) at (d) 1.5, (e) 2.0, and (f) 2.5 MPa. High-speed camera images of the aeroelectrolyte at concentrations of (g) 0.1, (h) 0.25, and (i) 0.5 M at 2.5 MPa. Flow rate data of the aeroelectrolyte for (j) 0.1, (k) 0.25, and (l) 0.5 M at 2.5 MPa. | |
2.4. Electrochemical properties of the aeroelectrolyte under various conditions
The electrochemical reaction behavior of the aeroelectrolyte was investigated for various injection pressures and aeroelectrolyte concentrations (Fig. 5 and Fig. S11, ESI†). The operating voltage of the cell increased as the aeroelectrolyte generation pressure increased from 1.5 MPa to 2.5 MPa and tended toward the theoretical cell voltage of 1.1 V (Fig. 5a). In addition, the operating voltage was independent from the pressure during the reaction. The average operating voltage of the cell for the aeroelectrolyte generation pressures of 1.5, 2.0, and 2.5 MPa were approximately 0.97, 1.05, and 1.07 V, respectively, indicating a relatively small overpotential comparable to that of conventional bath electrolytes (Fig. S11b, ESI†). The current corresponding to the reactivity increased with increasing injection pressure, and this was consistent with the cell voltage measurements (Fig. 5b). Higher injection pressures of the aeroelectrolyte into the open electrochemical cell reactor produced a stable cell voltage close to the theoretical value, and an acceleration of the reaction rate for electrochemical reactions that involved the aeroelectrolyte. To quantify the total amount of product that formed based on the overall current value, the mass of electroplated Cu on the cathode was calculated using the Faraday constant (F = 96
485 C mol−1) (Fig. 5c).41 An increase in the aeroelectrolyte generation pressure from 1.5 to 2.0 MPa resulted in the plating of approximately 11 times more Cu and this amount nearly doubled when the pressure further increased from 2.0 to 2.5 MPa. Although the injection pressure of the aeroelectrolyte is not directly proportional to the total amount of Cu generated, a larger amount of Cu can be plated by raising the aeroelectrolyte injection pressure. These results show that the larger number of aeroelectrolyte droplets generated by increasing the pressure and injection power, induces a strong air circulation flow in the reactor to facilitate electrochemical reactions. We also examined the effects of the aeroelectrolyte concentration as another possible variable. Significant changes in the cell voltage of the reaction were observed at different aeroelectrolyte concentrations (Fig. 5d). The initial operating voltage of each cell increased (0.81, 0.88, 0.93, and 1.07 V) as the concentration increased (0.1, 0.25, 0.5, and 1.0 M) (Fig. S11d, ESI†). However, rather than stabilizing during the reaction, the operating voltage gradually decreased, particularly at lower concentrations, indicating that a severe overpotential was induced by the low ion concentration in the aeroelectrolyte droplets. The current measurements also indicated an increasing trend in reactivity with increasing ion concentration (Fig. 5e). Notably, the concentration of 0.5 M appears to be a pivotal level compared to lower or higher values. As observed in Fig. 4, the number of particles generated and the strength of the air circulation began to rapidly increase at this concentration. At concentrations of 0.1 M and 0.25 M, a weaker current is generated, and the reaction current is quickly saturated. On the other hand, at concentrations of 0.5 M and 1.0 M, the reaction current continued to strengthen. Using these data, the total amount of Cu electroplated on the cathode during the reaction was also calculated, with a tendency toward the increased deposition of Cu at higher concentrations (Fig. 5f). However, an increase in the concentration beyond 0.5 M to 1.0 M did not increase the amount of Cu electroplated on the cathode, thereby demonstrating that the concentration should at least equal the critical level to maximize the number of aeroelectrolyte particles and the diffusion flow corresponding to the electrochemical reaction kinetics.
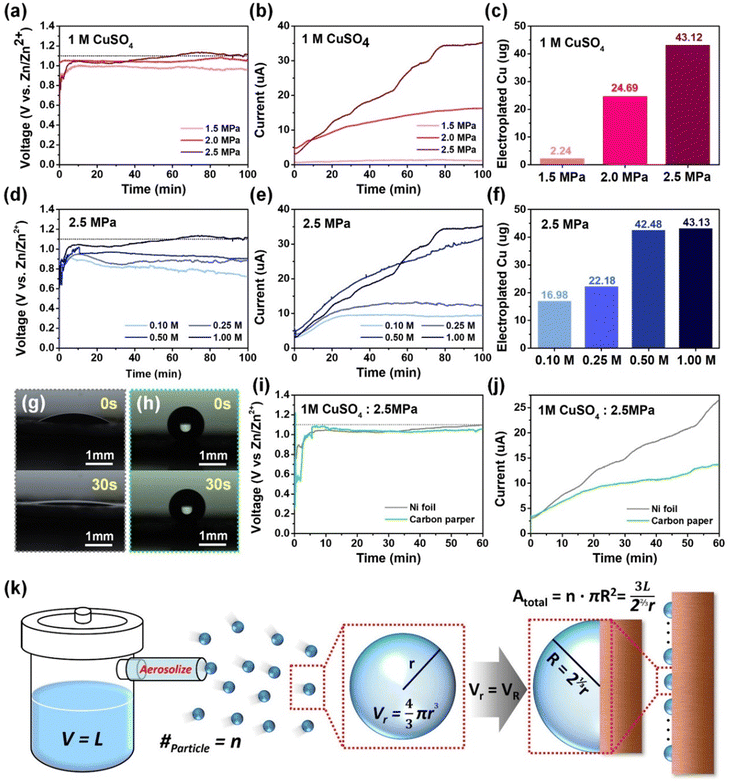 |
| Fig. 5 Electrochemical properties of the aeroelectrolyte cell with various controlling factors. Electrochemical performance at 1.0 M and various pressure levels: (a) cell voltage test, (b) current test, and (c) amount of electroplated Cu. Electrochemical performance at 2.5 MPa for various concentrations: (d) cell voltage test, (e) current test, and (f) amount of electroplated Cu. Contact angle measurement of the electrolyte on (g) Ni foil and (h) carbon paper. The inserted time in seconds represents the elapsed time after the electrolyte adsorbed onto the electrode. (i) Cell voltage test and (j) current test of the Ni foil and carbon paper cathode. (k) Schematic of the relationship among the total contact area of the aeroelectrolyte with the electrode surface, generated amount of electrolyte, and droplet diameter. The symbols in the equations represent the following: the total volume (V) of the aerosolized solution was assumed to be L, total number of aeroelectrolyte droplets formed (n), radius of the generated aerosol (r), radius of the adsorbed aerosol (R) and total adsorption area of aeroelectroylte on the electrode (Atotal). Vr and VR represent the volume of each aeroelectrolyte droplet and adsorbed aeroelectrolyte droplet, respectively. | |
The electrochemical surface area (ECSA), which is related to the contact characteristics between the electrode and aeroelectrolyte droplets, is a crucial variable that determines the electrochemical properties.36 However, under our experimental conditions, the deviation in the particle sizes among the aeroelectrolyte droplets was negligible (Fig. S9–S11, ESI†). Subsequently, the electrochemical reactivity of different types of electrodes and the corresponding ECSA were assessed by evaluating aeroelectrolyte-based cells using hydrophilic Ni foil and hydrophobic carbon paper as the electrodes. By measuring the contact angle between an electrolyte droplet and the electrode, we confirmed that the Ni foil was hydrophilic with a small contact angle, and the carbon paper was hydrophobic with a large contact angle (Fig. 5g and h). Both electrodes delivered the same cell voltage of 1.1 V, which remained stable during the reaction time (Fig. 5i) and indicated that the independence thereof from the surface features. In contrast, in the current test, the hydrophilic Ni foil, on which each aeroelectrolyte droplet occupied a larger contact area than on the hydrophobic carbon paper, produced a stronger current than the carbon paper (Fig. 5j). Using ex situ analysis, the discharge product on the cathode was confirmed to be electroplated Cu (Fig. S12 and S13, ESI†).42–44 Based on these results, the voltage is not significantly influenced by the individual size of aeroelectrolyte droplets related to the ECSA, whereas the current is greatly affected by the size of each particle.
Finally, to conclude the theoretical relationship between the aeroelectrolyte generation conditions and electrochemical reactivity, we simplified our experimental system. The relationship between the total adsorbed aeroelectrolyte area (Atotal), total number of adsorbed electrolyte droplets (n), adsorbed aeroelectrolyte droplet diameter (R), generated aeroelectrolyte amount (L), and aeroelectrolyte droplet diameter (r) can be expressed by the following equation (Fig. 5k):
| 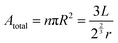 | (1) |
Because the particle size distribution differed negligibly according to our experimental conditions, the particle size was considered to be a constant value. Thus, in our work, the area of the reaction surface is proportional to the amount of aeroelectrolyte generated. In a conventional electrochemical system, the ECSA is considered as the area of the electrode surface exposed to the electrolyte. However, in the open electrochemical system in this study, the ECSA does not exist before the injection of the aeroelectrolyte. Therefore, the ECSA would need to be predicted by statistical calculations or by approximate measurements of the aeroelectrolyte particles.
3. Conclusions
In this study, we customized the foam factor of open-structure electrochemical cells using an aeroelectrolyte for facile atmospheric electrochemical reactions. The Daniell cell system was modified to validate the aeroelectrolyte-based cell. Nanofiber salt bridges were introduced as ion-conduction pathways to connect the electrodes to ensure reaction stability and continuity. The feasibility of the aeroelectrolyte system was confirmed by evaluating the voltage and current generated by an aeroelectrolyte-containing cell with a salt bridge. The high-speed camera observations revealed that the diffusion of the aeroelectrolyte was accelerated by increasing the pressure and concentration for generating the aerosol, while the average size of the individual aeroelectrolyte droplets was consistent at approximately 300 nm. A stable operating voltage close to the theoretical value of 1.1 V and stronger current were also confirmed with increased injection pressure and aeroelectrolyte concentration. The operating voltage remained stable regardless of the type of electrode, yet the current produced on the hydrophilic Ni foil was stronger than that on the hydrophobic carbon paper. Finally, the ECSA associated with the total area occupied by the adsorbed aeroelectrolyte on the electrodes was calculated to determine the theoretical relationship between the physical characteristics of the aeroelectrolyte droplets and the electrochemical reactivity. Aeroelectrolyte-based open-cell systems present a new path for the development of customized gas-related electrochemical reactions.
4. Experimental section
4.1. Electrode preparation
Zn metal (Welcos) was employed as the anode, and the cathode was composed of Cu foil (Welcos), Ni foil (Welcos), or carbon paper (SGL Carbon). The cathode was varied according to the experimental conditions. The dimensions of each electrode were set at 1.8 cm in width and 3.6 cm in height. The electrodes were positioned on a supporting paper substrate with a distance of 0.5 cm between them.
4.2. Salt bridge coating process
0.09 g potassium nitrate (KNO3, 99.0%), 0.5 g polyacrylonitrile (PAN, MW 150
000) and 0.5 g polyethylene oxide (PEO, Mv 100
000) were dissolved in DMF (6 g, 99.8%), and the obtained solution was magnetically stirred at 60 °C overnight. The solution was placed in a 10 mL Luer lock syringe and inserted into the syringe pump, which was approximately 20 cm from the cylinder collector. The solution was injected through a 27-gauge needle and collected on the substrate at an applied voltage of 16 kV to generate an electrical field. The feed rate for electrospinning was 0.3 mL h−1. The amount of salt bridges formed was controlled by varying the electrospinning time. For the cell with the salt bridge, where the electrospinning time was not specified, all samples were electrospun for 10 min. The morphology of the salt bridges was analyzed using scanning electron microscope (SEM, Hitachi).
4.3. Electrolyte preparation
Copper sulfate (CuSO4, 99.0%) was dissolved in distilled water. The bath electrolyte was prepared by placing 50 mL of the CuSO4 solution in a beaker. Additionally, the sprayed electrolyte was prepared by filling 50 mL of the CuSO4 solution into a spray bottle (DH.Bot321, Daihan Scientific) and spraying at 10-min intervals. The aeroelectrolyte was generated by filling 50 mL of the CuSO4 solution into an aerosol atomizer (DS-A103 Atomizer, DONG SUNG Industry Co., Ltd) using compressed air.
4.4. Electrochemical characterization
All the electrochemical properties were evaluated using a potentiostat (SP-200, BioLogic). Open-circuit voltage (OCV) was measured to determine the cell voltage. For the current measurement, a zero-voltage current (ZVC) was applied to measure the current that brought the cell voltage to zero. The measured current was mirrored symmetrically along the x axis to calculate the actual current flow. For the CA test, 1.1 V was applied to each electrode.
4.5. Characterization of the aeroelectrolyte
A high-speed camera (HAS-D71, DITECT) was used to detect aerosol movement. The particle velocity was analyzed using particle image velocimetry software (Flownizer 2D, DITECT). The hydrophilicity and hydrophobicity of each electrode in the electrolyte were assessed by measuring the contact angle between the electrode and the electrolyte drop. The contact angles were analyzed using a contact angle meter (DSA 25, Kruss). The particle-size distribution of the aeroelectrolyte was measured for 30 s using an aerosol spectrometer (11-D, Grimm).
4.6.
Ex situ characterization
A spot-plated electrode was prepared using a bath electrolyte cell that reacted electrochemically for 1 s. An electroplated electrode was prepared using an aeroelectrolyte cell that reacted electrochemically for 2 h. The salt bridge on the electrode was removed, and all electrodes were washed with distilled water and dried before the experiment. Surface characterization of the discharged cathode of the aeroelectrolyte cells was performed using XPS (K-alpha, Thermo UK) and X-ray diffraction XRD (D8 Advance, Bruker).
Author contributions
Yeji Lim and Won-Hee Ryu conceived the study and designed the experiments. Yeji Lim prepared the samples and conducted the electrochemical and material analyses. All the co-authors helped with the experiments. Yeji Lim, Won-Hee Ryu, and all the coauthors discussed and analyzed the data. Yeji Lim and Won-Hee Ryu prepared the manuscript. Won-Hee Ryu supervised the project. All the co-authors agree with the content of the manuscript in its current form.
Data availability statement
Data supporting the findings of this study are available from the corresponding author upon request.
Conflicts of interest
The authors declare no competing interests.
Acknowledgements
This study was supported by grants from the National Research Foundation of Korea (NRF) funded by the Korean government (MSIT) (No. RS-2023-00208983 and 2018R1A5A1025224).
References
- V. Viswanathan, A. H. Epstein, Y.-M. Chiang, E. Takeuchi, M. Bradley, J. Langford and M. Winter, Nature, 2022, 601, 519–525 CrossRef CAS PubMed
.
- S. A. Akhade, N. Singh, O. Y. Gutiérrez, J. Lopez-Ruiz, H. Wang, J. D. Holladay, Y. Liu, A. Karkamkar, R. S. Weber and A. B. Padmaperuma, Chem. Rev., 2020, 120, 11370–11419 CrossRef CAS PubMed
.
- N. D. Popovich, D. Rajagopal, E. Tasar and A. Phadke, Nat. Energy, 2021, 6, 1017–1025 CrossRef
.
- L. Janssen and L. Koene, Chem. Eng. J., 2002, 85, 137–146 CrossRef CAS
.
- T. H. Meyer, I. Choi, C. Tian and L. Ackermann, Chem, 2020, 6, 2484–2496 CAS
.
- F. Duffner, N. Kronemeyer, J. Tübke, J. Leker, M. Winter and R. Schmuch, Nat. Energy, 2021, 6, 123–134 CrossRef CAS
.
- X. Li, Z. Cheng and X. Wang, Electrochem. Energy Rev., 2021, 4, 136–145 CrossRef CAS
.
- G. Korotcenkov, S. D. Han and J. R. Stetter, Chem. Rev., 2009, 109, 1402–1433 CrossRef CAS PubMed
.
- H. S. Kim, B. Kim, H. Park, J. Kim and W. H. Ryu, Adv. Energy Mater., 2022, 12, 2103527 CrossRef CAS
.
- H.-S. Kim, J.-Y. Lee, J.-K. Yoo and W.-H. Ryu, ACS Mater. Lett., 2021, 3, 815–825 CrossRef CAS
.
- B. Kim, K. Shin, G. Henkelman and W.-H. Ryu, Chem. Eng. J., 2023, 477, 147141 CrossRef CAS
.
- X. Wang, Z. Li, Y. Qu, T. Yuan, W. Wang, Y. Wu and Y. Li, Chem, 2019, 5, 1486–1511 CAS
.
- Y.-J. Rho, B. Kim, K. Shin, G. Henkelman and W.-H. Ryu, J. Mater. Chem. A, 2022, 10, 19710–19721 RSC
.
- I. Sullivan, A. Goryachev, I. A. Digdaya, X. Li, H. A. Atwater, D. A. Vermaas and C. Xiang, Nat. Catal., 2021, 4, 952–958 CrossRef CAS
.
- G. F. Chen, S. Ren, L. Zhang, H. Cheng, Y. Luo, K. Zhu, L. X. Ding and H. Wang, Small Methods, 2019, 3, 1800337 CrossRef
.
- X. Sun, P. Wang, K. Davey, Y. Zheng and S. Z. Qiao, Small, 2023, 19, 2303428 CrossRef CAS PubMed
.
- B. Deng, M. Huang, X. Zhao, S. Mou and F. Dong, ACS Catal., 2021, 12, 331–362 CrossRef
.
- C. Xia, P. Zhu, Q. Jiang, Y. Pan, W. Liang, E. Stavitski, H. N. Alshareef and H. Wang, Nat. Energy, 2019, 4, 776–785 CrossRef CAS
.
- S. Kim, H. S. Kim, B. Kim, Y. J. Kim, J. W. Jung and W. H. Ryu, Adv. Energy Mater., 2023, 13, 2301983 CrossRef CAS
.
- P. Zhu, Z.-Y. Wu, A. Elgazzar, C. Dong, T.-U. Wi, F.-Y. Chen, Y. Xia, Y. Feng, M. Shakouri and J. Y. Kim, Nature, 2023, 618, 959–966 CrossRef CAS PubMed
.
- D. Wakerley, S. Lamaison, J. Wicks, A. Clemens, J. Feaster, D. Corral, S. A. Jaffer, A. Sarkar, M. Fontecave and E. B. Duoss, Nat. Energy, 2022, 7, 130–143 CrossRef CAS
.
- T. Liu, J. P. Vivek, E. W. Zhao, J. Lei, N. Garcia-Araez and C. P. Grey, Chem. Rev., 2020, 120, 6558–6625 CrossRef CAS PubMed
.
- E. W. Lees, B. A. Mowbray, F. G. Parlane and C. P. Berlinguette, Nat. Rev. Mater., 2022, 7, 55–64 CrossRef CAS
.
- F. S. Gittleson, R. E. Jones, D. K. Ward and M. E. Foster, Energy Environ. Sci., 2017, 10, 1167–1179 RSC
.
- H. Rabiee, L. Ge, X. Zhang, S. Hu, M. Li and Z. Yuan, Energy Environ. Sci., 2021, 14, 1959–2008 RSC
.
- M. Asadi, B. Sayahpour, P. Abbasi, A. T. Ngo, K. Karis, J. R. Jokisaari, C. Liu, B. Narayanan, M. Gerard and P. Yasaei, Nature, 2018, 555, 502–506 CrossRef CAS PubMed
.
- F. Pan and Y. Yang, Energy Environ. Sci., 2020, 13, 2275–2309 RSC
.
- R. Iwata, L. Zhang, K. L. Wilke, S. Gong, M. He, B. M. Gallant and E. N. Wang, Joule, 2021, 5, 887–900 CrossRef CAS
.
- A. Angulo, P. van der Linde, H. Gardeniers, M. Modestino and D. F. Rivas, Joule, 2020, 4, 555–579 CrossRef CAS
.
- H. Sun, Z. Yan, F. Liu, W. Xu, F. Cheng and J. Chen, Adv. Mater., 2020, 32, 1806326 CrossRef CAS PubMed
.
- J. Li, G. Chen, Y. Zhu, Z. Liang, A. Pei, C.-L. Wu, H. Wang, H. R. Lee, K. Liu and S. Chu, Nat. Catal., 2018, 1, 592–600 CrossRef CAS
.
- M. F. Lagadec and A. Grimaud, Nat. Mater., 2020, 19, 1140–1150 CrossRef CAS PubMed
.
-
C.-s Wen, The fundamentals of aerosol dynamics, World Scientific, 1996 Search PubMed
.
-
W. C. Hinds and Y. Zhu, Aerosol technology: properties, behavior, and measurement of airborne particles, John Wiley & Sons, 2022 Search PubMed
.
- E. Kayahan, M. Wu, T. Van Gerven, L. Braeken, L. Stijven, C. Politis and M. E. Leblebici, J. Aerosol Sci., 2022, 166, 106049 CrossRef CAS PubMed
.
-
A. J. Bard, L. R. Faulkner and H. S. White, Electrochemical methods: fundamentals and applications, John Wiley & Sons, 2022 Search PubMed
.
-
A. V. Da Rosa and J. C. Ordóñez, Fundamentals of renewable energy processes, Academic Press, 2021 Search PubMed
.
- G. Y. Kim, K. R. Yoon, K. Shin, J. W. Jung, G. Henkelman and W. H. Ryu, Small, 2021, 17, 2103755 CrossRef CAS PubMed
.
- G.-Y. Kim, J. Lee, Y.-J. Rho, W.-H. Kim, M. Kim, J.-H. Ahn and W.-H. Ryu, Chem. Eng. J., 2022, 446, 136951 CrossRef CAS
.
- Z. Hou, Y. Gao, R. Zhou and B. Zhang, Adv. Funct. Mater., 2022, 32, 2107584 CrossRef CAS
.
-
M. Schlesinger and M. Paunovic, Modern electroplating, John Wiley & Sons, 2014 Search PubMed
.
- D. T. Tran, S. Prabhakaran, D. H. Kim, N. H. Kim and J. H. Lee, Appl. Catal., B, 2021, 294, 120263 CrossRef CAS
.
- S. Li, P. Ma, C. Gao, L. Liu, X. Wang, M. Shakouri, R. Chernikov, K. Wang, D. Liu and R. Ma, Energy Environ. Sci., 2022, 15, 3004–3014 RSC
.
- W. Kang, H. Guo and A. Varma, Appl. Catal., B, 2019, 249, 54–62 CrossRef CAS
.
|
This journal is © The Royal Society of Chemistry 2024 |