A large-capacity, superhigh-rate integrated lithium metal anode with top-down composition gradient enabled by polyantimonic acid†
Received
8th December 2023
, Accepted 30th April 2024
First published on 1st May 2024
Abstract
Interface engineering is an effective approach to solving the difficult Li-dendrite issues in lithium–metal batteries, and yet a robust large-capacity Li anode (≥20 mA h cm−2) working at an ultrahigh rate density (≥20 mA cm−2) is still rarely achieved. Herein, an integrated Li–metal-based anode with a top-down composition gradient (Li2O–LiOH–Li3Sb/Li) is constructed through a thermal-induced reaction of molten Li with polyantimonic acid (PAA). Unexpectedly, the lattice-water-containing PAA is found in favor of a mild but homogeneous lithiation reaction, whilst accompanied by a spontaneous phase separation between the resulting Li2O, LiOH, and Li3Sb components due to their significant interfacial energy discrepancy. The consequent Li2O-enriched top layer affording a high Young's modulus (>10 GPa) and electron-shielding ability can effectually suppress the Li-dendrite growth, while the underlying LiOH–Li3Sb interphase as ionic-channels homogenize Li+-flux distribution, in turn enabling dendrite-free Li deposition in a lithiophilic Li3Sb/Li bottom layer. With this free-standing integrated electrode, large-areal-capacity symmetrical cells (25 mA h cm−2) can maintain over 1280 h Li plating/stripping cycles at an ultrahigh-current-density of 50 mA cm−2, and the full-cells paired with high-capacity LiCoO2 (3.5 mA h cm−2) exhibit improved cycling stability under a practical low N/P ratio (1.33). Importantly, this robust integrated anode also showcases decent compatibility with gel-polymer/Li7La3Zr2O12 solid-state electrolytes, signifying the application potential in safe Li–metal batteries.
Broader context
Metallic Li is the most auspicious anode material towards next-generation high energy density batteries. However, the challenging issues of uncontrolled Li-dendrite growth and unstable solid electrolyte interface (SEI) have impeded the practical development of Li metal batteries. Although extensive interface engineering strategies have been developed aiming at stabilizing the Li metal anodes, artificial SEI with low ionic conductivity and low interfacial energy has still failed to fulfill the requirements of practical Li anode implementation at high-rate density (>20 mA cm−2) and areal capacity (>10 mA h cm−2). Here, we demonstrate the feasibility of using lattice-water-containing polyantimonic acid in the construction of an integrated Li–metal anode with a unique top-down compositional gradient of Li2O–LiOH–Li3Sb/Li. This gradient electrode structure simultaneously exhibits a high mechanical modulus, superb ionic conductivity, and strong lithiophilicity from the top Li2O-rich artificial SEI to the bottom lithiophilic Li3Sb/Li layer. As such, this integrated Li anode not only achieves unprecedented plating/stripping longevity at both ultrahigh rate density and large capacity, but also showcases enhanced cycling reversibility in practical high-loading, low-N/P-ratio full cells, even if applied with quasi- and all-solid-state electrolytes. This work provides a profitable strategy to design a novel integrated anode with promising electrochemical performance for practical energy-dense Li metal batteries.
|
Introduction
Lithium metal anodes (LMAs), as the ultimate candidate anode for next-generation Li-based battery technologies, have obtained widespread interest due to their unparalleled theoretical specific capacity (3860 mA h g−1) and their lowest reduction potential (−3.04 V vs. standard hydrogen electrode).1,2 However, the practical application of rechargeable lithium metal batteries remains a roadblock that has been jeopardized by the safety issues resulting from Li dendrites,3,4 which triggers more parasitic reactions and accelerates electrolyte depletion with a shorter cycling life and lower Coulombic efficiency.5–7 Essentially, due to the natural solid electrolyte interphase (SEI) with compositional and structural non-uniformity and limited flexibility, it typically gives rise to random Li+-flux distribution and continuous SEI disintegration with preferential sites for heterogeneous Li deposition, thereby increasing the odds of disordered Li dendrite growth. These issues are further exacerbated under the large current densities and high capacities demanded for the next-generation batteries. Encouraged by a growing understanding of the physical and chemical properties of SEI on Li metal, interface engineering is developed to overcome the drawbacks of native SEI. Approaches have been devoted to constructing either in situ8,9 or ex situ10–12 stable artificial SEI (ASEI) on pristine Li with ideal features, such as high mechanical strength, high ionic conductivity, and chemical/electrochemical stability.13
Among them, constructing alloying metal-derived ASEI, that is, introducing Au,14 Ag,15 In,16,17 Sn,18,19 and Sb20 to the Li anode, is deemed a practical method since they can react with Li to form a lithiophilic Li–M alloy interphase (M = Au, Ag, In, Sn and Sb). In particular, lithium-antimony (Li3Sb) alloy as a superionic conductor (Li+-ion diffusion coefficient: ∼2.0 × 10−4 cm2 s−1)20 is regarded as a rapid-ion diffusion layer on the Li anode surface with low interfacial resistance or barriers in both solid-state electrolytes21,22 and liquid-electrolyte systems.23–25 However, the lithiophilic Li-alloy layers are often accompanied by high electronic conductivity and low interfacial energy with metallic lithium, which inevitably induce Li nucleation inside the layer and vertical dendritic Li growth. In this sense, undesirable nucleation within SEI and parasitic side reactions with electrolytes triggered by potential electron leakage are aggravated when the cells operate under high current densities. This explains why long-term cycling stability is scarcely obtained in most Li-alloy-coated LMAs at high current densities.26,27 On the other hand, to achieve thermodynamically-induced lateral mobility of Li-ion along the SEI without vertical dendrite formation during the Li+ plating/stripping process, it is essential for the ASEI layer to possess high surface energy28 and high interface energy to bulk Li, which is a key criterion in the assessment of effectiveness in Li-dendrite suppression.19,29 For example, Goodenough et al.30 and Lee et al.31 have determined that a robust LiF-rich ASEI with high interfacial energy and robust mechanical strength can enhance the Li-electrolyte interfacial stability and dramatically inhibit the Li nucleation within SEI.29 However, to simultaneously fulfill the demands mentioned above of high ion conductivity and high interface energy, it remains a critical challenge to construct an endurable ASEI of intimate interfacial contact with Li substrates that can be compatible with large-capacity (≥20 mA h cm−2), high-rate-current (≥20 mA cm−2), and dendrite-free LMAs.
Different from previous interface engineering involving trivalent antimony (SbIII) compounds such as fluoride,20 sulfide,25 chloride,23 and iodide,24 our research in this work focuses on SbV-containing hydrated antimony pentoxide, (H3O)2Sb2O6·nH2O, also referred to as polyantimonic acid (PAA). PAA has inherent proton conductivity, ionic exchangeability, and thermal resistance, making it useful for various applications, including fuel-cell electrolytes, impurity ion removers, flame retardants, etc.32 Our interest is inspired by its unique pyrochlore structure, where corner-sharing SbO6 octahedra are interconnected forming a 3D open tunnel-like skeleton with interstitial cavities containing hydronium (H3O+) at the tunnel centers (16d position) and zeolitic water molecules at the junction points (8b site) (Fig. S1, ESI†).32,33 The presence of lattice water in PAA is of special intention as they could potentially be involved in the chemical reaction associated with Li and thus generate lithium hydroxide (LiOH). The LiOH component with a Li+-ion conductivity of 1.99 × 10−5 mS cm−1,34 has been confirmed to be capable of enhancing the stability of the Li-electrolyte interface with depressed parasitic reactions.35
As a proof-of-concept study, for the first time, we report a well-developed integrated lithium metal-based anode with a top-down composition gradient of Li2O–LiOH–Li3Sb/Li through a thermal-induced reaction between molten Li and PAA grown on an electro-oxidized carbon cloth (Li-PAA@EOCC). During the transformation of PAA, the formed lithiophobic Li2O is spontaneously isolated from the LiOH and Li3Sb due to the significant interfacial energy difference between Li2O/Li, LiOH/Li, and Li3Sb/Li. This spontaneous phase separation leads to a unique composition gradient in the integrated anode together with the following top-down structural advantages: (i) the top layer of the Li2O-rich artificial interphase affording an electronic insulative ability and high Young's modulus can substantially prevent undesirable Li nucleation within/outwith the ASEI; (ii) the enrichment of ionic-conductor LiOH at the Li3Sb interface induces directional charge transfer to facilitate even distribution of Li+ flux and dendrite-free Li plating in the lithiophilic Li3Sb/Li bottom layer. Hence, the Li-PAA@EOCC as free-standing electrodes not only achieve unprecedented plating/stripping longevity of more than 1280 h at both high rate density and large capacity (50 mA cm−2/25 mA h cm−2) in symmetric cells, but also showcase enhanced cycling reversibility in practical high-loading, low-N/P-ratio full cells, even if applied with quasi- and all-solid-state electrolytes. This work demonstrates prospects to develop a viable approach toward developing a free-standing integrated Li anode for practical application in high-performance lithium–metal batteries.
Results and discussion
Fabrication and characterization of the integrated electrode
As schematically delineated in Fig. 1a, the Li-PAA@EOCC anode was prepared by the hydrothermal growth of PAA crystals on electro-oxidized carbon cloth (PAA@EOCC) followed by molten Li infusion. Before the hydrothermal synthesis of PAA@EOCC, electrochemical anodic oxidation pretreatment was implemented to introduce oxygen-containing functional groups (–COH, –C
O, and –COOH) on pristine CC to improve its surface hydrophilicity, as confirmed by X-ray photoelectron spectroscopy (XPS, Fig. S2, ESI†). Tuning the electro-oxidation time is crucial for the uniform growth of PAA on CC substrate (Fig. S3, ESI†). Field emission scanning electron (FE-SEM) images (Fig. 1b) reveal the change of the CC surface state at different stages. Compared to the smooth surface of pristine CC fibers, the EO-treated CC exhibits a rough surface with exfoliated carbon layers. In the case of PAA@EOCC, a dense layer of octahedral PAA nanocrystals uniformly covers the treated CC fiber surface with a coating thickness of around 300 nm (Fig. 1b and Fig. S4, ESI†) and uniform elemental O and Sb distribution confirmed by the energy-dispersed spectroscopy (EDS) mappings (Fig. 1c). The optical photos manifest the color change for the surface of pristine CC, EOCC, and PAA@EOCC from dark grey, black, to grey-white, respectively (Fig. S5, ESI†). In contrast, very few PAA particles can grow on pristine CC without the EO pretreatment (Fig. S6, ESI†).
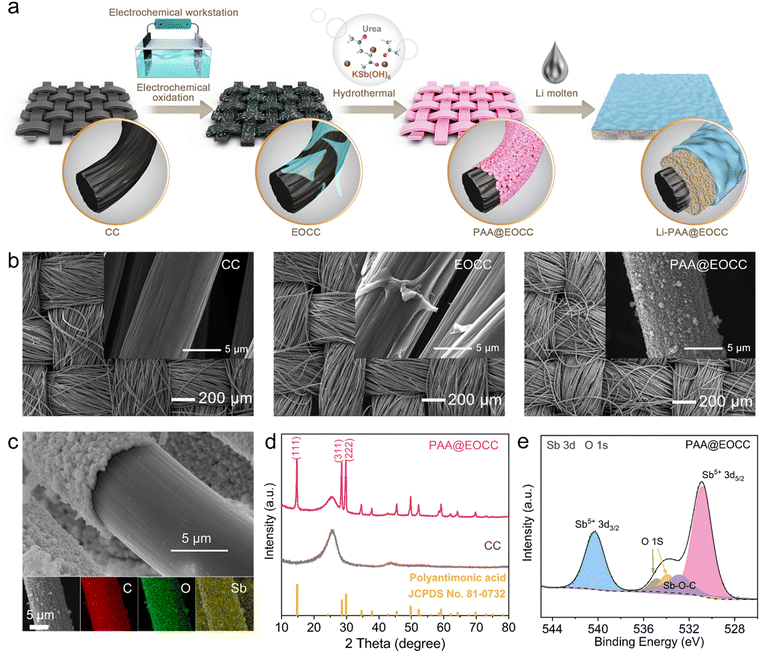 |
| Fig. 1 Design strategy of the integrated electrode. (a) Schematic illustration of the preparation process of Li-PAA@EOCC. (b) SEM images of CC, EOCC, and PAA@EOCC after the corresponding reaction steps (the insets are the magnifications). (c) SEM and corresponding EDS mapping of PAA@EOCC. (d) XRD patterns of CC and PAA@EOCC. (e) Sb 3d XPS spectra of PAA@EOCC. | |
The X-ray diffraction (XRD) pattern in Fig. 1d identifies the good crystalline phase of PAA@EOCC with sharp diffraction peaks indexing to the pyrochlore phase of polyantimonic acid (JCPDS No. 81-0732). The high-resolution Sb 3d spectrum displays two doublets of 3d5/2 and 3d3/2 located at 531.0 eV and 540.4 eV (Fig. 1e), corresponding to the valence states of Sb5+ in PAA@EOCC.36,37 The peak of O 1s introduced by EOCC appears in the neighbouring position of Sb 3d5/2. Besides, the appearance of the unusual Sb–O–C bond at 286.3 eV in the C 1s and 532.8 eV in the O 1s branch (Fig. S7, ESI†) reveals an interfacial chemical bond between PAA and EOCC, ensuring a tight adhesion with each other.38 Thermogravimetric analysis (TGA) was used to determine the content of PAA in the PAA@EOCC. As shown (Fig. S8a, ESI†), the weight loss of PAA@EOCC from room temperature to 900 °C is associated with dehydration, oxygen removal, and carbon decomposition processes,39 and the total mass decreased to the initial weight of 10.3 wt% along with the final product of Sb6O13 (JCPDS No. 33-0111) identified from the corresponding XRD pattern (Fig. S8b, ESI†). Thus, the actual PAA content in PAA@EOCC is estimated to be 14.1 wt%.
Based on the above TGA results, one can note that the lattice water in the PAA can be fully removed by suitable thermal treatment, thus providing us with a viable way to determine the effect of lattice water in PAA on the reaction with molten Li. Consequently, the dehydrated sample without lattice water (denoted as PAA-w/o@EOCC) was obtained by heating PAA@EOCC at 500 °C. XRD and XPS results (Fig. S9, ESI†) confirm the final formation of Sb6O13, consistent with the sample collected in the above TGA. The structural analysis obtained from the Rietveld refinement (Fig. S10 and Table S1, ESI†) further verifies that the hydronium and zeolitic water at 16d and 8b positions are respectively substituted by Sb3+ and O2− after the transition from PAA to Sb6O13 (Fig. S11, ESI†), indicating the complete dehydration in the as-obtained PAA-w/o@EOCC counterpart.
Fig. 2a illustrates the fabrication process of the Li-PAA@EOCC and Li-PAA-w/o@EOCC electrodes using a molten Li infusing approach. This thermal-induced lithiation reaction is mainly driven by the chemical reaction and capillary effects. The color evolution from black to bright grey indicates that the molten Li can be gradually infused into the PAA@EOCC within 2 min (Fig. 2b and Movie S1, ESI†). The loading capacity of Li-PAA@EOCC is determined to be ∼61 mA h cm−2 (Fig. S12a, ESI†), corresponding to the gravimetric capacity of around 2116 mA h g−1 based on the total weight of the electrode (Table S2, ESI†). In sharp contrast, an instantaneous, violent yet heterogeneous lithiation reaction in PAA-w/o@EOCC is carried out within only 2 s, which is also accompanied by sparks (Fig. 2c and Movie S2, ESI†), and finally a higher Li-loading capacity (∼74 mA h cm−2) is obtained (Fig. S12b, ESI†). XRD was employed to understand the compositional evolution after Li infusion. The sharp peaks of metallic Li corresponding to (110), (200), and (211) are clearly detected, suggesting the successful loading of Li. Meanwhile, the presence of diffraction peaks corresponding to Li2O and Li3Sb signifies that both PAA and Sb6O13 experienced a conversion and alloying process, yielding Li2O and stable intermetallic Li3Sb (Fig. 2d). The formation of hexagonal Li3Sb (h-Li3Sb) is in accordance with the Li–Sb binary phase diagram, as depicted in Fig. S13 (ESI†). Of note, the diffraction peaks indexable to the LiOH component are observed in Li-PAA@EOCC, indicating that the crystal water in PAA indeed reacts with molten Li. XPS analysis ascertains that the chemical constituents on both electrode surfaces are Li2O accompanied by a minor amount of amorphous SbOx (Fig. S14, ESI†). The discerned spot of residual SbOx on the surface is likely derived from the partial oxidation of Sb.40 The top-view SEM images (Fig. 2e) show the smooth and flat surface of the Li-PAA@EOCC electrode, indicating the homogeneous infusion of molten Li, while a clear contrast of light and shade from the cross-sectional image demonstrates an obvious up-down tiered structure. Indeed, the EDS mapping reveals that O and Sb are distributed separately in the electrode, where the O element is enriched in the upper layer while the Sb element is concentrated in the lower layer (Fig. 2e), which is also evidenced by the color differences between the upper and bottom surfaces (Fig. S15, ESI†). In contrast, the Li-PAA-w/o@EOCC evinces heterogeneity on the surface, as characterized by the exposed carbon fibers with non-uniform element distribution of O and Sb. Also, no obvious element separation is observed from the cross-sectional SEM images (Fig. 2f). This result indicates that due to the absence of lattice water, the PAA-w/o@EOCC counterpart suffers from a violent reaction with molten Li within split seconds, resulting in insufficient self-diffusion of the products and the final formation of a mixture of Li2O and Li3Sb instead of phase separation, which is similar to other reported works.41
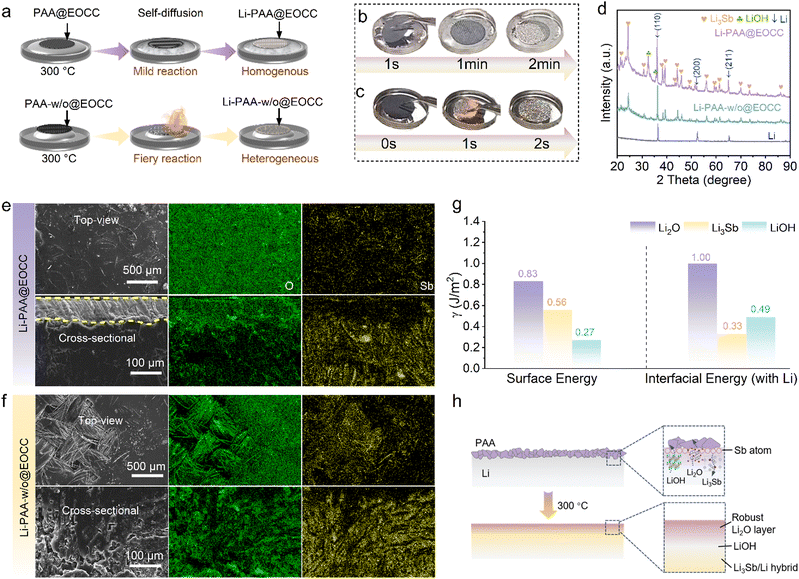 |
| Fig. 2 Fabrication and characterization of the integrated electrode. (a) Schematic diagrams for the fabrication of Li-PAA@EOCC and Li-PAA-w/o@EOCC. Time-lapse images of the molten Li infusion progress of (b) Li-PAA@EOCC and (c) Li-PAA-w/o@EOCC. (d) XRD patterns of Li-PAA@EOCC, Li-PAA-w/o@EOCC, and bare Li. Top-view/cross-sectional SEM images and corresponding elemental mappings of (e) Li-PAA@EOCC and (f) Li-PAA-w/o@EOCC. (g) Surface energies of Li2O (111), Li3Sb (002), and LiOH (001) and the interfacial energies of the Li2O/Li, Li3Sb/Li, and LiOH/Li interface. (h) Schematic diagram of the phase separation upon the PAA and molten Li reaction. | |
Mechanism behind phase separation in the integrated electrode
To unveil the mechanism behind the phase separation in the Li-PAA@EOCC electrode, the surface energies (γX) of Li2O, LiOH, and Li3Sb, and their interfacial energies (γX–Li) relative to bulk Li (Li2O/Li, LiOH/Li, and Li3Sb/Li) were calculated (Fig. 2g), respectively, based on density functional theory (DFT) methods (Fig. S16, ESI†). Generally, the difference between the γX and the γX–Li in a Li–X phase system can thermodynamically drive the phase separation. Based on the thermodynamical derivation, the other phase X on the surface (nX) or in the bulk of Li (NX) is determined using eqn (1):30 | 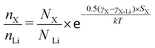 | (1) |
where γX and γX–Li are the surface energy of Li and the interfacial energy with respect to Li, respectively, SX is the surface area of the X particle, k is the Boltzmann constant, and T is the absolute temperature. If γX < γX–Li, then,
indicating more X on the surface of Li; after that, phase separation occurs.
According to the calculation results in Fig. 2g, Li2O exhibits the largest surface energy (0.83 J m−2) and interfacial energy (1.00 J m−2), indicating its strong lithiophobicity.30,42 To minimize the surface energy, Li2O would undergo preferential diffusion, accumulation on Li metal surfaces, and then phase separation from Li metal. As for Li3Sb, it manifests a notably reduced interfacial energy γLi3Sb–Li of 0.33 J m−2 as compared to Li2O, but a higher surface energy γLi3Sb of 0.56 J m−2, indicating that the lithiophilic Li3Sb is inclined to penetrate the bulk of Li. Besides, the compositional gradient at the Li2O/Li interface is also scrutinized by DFT calculations. The atomic structures of Li3Sb/Li2O and LiOH/Li2O with corresponding interfacial energies are summarized in Fig. S17 (ESI†). The Li3Sb exhibits a significantly higher γLi3Sb–Li2O (1.15 J m−2) than γLi3Sb–Li (0.33 J m−2), suggesting its proclivity for enrichment within the bulk of Li (Fig. S18, ESI,† more details are available in Note S2, ESI†). Conversely, the LiOH displays a lower γLiOH–Li (0.49 J m−2) than γLiOH–Li2O (1.18 J m−2), implying the convergence of LiOH at the Li2O/Li interface. Therefore, such surface/interfacial energy differences would propel the multiple components to form a composition gradient thermodynamically, i.e., Li2O–LiOH–Li3Sb, in the resulting Li-PAA@EOCC electrode (Fig. 2h). Furthermore, a verification experiment with pure PAA powder is also designed to confirm the phase separation progress (Fig. S19–S21, ESI†).
Features of the composition gradient in the integrated electrode
To clarify the effectiveness of the Li2O-rich top layer as ASEI in stabilizing the Li metal anode, its physicochemical properties were investigated. The combined assessment of Li/SEI interfacial energy (γ) and bulk modulus (E) of the SEI component, termed as γE, is a pivotal parameter in evaluating Li-dendrite suppression capability.29 The Li2O exhibits a remarkably high γE value of 4868.8 eV Å−2 MPa−1, surpassing that of common SEI compounds (e.g., Li2CO3, LiOH, Li3Sb) (Fig. 3a and Table S3, ESI†). In the Li2O-rich ASEI layer, Li2O is poised to confer robust mechanical strength and resist volume-induced stress during the Li plating/stripping process.
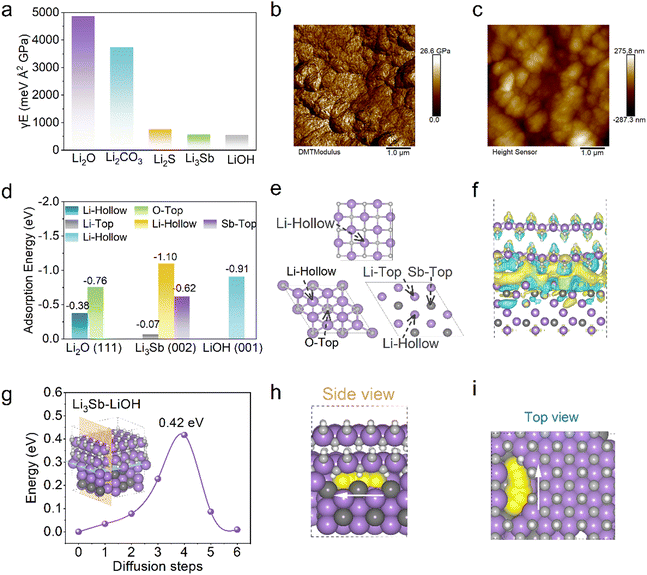 |
| Fig. 3 Mechanical properties and theoretical investigation of the integrated electrode. (a) Summary of Li-dendrite suppression ability γE for different compounds. (b) AFM Young's modulus and (c) AFM surface roughness (area 5 × 5 μm) of Li-PAA@EOCC. (d) Adsorption energy and corresponding adsorption sites for Li atom on the Li2O (111), Li3Sb (002), and LiOH (001) surfaces. (e) Comparison of adsorption energy at different adsorption sites. (f) Charge density difference of LiOH (001) and Li3Sb (002). (g) The calculated energy profile of Li along the diffusion path at the interface of Li3Sb (002)/LiOH. (h) and (i) The diffusion pathway. The charcoal grey, pale grey, purple, white, and yellow balls demote Sb, O, Li, and H and adsorbed Li atoms. | |
As such, the reinforced interphase indeed presents a noteworthy mechanical strength of 10.6 GPa (Fig. 3b), higher than the critical modulus threshold of 6.0 Gpa to inhibit Li-dendrite growth.43 The root mean square roughness (Rq) and average roughness (Ra) are 71.1 nm and 56.2 nm, respectively (Fig. 3c). Besides, the negligible contact angle confirms the desirable favorable electrode/electrolyte interface contact, ensuring a homogeneous distribution of Li-ion flux in Li-PAA@EOCC (Fig. S22, ESI†). The electronic resistivity of the Li2O-rich ASEI is calculated to be 7.4 × 103 Ω cm (Fig. S23, ESI†), comparable to reported values for the Li3Sb/LiF (3.8 × 104 Ω cm)20 and Li13In3/LiCl layer ((0.5 ± 0.2) × 104 Ω cm).44 Conversely, this Li2O-rich layer exhibits exceptional ionic conductivity, reaching 1.21 × 10−4 S cm−1 (Fig. S24, ESI†), which is four-five orders of magnitude higher than the native SEI components Li2CO3 (∼10−8 S cm−1)44 and LiF (∼10−9 S cm−1),45 and is even comparable to the high ionic conductivity of Li2S (10−5 S cm−1).46 In comparison to Li-PAA-w/o@EOCC without the Li2O-rich ASEI, the Li-PAA@EOCC exhibits an auspicious synergy of robust mechanical strength, electronic insulation, and ionic conductivity for the Li2O-rich ASEI; thus, in turn facilitating rapid and uniform Li-ion transport and deposition beneath the ASEI layer (Fig. S25, ESI†).
To gain a comprehensive understanding of the role of LiOH and Li3Sb in bolstering the stability of Li-PAA@EOCC, we conducted DFT calculations. Fig. 3d compares the adsorption energies of Li atoms on Li2O (111), Li3Sb (002), and LiOH (111) surfaces, with the corresponding possible adsorption sites depicted in Fig. 3e. For the Li3Sb (002) surface, the most stable position for Li adsorption is the hollow site with an adsorption energy of −1.10 eV, which is larger than that of Li2O (−0.38 eV) and LiOH (−0.91 eV), indicating that Li+ are prone to uniformly distribute around Li3Sb. The charge density differences further substantiate a solid interfacial interaction between LiOH (111) and Li3Sb (002) (Fig. 3f). Specifically, LiOH readily donates and transfers charge, resulting in charge accumulation at the Li3Sb interface and charge depletion at the LiOH interface. This aligns with the averaged electrostatic potential (ESP) curve of the Li3Sb/LiOH interface (Fig. S26, ESI†). The establishment of directional charge transfer from LiOH to Li3Sb is expected to expedite Li+ transfer kinetics from the LiOH surface to Li3Sb. Hence, incorporating the LiOH component into the Li-PAA@EOCC electrode through the reaction of PAA with molten Li could yield ample LiOH/Li3Sb interfaces beneath the Li2O-rich ASEI. Accordingly, the Li+ diffusion barrier at the Li3Sb (002)/LiOH (111) substantially decreases to 0.42 eV (Fig. 3g–i), which is much lower than that at the Li3Sb bulk (0.74 eV)40 and Li3Sb (002)/Li2O (111) (0.75 eV, Fig. S27, ESI†). These findings directly prove that introducing LiOH into Li-PAA@EOCC is able to effectively enhance Li+ diffusion kinetics within the electrode. To further corroborate that, we measured Li+ diffusion experimentally in the Li-PAA@EOCC and Li-PAA-w/o@EOCC using the galvanostatic intermittent titration technique (GITT) (Fig. S28, ESI†). As expected, the Li+ diffusion coefficient (DLi+) for Li-PAA@EOCC is around 10−8 cm2 s−1, nearly ten-fold higher than that of Li-PAA-w/o@EOCC (10−9 cm2 s−1). Both DLi+ values for Li-PAA@EOCC and Li-PAA-w/o@EOCC align with the reported Li/Li-alloy anodes (10−10–10−6 cm2 s−1)47–49 and significantly exceed the self-diffusion coefficient of bulk Li metal (∼10−11 cm2 s−1) by three orders of magnitude.50
Electrochemical Li plating/stripping performance in symmetrical cells
The electrochemical performance of Li-PAA@EOCC compared to Li-PAA-w/o@EOCC and bare Li under different current densities is scrutinized in symmetric cells. As shown in Fig. 4a, the nucleation overpotential of Li-PAA@EOCC is about 46 mV at a constant current density of 5 mA cm−2, lower than that of bare Li (78 mV), indicating enhanced Li+ transfer kinetics across the Li2O-rich ASEI and uniform Li plating. At 1 mA cm−2 and 1 mA h cm−2 (Fig. 4c), the bare Li suffers from random polarization voltage oscillations within a meager 100 hours due to the emergence of dendritic Li formation, while a prolonged life span of 400 hours is obtained for the Li-PAA-w/o@EOCC electrode due to the presence of lithiophilic Li3Sb alloys. Comparatively, the Li-PAA@EOCC symmetric cell is capable of maintaining an extended lifespan up to 2000 hours with a slight overpotential of 12 mV. Even at the condition of 5 mA cm−2 and 1 mA h cm−2, Li-PAA@EOCC can still stably sustain a lifespan more than 1050 cycles (Fig. S29a and S30, ESI†), remarkably superior to bare Li and Li-PAA-w/o@EOCC symmetrical cells. Moreover, the Li-PAA@EOCC symmetrical cell also showcases a better rate performance than Li-PAA-w/o@EOCC and bare Li electrodes (Fig. 4b). Specifically, dramatically improved stability with relatively lower overpotential values of 14, 18, 28, 34, 53, and 110 mV is observed for the Li-PAA@EOCC symmetrical cell at various current densities of 0.5, 1, 2, 3, 5, and 10 mA cm−2, respectively.
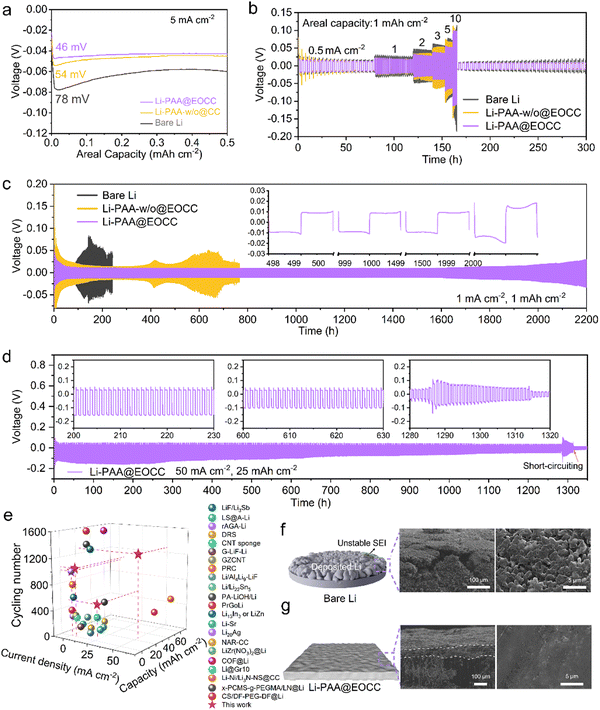 |
| Fig. 4 Electrochemical performance of symmetric cells. (a) Nucleation overpotentials of Li-PAA@EOCC, Li-PAA-w/o@EOCC, and bare Li at a current density of 5 mA cm−2. (b) Rate and (c) cycling performance of symmetric cells with Li-PAA@EOCC, Li-PAA-w/o@EOCC, and bare Li. (d) Cycling performance of the Li-PAA@EOCC electrode in the symmetric cell at an ultrahigh current density of 50 mA cm−2 and large capacity of 25 mA h cm−2. (e) Comparison of the electrochemical performance of Li-PAA@EOCC with previously reported Li–metal anodes. Schematic illustration and SEM images of the deposition morphology between (f) bare Li and (g) Li-PAA@EOCC after plating with 1 mA h cm−2 Li at 5 mA cm−2. | |
The electrochemical Li plating/stripping behavior of Li-PAA@EOCC electrodes was further investigated under practical harsh conditions involving high current densities and large areal capacities. When the applied current density increases to 20 mA cm−2, the Li-PAA@EOCC symmetrical cell shows a stable overpotential of 80 mV for a lifespan of 500 cycles (Fig. S29b, ESI†). Furthermore, the symmetrical cell with Li-PAA@EOCC exhibits sustained operation for 1280 hours with a low overpotential of 100 mV, even at an ultrahigh rate density of 50 mA cm−2 and large areal capacity of 25 mA h cm−2 (Fig. 4d). Note that 50 mA cm−2 is an unprecedented high rate density in the Li–metal anode measurements, which has rarely been adopted, if any. The electrochemical performance of Li-PAA@EOCC also outperforms the majority of reported Li anodes based on interface engineering (Fig. 4e and Table S4, ESI†).
To investigate the kinetics of Li+ plating/stripping in different electrodes, the activation energy barriers pertaining to electrochemical processes were calculated using the temperature-dependent electrochemical impedance spectroscopy (EIS) based on the Arrhenius equation. Compared with bare Li (Ea,SEI = 85.34 kJ mol−1, Ea,ct = 71.33 kJ mol−1) and Li-PAA-w/o@EOCC (Ea,SEI = 75.85 kJ mol−1, Ea,ct = 58.03 kJ mol−1) symmetric cells, the lower activation energy of Li-PAA@EOCC symmetric cells (Ea,SEI = 62.46 kJ mol−1 and Ea,ct = 36.5 kJ mol−1) implies easier Li-ion migration in the SEI layer and faster Li+/Li redox conversion (Fig. S31 and Tables S6–S8, ESI†).51,52 This attests to the expedited kinetics of Li plating/stripping in the Li-PAA@EOCC electrode, which can be further validated by the highest exchange current density of 1.63 mA cm−2 for Li-PAA@EOCC than bare Li (0.42 mA cm−2) and Li-PAA-w/o@EOCC (0.81 mA cm−2) determined by the Tafel plots (Fig. S32, ESI†).
EIS measurements for the multiple cycles of symmetric cells were employed to assess the interfacial evolution of the electrode (Fig. S33 and Table S5, ESI†). Li-PAA@EOCC demonstrates the lowest Li+ transfer resistance (RSEI) (16.43 Ω) and interfacial charge-transfer resistance (Rct) (3.02 Ω) following the initial cycles, signifying its superior ionic conductivity and electrochemical activity. After 30 cycles, the Rct for bare Li declines due to the deterioration of the native SEI and the emergence of high-surface-area dendritic Li. As the cycling proceeds for 50 cycles, the Rct increases for both bare Li and Li-PAA-w/o@EOCC owing to the active Li loss and dead Li formation. In contrast, the Li-PAA@EOCC electrode still maintains a low and stable resistance, indicating enhanced interfacial stability. The interfacial stability for Li-PAA@EOCC after Li deposition was reaffirmed by SEM. As illustrated in Fig. 4g, Li deposition on Li-PAA@EOCC shows a uniform, flat, and dendrite-free surface, while discernible loose and nodular dendrite layers with accompanying cracks are observed on bare Li (Fig. 4f).
Li deposition behavior on the integrated electrode upon cycling
To better capture the microscopic morphology information of deposited Li inside a battery, small-angle neutron scattering (SANS) was carried out (Fig. 5a). After cycling for 100 hours at 1 mA cm−2 and 1 mA h cm−2, the SANS intensity signals were collected at different Li anodes, and they were fitted by the Guinier-Porod model to evaluate the fitted parameters and microstructural variations (Fig. 5b and c), including dimension variable (s), a radius of gyration (Rg), and Porod exponent (d). The s parameter is defined to model geometry objects: for spheres, s = 0, while s = 1 points to rods, and for s = 2 indicates lamellae.53 Generally, the scattering particle size can be estimated by Rg, and the d in the range of 3 to 4 delineates the surface fractal dimension,54 where d = 4 indicates a smooth surface and d = 3 stands for a rough surface. Compared with bare Li and Li-PAA-w/o@EOCC, the SANS intensity curve of Li-PAA@EOCC shifts downward, and the corresponding power law increases from 3.5 to 3.89 with decreasing Rg value, indicating a smaller size of the scatterer and a denser and uniform Li deposition. In contrast, deposited dendritic Li accumulates on the surface of bare Li and Li-PAA-w/o@EOCC, which is reflected in the larger size (Rg) and rougher surface (d). These phenomena coincide with the corresponding SEM images (Fig. S34, ESI†). Note that the cracks are observed on the bare Li surface, which is governed by the consumption of Li+, a process influenced by the constrained interface of microsized lithium grains, consequently exerting an influence on the uniformity of the stripping process (Fig. S35, ESI†).55
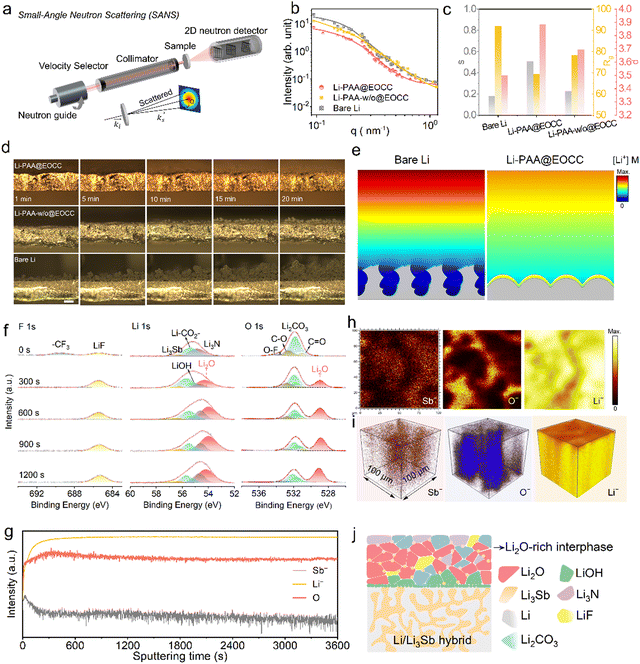 |
| Fig. 5 Microscopic morphology and ASEI composition of cycled electrodes. (a) Schematic diagram of SANS set-up for detection. (b) Fitted curves of SNAS intensity signals for electrodes after cycling. (c) Fitted results of the dimensional parameter, radius of gyration, and power-law exponent. (d) In situ optical microscopy observations of the Li deposition on Li-PAA@EOCC, Li-PAA-w/o@EOCC, and bare Li electrodes under 1 mA cm−2 for 20 min (scale bare, 200 μm). (e) Simulated Li-ion concentration for bare Li and Li-PAA@EOCC. (f) XPS depth profiles of Li-PAA@EOCC electrodes after 20 cycles with Li deposition capacity of 1 mA h cm−2. (g) TOF-SIMS depth profiles with the depth increasing on cycled Li-PAA@CC. (h) TOF-SIMS mapping and (i) 3D spatial distribution of various ions on cycled Li-PAA@EOCC. (j) Schematic illustration of the SEI composition in Li-PAA@EOCC after cycles. | |
The real-time optical microscopy images of the in situ Li deposition were obtained at 1 mA cm cm−2 to directly monitor the Li+ plating progress. Obvious dendritic Li grown on bare Li was observed after 1 min and overgrows over time. Likewise, after 10 min, Li dendrites gradually grow along the edge of Li-PAA-w/o@EOCC without the protection of the Li2O-rich layer. The situation for the Li-PAA@EOCC is quite different, where the gradual alteration in the luster on its surface is attributed to the homogeneous and refined deposited Li grains, forming a smooth and flat surface following 20 min of Li plating. Phase-field simulation of Li-ion concentration was performed to theoretically evaluate the role of the Li2O-rich ASEI in stabilizing the Li metal anodes (Fig. 5e). Bare Li demonstrates an uneven Li+ concentration distribution with notably depleted Li+ near its surface, leading to a high concentration gradient and driving for Li dendrite growth.56 Conversely, Li-PAA@EOCC manifests enriched Li+ concentration within the ASEI layer, accompanied by a reduced concentration gradient, effectively ameliorating the “space charge effect” near the deposition interface.
To gain the constituents of the ASEI after cycling, depth-dependent XPS was performed for its chemical composition detection (Fig. 5f). Detailed analysis of the F 1s spectrum shows that the surface primarily consists of LiF and –CF3, derived from the C–F dissociation in LiTFSI of the ether electrolytes. In parallel, the presence of a Li3N peak at 54.6 eV indicates the decomposition of LiNO3. Before etching, the surfaces of bare Li and Li-PAA@EOCC are chiefly comprised of Li3N, LiF, C–O, C
O, and Li2CO3, being consistent with the constituents found in native SEI formed in ether-based electrolyte,57 whereas a trace of Li3Sb alloys at 56.1 eV could be observed on the Li-PAA@EOCC surface. As sputtering time increases, the signal for Li2O can be detected at 529 eV for O 1s and 54.2 eV for Li 1s spectra. Significantly intensified LiF and Li2O signals are observed in the bare Li upon sputtering, implying continuous electrolyte decomposition and a thick SEI layer formation (Fig. S36, ESI†). In contrast, the component peak intensities for Li-PAA@EOCC, except for Li2O, diminish or remain low with increased sputtering depth. This highlights the stability of Li2O within the ASEI of Li-PAA@EOCC by alleviating undesirable side reactions with electrolytes. It can also be observed that the top interphase of cycled Li-PAA@EOCC is a Li2O-rich layer (O− refer to Li2O), which could be semi-quantitatively determined by time-of-flight secondary-ion mass spectroscopy (TOF-SIMS) profiles (Fig. 5g). The high-resolution overlayer images (Fig. 5h) and corresponding 3D reconstructed sputtering images (Fig. 5i) further reveal the cumulative signals of Li−, O−, and Sb− on cycled electrodes, suggesting that these ASEI components afford high structural and compositional uniformity along the depth direction. Therefore, the upper region of cycled Li-PAA@EOCC can be rationally described as a surface layer dominated by native SEI constituents derived from ether-based electrolytes and the inward transition to an Li2O-rich layer (Fig. 5j).
To better reveal the importance of compositional homogeneity of the Li2O-rich layer, we simulated the current density distribution (Fig. S37, ESI†). Nonuniform Li-ion streamlines amass at the protrusion of the bare Li electrode, characterized by higher current density, and trigger sharp nuclei. For Li-PAA@EOCC, however, the plotted Li-ion flux streamlines converge beneath the ASEI layer, facilitating the redistribution of Li+ and promoting uniform deposition. This integrated Li-PAA@EOCC electrode with the Li2O-rich ASEI layer not only induces dense and homogeneous Li deposition but also enhances cycling stability without dendrite growth and structural change, potentially inhibiting the Li-electrolyte side reaction.
Applicability of the integrated electrode in full batteries
To demonstrate the practical application of the Li-PAA@EOCC, full cells were assembled using the Li-PAA@EOCC as free-standing anodes paired with commercial LiFePO4 (LFP) cathodes. Fig. 6a presents the rate capability of LFP‖Li-PAA@EOCC and LFP‖bare Li full cells at current densities of 0.2, 0.5, 1, 2, 3, 5, 10, and 20C (1C = 170 mA h g−1). Note that the LFP‖Li-PAA@EOCC delivers similar capacities as LFP‖bare Li at low rates (e.g., 0.2C: 158.2 vs.154.9 mA h g−1), but higher capacities at higher rates (i.e., 10C: 95.3 vs. 35.5 mA h g−1) can be achieved for the former one. The superior rate capability of the LFP‖Li-PAA@EOCC benefits from enhanced ion/electron kinetics, as evidenced by the alleviated voltage hysteresis at high rates (Fig. 6b and Fig. S38, ESI†) and reduced interfacial charge transfer resistance (Fig. S39, ESI†). In the subsequent long-cycling test at a current density of 1C, the LFP‖Li-PAA@EOCC delivers a decent capacity retention rate >80% for 1000 cycles (Fig. 6c), indicating the highly reversible Li+ plating and stripping process at the anode. We further evaluate the LFP full cell with a high mass loading. As shown in Fig. S40 (ESI†), when paired with high-mass-loading LFP (12 mg cm−2), the full cell with the Li-PAA@EOCC anode possesses enhanced cycling stability and capacity retention of ∼97.4% after 250 cycles at 1C. While with the bare Li anode, the LFP‖Li full cell shows a similar capacity in the first 70 cycles but experiences gradual capacity decay in the subsequent cycles. Also, a good cycling stability of the full cell can still be obtained even under a higher LFP loading (areal capacity: 2.4 mA h cm−2, Fig. S41, ESI†). For the electrochemical evaluation under more challenging conditions of a large-capacity cathode and limited Li excess, full cells at a practical low N/P ratio of 1.33 were further assembled with high-mass-loading LiCoO2 (LCO, 20 mg cm−2). The as-assembled Li-PAA@EOCC‖LCO full cell displays an initial capacity of 3.5 mA h cm−2 during the first cycle, slowly decreasing to 2 mA h cm−2 after 100 cycles. In sharp contrast, the LCO‖bare Li full cell delivers a lower initial capacity of 3.2 mA h cm−2 and experiences rapid degradation, declining to only 0.5 mA h cm−2 merely after 16 cycles (Fig. 6d). Notably, the Li-PAA@EOCC‖LCO exhibits a reduced overpotential at the onset of the charging plateau (Fig. S42, ESI†, dotted box), signifying accelerated kinetics at the electrode/electrolyte interface. Furthermore, we also attempted to assemble the full cell with a higher-capacity LCO cathode (4.5 mA h cm−2) (Fig. S43, ESI†). Although the initial capacity of Li-PAA@EOCC is relative lower than that of bare Li at 0.1C, it can still be maintained for 30 cycles at 0.2C. In contrast, the bare Li suffers from a dramatic capacity decline only after 7 cycles at 0.2C.
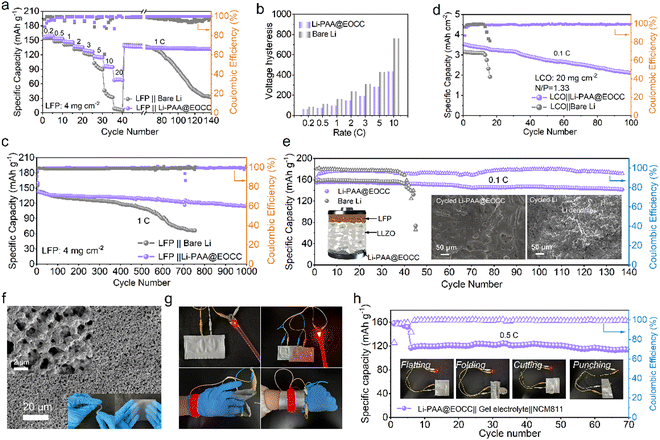 |
| Fig. 6 Electrochemical test results of full cells. (a) Rate performance of LFP‖Li-PAA@EOCC and LFP‖Bare Li cells. (b) Voltage hysteresis of LFP‖Li-PAA@EOCC and LFP‖Bare Li cells at different rates. (c) Long-term cycling stability tests at 1C for 1000 cycles. (d) Cycling performance of LCO‖Li-PAA@EOCC and LCO‖bare Li cells with high mass loading. (e) Cycling performance of Li-PAA@EOCC‖LLZO‖LFP and bare Li‖LLZO‖LFP all-solid-state cells; the insets show the SEM images of the corresponding cycled anode surface. (f) SEM images and optical photos of the PVDF-HFP film. (g) Flexibility test of the quasi-solid pouch cell (Li-PAA@EOCC‖Gel electrolyte‖NCM811) for lighting a red LED bracelet up. (h) Cycling performance of the flexible quasi-solid pouch cell and its powering of an LED under different states. | |
Given the highly stable Li2O-rich ASEI layer in Li-PAA@EOCC, an all-solid-state Li metal battery configuration was coupled with garnet Li7La3Zr2O12 (LLZO) electrolyte and LFP cathode. The commercial LLZO pellet with a diameter of 13 mm and a thickness of 1.1 mm was adopted as the solid-state electrolyte (Fig. S44, ESI†). As shown in Fig. 6e, the capacity retention of LFP‖LLZO‖Li-PAA@EOCC remains at 94.7% after 100 cycles, while LFP‖LLZO‖bare Li shows a rapid capacity decay even after 40 cycles (Fig. 6e and Fig. S45, ESI†). Moreover, a smooth and dendrite-free surface morphology is observed for the cycled Li-PAA@EOCC anode (inset in Fig. 6e), being reasonably in favor of its good electrochemical stability in all-solid-state batteries, whereas in contrast, the severe formation of Li dendrites and dead Li can be observed on the cycled bare Li surface (inset in Fig. 6e).
For wearable electronics and portable storage devices, it is essential to employ high-safety, high-flexibility, and high-energy-density battery systems. Thus, we further investigated the compatibility of the integrated Li-PAA@EOCC anode with a quasi-solid polymer electrolyte, poly(vinylidene fluoride-co-hexafluoropropylene) (PVDF-HFP). As shown in Fig. 6f, the as-synthesized PVDF-HFP is in a gelatinous state, showing an obvious porous microstructure and semi-transparent appearance together with excellent mechanical flexibility. Accordingly, a high-voltage quasi-solid Li metal pouch cell (Li-PAA@EOCC‖Gel electrolyte‖NCM811) was subsequently assembled with Li-PAA@EOCC as the anode and high-nickel LiNi0.8Co0.1Mn0.1O2 (NCM811) as the cathode to showcase practicality in flexible and safe devices. As shown in Fig. 6g, the LED wristband can be powered with equivalent brightness by blending and wearing them on the demonstrator's wrists. In addition, a red LED light can be lightened even under challenging situations, such as cutting and punching, without short-circuiting or cell burnout. Meanwhile, this pouch cell also shows remarkable cycling stability under 0.1C for cell activation and 0.5C for 70 cycles (Fig. 6h and Fig. S46, ESI†). Overall, the Li-PAA@EOCC anode demonstrates an enhanced interface stability to overcome the challenges in liquid/solid-state electrolyte and to be well compatible with each of electrolyte systems (Fig. S47, ESI†), showing the potential versatility in liquid and solid battery systems.
Conclusions
In summary, we have demonstrated a viable approach of using pyrochlore-type PAA to fabricate an ultrahigh-rate, large-capacity integrated Li–metal-based anode with a unique top-down composition gradient of Li2O–LiOH–Li3Sb/Li triggered by their dramatic differences in surface and interfacial energies. Compared with the PAA-w/o counterpart, the lattice-water-containing PAA not only favors the mild yet homogeneous lithiation reaction with molten Li, guaranteeing the sufficient self-regulated diffusion progress of multiple components (Li2O, LiOH, and Li3Sb) to construct an integrated electrode with a reinforced interface, but also underscores the significance of reconfiguration and utilization of interface products (e.g., Li2O) through spontaneous phase separation. In the resultant integrated Li-PAA@EOCC electrode, the bottom lithiophilic Li3Sb/Li mixed layer reduces the interfacial impedance and enables uniform Li deposition, while the top lithiophobic Li2O layer suppresses Li dendrite growth due to a high interfacial energy to Li, robust mechanical strength, and high electron resistance. More importantly, by introducing ionic-conductor LiOH beneath the top ASEI due to the reaction of hydronium and zeolitic water in PAA crystals with molten Li, the interfaces between LiOH and Li3Sb establish directional charge transfer and thereby effectively reduce the Li+ diffusion energy barrier in the whole integrated electrode. The as-developed Li-PAA@EOCC as free-standing anodes exhibit excellent long-term cyclic stability over 1280 hours under a large capacity of 25 mA h cm−2 at an ultrahigh rate of 50 mA cm−2, which far outperforms bare Li anodes. Attributed to these unique structural and composition superiorities, the Li-PAA@EOCC anodes not only bestow impressive cycling performance in high-loading, low N/P ratio full cells, but also showcase decent compatibility with quasi- and all-solid-state electrolytes, suggesting the potential in constructing energy-dense working lithium metal batteries.
Experimental
Synthesis of integrated Li-PAA@EOCC anodes
All chemicals were of analytical grade and were directly used as received without further purification. Firstly, a piece of carbon cloth (4 × 6 cm, mass per unit areal 12 mg cm−2, from CeTech) was cleaned by sonication successively with acetone, alcohol, and deionized water for 30 min each to remove the surface impurity. Secondly, the electrochemical oxidation was carried out in electrodeposition equipment containing NH4H2PO4 (0.174 mol L−1) electrolyte under a constant voltage of 5 V for 5 min to obtain EOCC. Then, the PAA@EOCC was prepared using a hydrothermal method. In a typical synthesis,36 340 mg KSb(OH)6 (Klamar, China, CAS No. 12208-13-8) and 0.9 g urea were dissolved in 37 mL deionized water and thoroughly mixed under magnetic stirring and heated at 90 °C. Sequentially, 23 mL of CH3OH was added into the mixed solution and then transferred into a 100 mL Teflon-lined stainless-steel autoclave, and as-prepared EOCC was put into the solution, followed by a hydrothermal reaction at 170 °C for 12 h to obtain PAA@EOCC.
The infusion progress of molten Li into PAA@EOCC specimens was performed at 300 °C within an Ar-purified glovebox (MBraunLabMaster) with H2O and O2 below 0.1 ppm. Before the molten lithium infusion, PAA@EOCC samples were dried at 110 °C to eliminate the physical adsorbed water on the surface. The polished Li foil was melted in a stainless shell, and it would gradually infuse into PAA@EOCC within 2 min and stood at 300 °C for 10 min to obtain the integrated Li-PAA@EOCC electrode.
Materials characterization
The morphologies and element distributions were characterized via field-emission scanning electron microscopy (SEM, Hitachi S-4800, 10 kV) coupled with an energy-dispersive X-ray spectrometer (EDS). Physical phase identification was conducted using an X-ray diffraction analyzer (XRD, Philips X’pert TROMPD) with monochromatic Cu Kα radiation. The easily oxidized samples were prepared in the glove box and sealed with Kapton film to avoid oxidation for XRD measurements. The XPS measurements were conducted by Thermo Fisher (Thermo Scientific K-Alpha+) with a monochromatic Al Ka X-ray and Ar-ion sputtering gun. The easily oxidized Li-containing samples were prepared in a glovebox and transferred into an XPS instrument via a sealed transfer vessel. A time-of-flight secondary ion mass spectrometry instrument (TOF-SIMS, TOF SIMS5, IONTOFGmbh, Germany) was used to characterize the composition and depth profiles of the PAA@EOCC electrode surface. The data were recorded in a static mode, where the sputtering gun (Cs+) was applied to an area of 50 × 50 μm in a negative mode. We used atomic force microscopy (AFM, Bruker Dimension Icon, Germany) to test the mechanical strength and roughness of the Li-PAA@EOCC surface.
Electrochemical measurements
CR2032-type coin cells were assembled in a highly purified Ar-filled glove box (H2O < 0.1 ppm and O2 < 0.1 ppm). Celgard®2400 was used as the separator for all cells. Two Li foils (thickness of 600 μm, Canrd Co. Ltd, China) or two Li-PAA@EOCC electrodes were assembled with 1.0 M lithium bis trifluoro-methanesulfonamide (LiTFSI) in DOL
:
DME = 1
:
1 vol% with 1% LiNO3 (LS002, Dodo Chem) as the electrolyte for the symmetric cells. A slurry was prepared to fabricate the LFP cathodes by mixing lithium iron phosphate powder, super P, and polyvinylidene fluoride with a mass ratio of 80
:
15
:
5 in N-methyl-2-pyrrolidone as the solvent. The slurry was coated on aluminum foil with a mass loading of 4 mg cm−2. For LFP full cells, the electrolyte used was 1.0 M lithium hexafluorophosphate (LiPF6) in EC
:
DEC = 1
:
1 vol% (LB008, Dodo Chem). The high-mass-loading (20 mg cm−2) LCO electrode slices were purchased from Canrd Co. Ltd. To standardize the testing, 60 μL of electrolyte was used in each coin cell. For the all-solid-state cells, LLZO powder was purchased from KeJing Co. Ltd (Hefei, China). The powder was pressed into pellets with a diameter of 13 mm and a thickness of ∼1100 μm. The full cells were assembled using LFP as the cathode and LLZO attached with Li-PAA@EOCC as the electrolyte and anode, and 5 μL 1.0 M LiPF6 in EC
:
DEC = 1
:
1 vol% solution was added to the cathode side to construct the lithium-ion conducting channel. For the synthesis of gel polymer electrolytes, 0.5 g PVDF–HFP powders (Sigma-Aldrich) were dissolved into 10.0 g NMP and stirred for 5 hours to obtain a precursor solution. Then, 1 g of LiTFSI/1-Ethy-3-methylimidazolium bis (trifluoromethylsulfonyl)imide (EMIMTFSI, Lanzhou Greenchem ILs) (3.8 M) was added to obtain a mixture and then cast on a glass plate. Finally, the PVDF–HFP gel membrane was obtained after drying in a vacuum oven at 80 °C for 12 hours. For the flexible quasi-solid-state pouch cells, the as-prepared Li-PAA@EOCC (30 × 40 mm) anode, PVDF–HFP gel electrolyte with a size of 40 × 50 mm, and NCM811 cathodes (30 × 35 mm) were assembled.
The galvanostatic charge–discharge tests were performed on the Neware Battery testing system (Neware Technology Ltd, China) at 25 °C. The electrochemical impedance spectroscopy (EIS) and linear sweep voltage (LSV) tests were carried out on an electrochemical workstation (PARSTAT MC, AMETEK). Specifically, the EIS tests were probed over 100 kHz to 10 MHz with a perturbation amplitude of 5 mV versus the open circuit potential.
Small-angle neutron scattering measurements
Small-angle neutron scattering (SANS) measurements were performed using the SANS-Suanni spectrometer at the China Mianyang Research Reactor (CMRR). The sample detector distance of 9.9 m with a wavelength of 0.605 nm, resulting in the q ranges going from 0.01 to 0.1 Å−1. The SANS profiles were modeled by the SASfit software.
Computational methods
Density functional theory calculation.
Based on density functional theory (DFT), the first-principles computations were performed by utilizing the Vienna Ab Initio Package (VASP)58 within the generalized gradient approximation (GGA) using the Perdew–Burk–Ernzerhof (PBE) formulation.59 The projected augmented wave (PAW)60 potentials were implemented to describe the electron–ion interactions via a plane wave basis with a cutoff energy of 450 eV and a k-point mesh spacing of 0.03 Å−1 was adopted. The convergence threshold for the electronic energy was set to 10−5 eV. The DFT-D3 method used empirical correction in Grimme's scheme to describe the van der Waals interactions. The climbing image nudged-elastic band (CI-NEB) method in VASP was employed to determine the diffusion barriers for Li atom hopping between the adjacent interstitial sites at the interfaces.61 More detailed information is available in Note S1 (ESI†).
Finite element simulation.
The finite element simulation (FES) was performed to simulate the distribution of electric fields and the concentration of Li+ based on COMSOL Multiphysics. A rectangular electrolyte domain with a size of 34 × 35 μm is applied. The diffusion coefficient in the electrolyte domain was set to 10−10 m2 s−1. The diameter of the carbon nanofiber was set as 9 μm, and a 1 μm thick Li2O-rich ASEI diffusion layer was coated on the carbon nanofiber with a diffusion coefficient of 8.89 × 10−8 cm2 s−1. The initial Li-ion concentration was 1 M, and a constant current density of 2 mA cm−2 was applied.
Author contributions
Y. Y. Z., Y. Z., and H. W. conducted the experiments and designed the project and wrote the manuscript. Y. G., K. Y., Q. W. and M. Y. analyzed the results.
Conflicts of interest
There are no conflicts to declare.
Acknowledgements
We gratefully acknowledge the financial support from the National Natural Science Foundation of China (21878192) and the Sichuan Province Science and Technology Support Program (No. 2022YFG0114). The authors appreciate Dr Xiaoshan Zhang from the Lab Center of the College of Materials Science and Engineering in Sichuan University for the help in SEM measurements.
References
- W. Xu, J. Wang, F. Ding, X. Chen, E. Nasybulin, Y. Zhang and J.-G. Zhang, Energy Environ. Sci., 2014, 7, 513–537 RSC.
- A. Varzi, K. Thanner, R. Scipioni, D. Di Lecce, J. Hassoun, S. Dörfler, H. Altheus, S. Kaskel, C. Prehal and S. A. Freunberger, J. Power Sources, 2020, 480, 228803 CrossRef CAS.
- J. Xiao, Science, 2019, 366, 426–427 CrossRef CAS PubMed.
- R. Bouchet, S. Maria, R. Meziane, A. Aboulaich, L. Lienafa, J.-P. Bonnet, T. N. T. Phan, D. Bertin, D. Gigmes, D. Devaux, R. Denoyel and M. Armand, Nat. Mater., 2013, 12, 452–457 CrossRef CAS PubMed.
- G. Zheng, S. W. Lee, Z. Liang, H.-W. Lee, K. Yan, H. Yao, H. Wang, W. Li, S. Chu and Y. Cui, Nat. Nanotechnol., 2014, 9, 618–623 CrossRef CAS PubMed.
- P. Bai, J. Li, F. R. Brushett and M. Z. Bazant, Energy Environ. Sci., 2016, 9, 3221–3229 RSC.
- W. Deng, X. Yin, W. Bao, X. Zhou, Z. Hu, B. He, B. Qiu, Y. S. Meng and Z. Liu, Nat. Energy, 2022, 7, 1031–1041 CrossRef CAS.
- S. Kim, T. K. Lee, S. K. Kwak and N.-S. Choi, ACS Energy Lett., 2022, 7, 67–69 CrossRef CAS.
- A. Fu, J. Lin, Z. Zhang, C. Xu, Y. Zou, C. Liu, P. Yan, D.-Y. Wu, Y. Yang and J. Zheng, ACS Energy Lett., 2022, 7, 1364–1373 CrossRef CAS.
- Y. Liu, D. Lin, Z. Liang, J. Zhao, K. Yan and Y. Cui, Nat. Commun., 2016, 7, 10992 CrossRef CAS PubMed.
- C. Chang, Y. Yao, R. Li, Z. H. Guo, L. Li, C. Pan, W. Hu and X. Pu, Nano Energy, 2022, 93, 106871 CrossRef CAS.
- S. Li, J. Huang, Y. Cui, S. Liu, Z. Chen, W. Huang, C. Li, R. Liu, R. Fu and D. Wu, Nat. Nanotechnol., 2022, 17, 613–621 CrossRef CAS PubMed.
- Z. Yu, S. Seo, J. Song, Z. Zhang, S. T. Oyakhire, Y. Wang, R. Xu, H. Gong, S. Zhang, Y. Zheng, Y. Tsao, L. Mondonico, E. G. Lomeli, X. Wang, W. Kim, K. Ryu and Z. Bao, Adv. Energy Mater., 2022, 12, 2201025 CrossRef CAS.
- F. Guo, C. Wu, H. Chen, F. Zhong, X. Ai, H. Yang and J. Qian, Energy Storage Mater., 2020, 24, 635–643 CrossRef.
- Y. Jiang, Q. Lv, C. Bao, B. Wang, P. Ren, H. Zhong, Y. Yang, X. Liu, Y. Dong, F. Jin, D. Wang, T. Xiong, H. Liu, S. Dou, J. Wang and J. Xue, Cell Rep. Phys. Sci., 2022, 3, 100785 CrossRef CAS.
- X. Liang, Q. Pang, I. R. Kochetkov, M. S. Sempere, H. Huang, X. Sun and L. F. Nazar, Nat. Energy, 2017, 2, 1–7 Search PubMed.
- S. Choudhury, Z. Tu, S. Stalin, D. Vu, K. Fawole, D. Gunceler, R. Sundararaman and L. A. Archer, Angew. Chem., Int. Ed., 2017, 56, 13070–13077 CrossRef CAS PubMed.
- Z. Tu, S. Choudhury, M. J. Zachman, S. Wei, K. Zhang, L. F. Kourkoutis and L. A. Archer, Nat. Energy, 2018, 3, 310–316 CrossRef CAS.
- R. Pathak, K. Chen, A. Gurung, K. M. Reza, B. Bahrami, J. Pokharel, A. Baniya, W. He, F. Wu, Y. Zhou, K. Xu and Q. (Quinn) Qiao, Nat. Commun., 2020, 11, 93 CrossRef CAS PubMed.
- A. Hu, W. Chen, X. Du, Y. Hu, T. Lei, H. Wang, L. Xue, Y. Li, H. Sun, Y. Yan, J. Long, C. Shu, J. Zhu, B. Li, X. Wang and J. Xiong, Energy Environ. Sci., 2021, 14, 4115–4124 RSC.
- A. Wang, J. Li, M. Yi, Y. Xie, S. Chang, H. Shi, L. Zhang, M. Bai, Y. Zhou, Y. Lai and Z. Zhang, Energy Storage Mater., 2022, 49, 246–254 CrossRef.
- R. Dubey, J. Sastre, C. Cancellieri, F. Okur, A. Förster, L. Pompizii, A. Priebe, Y. E. Romanyuk, L. P. H. Jeurgens, M. V. Kovalenko and K. V. Kravchyk, Adv. Energy Mater., 2021, 11, 2102086 CrossRef CAS.
- S. Wang, J. Chen, H. Lu, Y. Zhang, J. Yang, Y. Nuli and J. Wang, ACS Appl. Energy Mater., 2021, 4, 13132–13139 CrossRef CAS.
- T. Chen, W. Kong, P. Zhao, H. Lin, Y. Hu, R. Chen, W. Yan and Z. Jin, Chem. Mater., 2019, 31, 7565–7573 CrossRef CAS.
- L. Luo, S. Xia, X. Zhang, J. Yang and S. Zheng, Adv. Sci., 2022, 9, 2104391 CrossRef CAS PubMed.
- R. Zhang, X.-B. Cheng, C.-Z. Zhao, H.-J. Peng, J.-L. Shi, J.-Q. Huang, J. Wang, F. Wei and Q. Zhang, Adv. Mater., 2016, 28, 2155–2162 CrossRef CAS PubMed.
- P. Barai, K. Higa and V. Srinivasan, J. Electrochem. Soc., 2016, 164, A180 CrossRef.
- D. Wang, W. Zhang, W. Zheng, X. Cui, T. Rojo and Q. Zhang, Adv. Sci., 2017, 4, 1600168 CrossRef PubMed.
- S. Liu, X. Ji, J. Yue, S. Hou, P. Wang, C. Cui, J. Chen, B. Shao, J. Li, F. Han, J. Tu and C. Wang, J. Am. Chem. Soc., 2020, 142, 2438–2447 CrossRef CAS PubMed.
- T. Wang, J. Duan, B. Zhang, W. Luo, X. Ji, H. Xu, Y. Huang, L. Huang, Z. Song, J. Wen, C. Wang, Y. Huang and J. B. Goodenough, Energy Environ. Sci., 2022, 15, 1325–1333 RSC.
- M.-H. Kim, T.-U. Wi, J. Seo, A. Choi, S. Ko, J. Kim, U. Jung, M. S. Kim, C. Park, S. Jin and H.-W. Lee, Nano Lett., 2023, 23, 3582–3591 CrossRef CAS PubMed.
- S. F. Mayer, J. E. Rodrigues, I. Sobrados, J. Gainza, M. T. Fernández-Díaz, C. Marini, M. C. Asensio and J. A. Alonso, Sci. Rep., 2021, 11, 17763 CrossRef CAS PubMed.
- O. Y. Kurapova, P. M. Faia, A. A. Zaripov, V. V. Pazheltsev, A. A. Glukharev and V. G. Konakov, Appl. Sci., 2021, 11, 11877 CrossRef CAS.
- S. Ma, J. Wang, J. Huang, Z. Zhou and Z. Peng, J. Phys. Chem. Lett., 2018, 9, 3333–3339 CrossRef CAS PubMed.
- Y. Cheng, Z. Wang, J. Chen, Y. Chen, X. Ke, D. Wu, Q. Zhang, Y. Zhu, X. Yang, M. Gu, Z. Guo and Z. Shi, Angew. Chem., Int. Ed., 2023, 62, e202305723 CrossRef CAS.
- B. Wang, Z. Deng, Y. Xia, J. Hu, H. Li, H. Wu, Q. Zhang, Y. Zhang, H. Liu and S. Dou, Adv. Energy Mater., 2020, 10, 1903119 CrossRef CAS.
- X. Zhou, Z. Zhang, X. Xu, J. Yan, G. Ma and Z. Lei, ACS Appl. Mater. Interfaces, 2016, 8, 35398–35406 CrossRef CAS PubMed.
- Z. Yang, W. Li, G. Zhang, J. Wang, J. Zuo, Q. Xu, H. Shan, X. He, M. Lv, J. Hu, W. Huang, J. Zhang and X. Li, Nano Energy, 2022, 93, 106764 CrossRef CAS.
- L. Y. Kovalenko, F. A. Yaroshenko, V. A. Burmistrov, T. N. Isaeva and D. M. Galimov, Inorg. Mater., 2019, 55, 586–592 CrossRef CAS.
- Y. Sun, K. Zhang, R. Chai, Y. Wang, X. Rui, K. Wang, H. Deng and H. Xiang, Adv. Funct. Mater., 2023, 33, 2303020 CrossRef CAS.
- D. Wang, T. Xie, C. Qin, X. Wang, G. Li, Y. Liu, H. Zou, L. Huang and Y. Wu, Adv. Funct. Mater., 2022, 32, 2206405 CrossRef CAS.
- X. Fan, X. Ji, F. Han, J. Yue, J. Chen, L. Chen, T. Deng, J. Jiang and C. Wang, Sci. Adv., 2018, 4, eaau9245 CrossRef CAS PubMed.
- C. Yan, X.-B. Cheng, Y.-X. Yao, X. Shen, B.-Q. Li, W.-J. Li, R. Zhang, J.-Q. Huang, H. Li and Q. Zhang, Adv. Mater., 2018, 30, 1804461 CrossRef PubMed.
- Q. Zhang, J. Pan, P. Lu, Z. Liu, M. W. Verbrugge, B. W. Sheldon, Y.-T. Cheng, Y. Qi and X. Xiao, Nano Lett., 2016, 16, 2011–2016 CrossRef CAS PubMed.
- D. Wu, J. He, J. Liu, M. Wu, S. Qi, H. Wang, J. Huang, F. Li, D. Tang and J. Ma, Adv. Energy Mater., 2022, 12, 2200337 CrossRef CAS.
- H. Chen, A. Pei, D. Lin, J. Xie, A. Yang, J. Xu, K. Lin, J. Wang, H. Wang, F. Shi, D. Boyle and Y. Cui, Adv. Energy Mater., 2019, 9, 1900858 CrossRef.
- S. Jin, Y. Ye, Y. Niu, Y. Xu, H. Jin, J. Wang, Z. Sun, A. Cao, X. Wu, Y. Luo, H. Ji and L.-J. Wan, J. Am. Chem. Soc., 2020, 142, 8818–8826 CrossRef PubMed.
- H. Zhang, S. Ju, G. Xia and X. Yu, Sci. Adv., 2022, 8, eabl8245 CrossRef CAS PubMed.
- Z. Shi, Electrochem. Solid-State Lett., 1999, 3, 312 CrossRef.
- E. Dologlou, Glass Phys. Chem., 2010, 36, 570–574 CrossRef CAS.
- H.-J. Liu, C.-Y. Yang, M.-C. Han, C.-Y. Yu, X. Li, Z.-Z. Yu and J. Qu, Angew. Chem., Int. Ed., 2023, 62, e202217458 CrossRef CAS PubMed.
- Y. Ma, L. Wei, Y. He, X. Yuan, Y. Su, Y. Gu, X. Li, X. Zhao, Y. Qin, Q. Mu, Y. Peng, Y. Sun and Z. Deng, Angew. Chem., Int. Ed., 2022, 61, e202116291 CrossRef CAS PubMed.
- B. Hammouda, J. Appl. Cryst., 2010, 43, 716–719 CrossRef CAS.
-
B. Hammouda, Probing nanoscale structures–the SANS toolbox, 2008.
- D. Luo, L. Zheng, Z. Zhang, M. Li, Z. Chen, R. Cui, Y. Shen, G. Li, R. Feng, S. Zhang, G. Jiang, L. Chen, A. Yu and X. Wang, Nat. Commun., 2021, 12, 186 CrossRef CAS PubMed.
- X. Xu, Y. Liu, J.-Y. Hwang, O. O. Kapitanova, Z. Song, Y.-K. Sun, A. Matic and S. Xiong, Adv. Energy Mater., 2020, 10, 2002390 CrossRef CAS.
- X.-B. Cheng, R. Zhang, C.-Z. Zhao, F. Wei, J.-G. Zhang and Q. Zhang, Adv. Sci., 2016, 3, 1500213 CrossRef PubMed.
- G. Kresse and J. Furthmüller, Comput. Mater. Sci., 1996, 6, 15–50 CrossRef CAS.
- J. P. Perdew, K. Burke and M. Ernzerhof, Phys. Rev. Lett., 1996, 77, 3865–3868 CrossRef CAS PubMed.
- G. Kresse and D. Joubert, Phys. Rev. B: Condens. Matter Mater. Phys., 1999, 59, 1758–1775 CrossRef CAS.
- G. Henkelman, B. P. Uberuaga and H. Jónsson, J. Chem. Phys., 2000, 113, 9901–9904 CrossRef CAS.
|
This journal is © The Royal Society of Chemistry 2024 |