DOI:
10.1039/D3EE02978F
(Review Article)
Energy Environ. Sci., 2024,
17, 867-884
Green recycling of spent Li-ion battery cathodes via deep-eutectic solvents†
Received
6th September 2023
, Accepted 15th December 2023
First published on 26th December 2023
Abstract
The growth in numbers of electric vehicles (EVs) has meant significant demand for lithium-ion batteries (LIBs), together with a need for recycling of spent LIBs. Current existing pyro- and hydro-metallurgical recovery technologies are not satisfactory due to poor economics and risks to the environment arising from high temperature and strong acid(s) used. However, deep-eutectic solvents (DESs) have attracted research attention for recycling of spent LIBs but are far from maturity. In this Review, we critically assess advances and applications of DESs in LIB cathode recycling, with emphasis on rational design and modification strategies to obviate practical problems. We establish DES development, categorize types, with selected important characteristics and quantitative measurement. We categorize design and recent progress of DESs and establish insight into highly efficient recovery of LIBs, and report new experimental data. We evidence leaching mechanism(s) and metal recovery from leach liquor, and assess limitations in DES stability and reusability, together with process optimization(s). We also provide a comparative flowchart of sustainable DES possibilities and practical challenges, together with a prospective on likely future directions and outcomes. We conclude that DESs permit green-recycling of spent LIB cathodes with recovery of important metal resources. Findings will be of interest and benefit to researchers and manufacturers for development of a circular and environmentally benign battery value-chain.
Broader context
To achieve carbon neutrality in transport, the rapid growth of electric vehicles (EVs) has led to a significant global growth in lithium-ion batteries (LIBs). This has necessitated sustainable battery recycling, including principles of circular economy and resource efficiency. Deep-eutectic solvents (DESs) appear advantageous for recycling as innovative ‘green’ solvents. In this timely Review the fundamentals of DESs are critically assessed. Important characteristics for metal leaching are established. Design for efficient and biocompatible DESs for selective metal recovery, together with leaching mechanism(s) are evidenced to highlight advantages of DESs for metal extraction from spent LIBs. Large scale potential for DESs green recycling is assessed, including obviating practical problems and economic and environmental sustainability, and limitations in DES stability and reusability. It is concluded that DESs permit green-recycling of spent LIB cathodes with recovery of important metal resources. Findings will be of benefit to researchers and manufacturers for design for a circular and environmentally benign battery recycling.
|
1. Introduction
There has been a significant increase in demand for clean energy and the switch to electric transport, particular electric vehicles (EVs). Governments globally have begun to introduce legislation to prohibit the sale of petrol-powered vehicles, whilst automotive companies are planning to produce exclusively EVs.1–3 The global EV market has a reported annual growth of 40 to 70% since 2011, with the sale of >2.1 million new EVs in 2019.2 Projections hypothesized by Bloomberg are that this trend will continue with annual global sales of EVs of 24.4 million by 2030.3
Lithium-ion batteries (LIBs) are the primary source of energy storage for EVs and appear essential for sustainable transport. The rapid expansion of demand for EVs has meant a significant demand for LIBs that are an expensive component of EVs. It is reportedly projected that global battery consumption will grow by 25% annually to 2.6 GW h by 2030.4,5 This increase in production of LIBs has directly increased demand for raw material(s), including lithium (Li), cobalt (Co), and nickel (Ni). The market price for these materials has fluctuated significantly in recent years due to imbalances in ‘supply and demand’,6,7 and; the accumulation of waste LIBs has potential risk(s) to the environment. An increase in consumption of metal resources is not sustainable.8 Development is needed therefore of efficient, economic and sustainable recycling for LIBs.9,10
Recycling for extraction of metals from LIBs can be practically classified into three (3) categories, (1) pyrometallurgy, (2) hydrometallurgy, and (3) direct recycling.11–13 Amongst these, pyrometallurgy is the most developed using high-temperature of 1200 to 1600 °C for melting of battery materials with high metal recovery rates of ∼100%. This is a universal method suitable for most batteries and is widely used in Europe, US and Japan. However, it is energy-intensive and not environmentally benign because of production of harmful pollutants including, fluorine, dioxins, and furans. Application is difficult, requiring additional steps.14–16 Recovered products, metal/alloy, cannot be directly used for electrode fabrication. Hydrometallurgy in contrast, is more flexible in using chemical solvents to leach targeted metals, followed by extraction and purification. However, there are practical problems in separating elements with similar properties and it generates significant waste, with risks to the environment.17–20 Despite use in commercial-scale, pyrometallurgy and hydrometallurgy are problematic in economy and sustainability. Direct recycling, retaining the cathode crystal structure for direct regeneration of battery components, is practically more attractive because of reduced energy consumption and waste. This technology is mainly applied for recovering LFP (lithium iron phosphate), and it remains at the laboratory and pilot scale. Because of input chemistry ‘sensitivity’ and poor quality of regenerated battery materials, it is reportedly not well developed and therefore not widely-adopted.21–23 There is therefore a need for suitable methods for sustainable recycling of LIBs.
Deep eutectic solvents (DESs) have attracted attention for recycling of LIBs because of unique properties including, high metal selectivity, cost effectiveness and ‘greenness’.24–26 They obviate significant drawbacks in current pyrometallurgy, hydrometallurgy and direct recycling methods, namely, high energy cost, hazardous waste generation and laboratory-scale limitations. Technical advantages of DES, particularly in metal selectivity, solvent recyclability and applicability to all apparent cathode types make these suitable for commercialization and development of a green battery industry. As a branch of hydrometallurgical methodology, DES resembles the process used for production of new batteries, Fig. 1(a). In comparison with traditional hydrometallurgy, DES has equal or similar leaching efficiency, is more cost-effective and energy-efficient, and; does not require extra reducing agents or waste treatment. A comparison of DES leaching paths with inorganic27–29 and organic acids30–32 is presents as Fig. 1(b). DES is therefore suitable for large-scale and sustainable battery recycling.
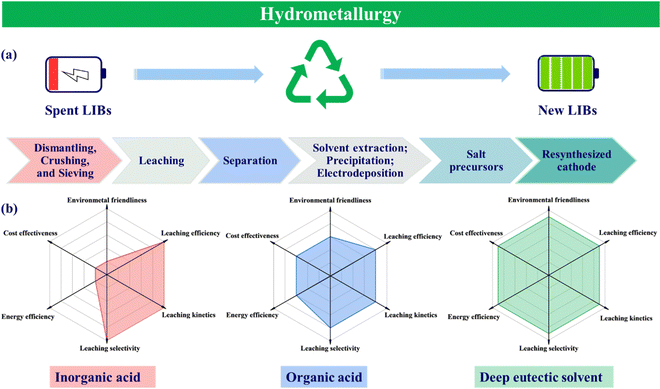 |
| Fig. 1 DESs vs other hydrometallurgical routes for recycling spent LIBs. | |
In this Review we provide a timely, critical, assessment of the fundamentals of DESs in which important characteristics for metal leaching are established. The design for efficient and biocompatible DESs for selectively metal recovery, together with leaching mechanism(s) are evidenced to highlight advantages of DESs for metal extraction from spent LIBs. Potential for large-scale DESs ‘green-recycling’ is assessed, including obviating practical problems and economic and environmental sustainability, and limitations in DES stability and reusability. Likely future directions and outcomes are evaluated in an informed prospective. We conclude that DESs permit green-recycling of spent LIB cathodes with recovery of important metals. Findings will be of benefit to researchers and manufacturers for design for a circular and environmentally benign battery recycling.
2. Development and properties of deep eutectic solvents (DESs) in LIBs recycling
2.1 Development
Discovery of DESs and development in spent LIB recycling is summarized schematically as Fig. 2(a). DES was first reported by Abbott et al.33 in 2003 when investigating the properties of choline chloride (ChCl) and urea mixtures, which exhibited a significantly lower melting point compared with individual components. DESs are characterized by a straightforward synthesis, low toxicity, biodegradability, and ability to dissolve a range of substances including, metal salts and cellulose.34 These unique properties enable DESs for a number of advantageous applications including, in chemical reactions, electrochemistry, CO2 absorption, and metal recovery.35–37 Since 2003, DESs have been reportedly applied widely including, use as solvents and electrolytes. Importantly, the use of DESs in solubilization of metal oxides is reported by Abbott et al.38 in 2006 that evidenced metals extraction from ores, electronic waste and industrial processes. In 2019, Tran et al.39 reported the dissolution of cathode materials by DES following dismantling of LIBs, namely, separating foil, binder, and residual conductive carbon through leachate filtering. They reported that DES (ChCl–EG) leached out 99.3% Co from LCO (lithium cobalt oxide) and was also effective in extracting 71% Li and 32% Co from NCM (lithium nickel manganese cobalt oxide). Subsequently, DES received significant research attention and showed great promise for the future of sustainable battery recycling. DES formulations were developed for specific metals and processes, for example, in 2020, researchers reported DES systems consisting of ChCl with urea,40 citric acid, malic acid (MAL), malonic acid, oxalic acid (OA),41 and PSA (p-toluene sulfonic acid).42 In 2021, other research teams developed novel DESs containing ChCl and monocarboxylic acids (formic acid FA, acetic acid AA, propionic acid PA, n-butyric acid BA),43 together with recovery of metals from leachate and reuse of DES.44
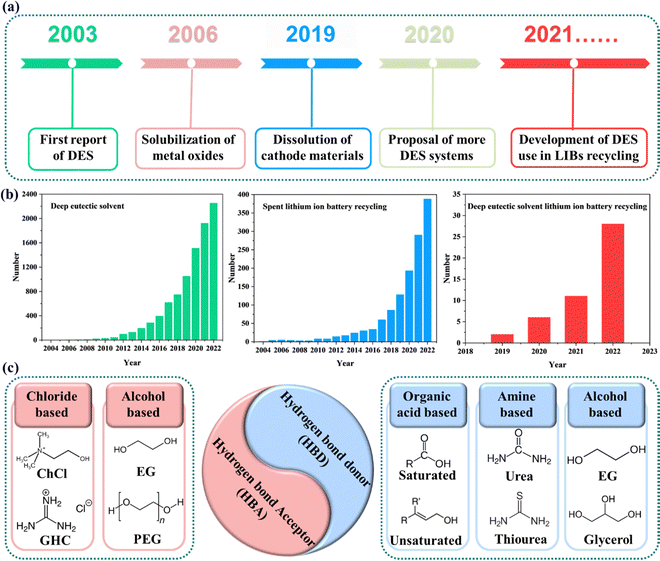 |
| Fig. 2 (a) Timeline for DESs development and application to recycling of spent LIBs; (b) publications from Web of Science with keywords “Deep eutectic solvent”, “Spent lithium ion battery recycling” and “Deep eutectic solvent lithium ion battery recycling”; (c) common HBDs and HBAs of DESs used in LIBs recycling. | |
Based on findings from Web of Science (accessed August 2023), the number of articles reporting on DESs and application to recycling of spent LIBs has exhibited a significant increase in the past two decades. In 2022, the number of articles on DESs exceeded the number on spent LIBs recycling by >fivefold. However, articles on recycling of spent LIBs via DES is <1% of the total number on spent LIBs recycling, and <0.1% of the total of articles on DES, Fig. 2(b). These findings evidence an increased research interest and significant potential in DES for application to LIB recycling. It is important to therefore understand fundamentals and development of DESs for designing and application to battery recycling.
DESs can be readily prepared via combining hydrogen bond acceptor (HBA) with hydrogen bond donor (HBD) in specific proportions.36 DESs can be conveniently classified into five (5) types based on composition, namely, Type: I comprises organic salt and metal salt, II is similar however uses metal salt hydrate, III contains organic salt and HBD, IV consists of metal salt and HBD, and; V is emerging category of non-ionic DESs including, menthol, thymol and trioctylphosphine oxide (TOPO).45–47 Among these, Types I, II and IV eutectics are commonly used in metal deposition, superconductors and lasers.48,49 Type III DESs have been reported for battery recycling. DES types are presented as Fig. 2(c).
2.2 Physiochemical properties and measurement of DESs
Capability of DESs is directly related to properties including, reducibility, acidity and viscosity. Additionally, they can however be toxic.
Reducibility.
The reducibility of DES is important in determining leaching efficiency of high valent metals, and is significantly influenced by the HBD component. Accurate determination therefore of reduction ability of HBD is needed. Fukui function calculations40 and ionization potential (IP) value50 are two (2) means for quantitatively comparing reducibility of DES, and significantly, predictions can be tested via cyclic voltammetry (CV). Fukui function is based on density functional theory (DFT) and is used to determine variation of electronic density over a reaction.51 The value, fA is the reducibility of atoms according to ‘attack’ type and ability. The calculation determines the likelihood of HBD losing electrons during oxidation and is obtained from: | fA+ = qA(N) − qA(N + 1), nucleophili attack | (1) |
| fA− = qA(N − 1) − qA(N), electrophilic attack | (2) |
| fA− = (qA(N − 1) − qA(N + 1))/2, radical attack | (3) |
where (qA(N − 1)), qA(N) and (qA(N + 1)) are, respectively, the charge on atom A in the cationic, neutral, and anionic, species.
The IP value evidences redox reactions and electron transfer. A low IP value indicates strong reducibility and a (more) reductive DES. IP can be divided into, (1) vertical IP (VIP) and (2) adiabatic IP (AIP), depending on whether relaxation or reorganization of the electron density is considered during electron removal. VIP is the minimum energy required for a molecule to lose an electron without changing structure, whilst AIP is the relaxation energy of the atom. VIP reportedly exhibits a linear relationship with oxidation potential, permitting direct prediction of reducibility based on negative energy value of the highest occupied molecular orbital (HOMO) energy in DFT, namely:50
| VIP = EV(N − 1) − EV(N) | (4) |
| AIP = EA(N − 1) − E(N) | (5) |
where
N is the number of electrons in the molecule,
E(
N) the neutral molecular potential without charge and
EV(
N − 1) and
EA(
N − 1), respectively, vertical and adiabatic potential energy when the molecule loses an electron.
VIP and AIP values are important in understanding reductive ability of DESs and for designing and optimizing DESs for metal recycling.
Acidity.
The acidity of DESs significantly affects the leaching of transition metal ions from spent LIB cathode material. Generally, acidic DESs are reportedly better at dissolving metal oxides than neutral and alkaline DESs. Amongst reported DESs, ChCl–urea is alkaline with a pH of 8.9, ChCl–glycerol and ChCl–EG are neutral with pH of 7.5 and 6.9 at 25 °C, whilst remaining DESs are frequently used as acidic with pH ranging from 0 to 3.52–54 However, direct pH measurement for some DESs is not practical and requires dilution with water, which can alter hydrogen bond structures of the DESs. Certain DESs, especially those that are hydrophobic, are non-aqueous that cannot be distinguished by pH measurement. It is necessary therefore to quantify the acidity of DES systems using alternative methods including Hammett acidity (H0), depending on particular properties.55 The Hammett acidity is suitable for highly concentrated acid solutions with negative pH values, or non-aqueous systems. Determination depends on UV-Vis spectroscopy using a basic indicator in water to measure the absorbance ratio of the protonated and deprotonated forms of the indicator.56 The acidity of DES is then computed from: | 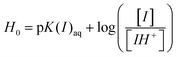 | (6) |
where I is indicator, pK(I) is ion equilibrium constant for indicator in water, [I]/[IH+] the absorbance ratio measured before and following addition of DES. The H0 value exhibits an inverse relationship with acidic ability.57,58 This quantification of acidity is important in designing DESs with the desired viscosity and hydrogen bond properties, especially for hydrophobic DESs that have a lower dielectric constant than hydrophilic ones.
Viscosity.
DESs exhibit greater viscosity than aqueous solutions with majority >100 cP at ambient temperature.59 The high viscosity adversely affects mass transfer, phase(s) separation and ionic conductivity, whilst too low a viscosity evidences a weaker intermolecular hydrogen bond network within the DESs components, leading to reduced metal dissolution. With ChCl-based DESs, the viscosity is strongly correlated with HBD type. The use of alcohols, carboxylic acids, sugars, or inorganic salts increases viscosity.47 In practical applications, the viscosities of DESs can be adjusted by adding a particular amount of water, or increasing temperature. The presence of water can significantly decrease viscosity of DES, sometimes by 10 to 30× times, and reportedly up to 200 × times in ChCl–OA at room temperature.60 However, an ‘excess’ of water alters the hydrogen bonding structure of DESs, which is not beneficial for efficient leaching. There is reportedly a relationship between temperature and DES viscosity that is described by Arrhenius and Vogel–Fulcher–Tammann equation (VFT) models over a narrow, and wide temperature range, respectively, namely:25,58 |  | (7) |
|  | (8) |
where η is the dynamic viscosity, E is the activation energy (kJ mol−1), A and A′ (Pas), B (K), and T0 (K) are empirical constants. This advantageously permits practical design of DESs with appropriate viscosity via controlling water content, or temperature.
Biodegradability and toxicity.
Because of the ‘naturalness’ of HBDs and HBAs, all DESs are reportedly ‘readily biodegradable’, however a systematic toxicology is not reported. DES can form supramolecular structures through hydrogen bonding, it is necessary therefore to find quantitative methods to establish biodegradability and toxicity.61,62 The biodegradability of DES was measured via a ‘closed bottle’ test described in OECD Guideline 301 D and independently reported.63–66 The biodegradation for amine-based DESs was maximum, followed by sugar-based, alcohol and acid DESs. It is reported that hydroxyl groups in the HBD exhibited positive effect, however, water content was hypothesized to have dual influences.67,68 Significantly, there is little report on microbial toxicity of DES, however limited information is available on methods used, namely: (1) agar and broth dilution, have been used to determine minimum inhibitory concentration of antimicrobial agents under specified test conditions that can cause complete microbial death – this however is not suitable for viscous or HDES;69,70 (2) well diffusion and disk diffusion are used as facile methods to establish antimicrobial activity of DES via measuring the inhibition zone diameter – however these reportedly are not suitable for HDES and, not reproducible;69,70 (3) Microtox assay, an in vitro detection using bioluminescent bacteria Aliivibrio fischeri to establish toxicity of diluted DESs samples including, ChCl-based DESs, however this gives only a relative toxicity;67,71,72 (4) FTIR-based bioassay that uses FTIR spectral change(s) to estimate toxicity of DESs. This method generates metabolic indexes that can be used for classification and relative quantification, indicating fast and reproducible capability.73
To ensure safe use of DESs in LIB recycling (and other applications), it is concluded that it is important to quantitatively establish physiochemical properties.
3. Application of DESs in LIBs recycling
3.1 Designing DESs
To boost leaching efficiency under mild reaction conditions, reported research has focused on targeted design for DESs via careful regulation of components. Table 1 presents a comparative summary of reported findings, together with new unreported findings from the present authors. Our findings include hydrophilic DESs with varying HBD with ChCl as the HBA, and hydrophobic DESs with DL-menthol as the HBA.
Table 1 Comparative summary of reported and unreported preliminary findings for varying HBD with ChCl as HBA
Variation factors |
DES components |
Cathode material |
Molar ratio (HBA/HBD) |
Leaching efficiency (%) |
HBA |
HBD |
Li |
Co |
Ni |
Mn |
Coordination |
ChCl56 |
EG (TFSA addition for pH = 3) |
Mixed MOs |
1 : 2 |
— |
0.0008 mol L−1 CoO |
0.0001 mol L−1 NiO |
0.0011 mol L−1 MnO |
EG (LA addition for pH = 3) |
Mixed MOs |
1 : 2 |
— |
0.06 mol L−1 CoO |
0.0012 mol L−1 NiO |
0.13 mol L−1 MnO |
|
Acidity of HBD |
ChCl |
Malonic acid |
LiMn0.15Co0.25Ni0.6O2 |
4 : 1 |
64.8 |
30.2 |
52.4 |
44.1 |
Citric acid |
|
4 : 1 |
72.8 |
50.5 |
63.3 |
86.9 |
Succinic acid |
|
4 : 1 |
35.9 |
10.3 |
19.9 |
6.9 |
Lactic acid |
|
1 : 2 |
63.5 |
29.0 |
47.7 |
44.0 |
Tartaric acid |
|
4 : 1 |
46.0 |
24.6 |
37.1 |
38.8 |
L-Ascorbic acid |
|
4 : 1 |
76.5 |
78.6 |
51.4 |
86.8 |
Maleic acid |
|
4 : 1 |
94.9 |
46.7 |
74.5 |
57.9 |
Acetic acid |
|
1 : 2 |
62.8 |
25.8 |
45.6 |
28.6 |
Oxalic acid |
|
4 : 1 |
79.1 |
1.0 |
1.0 |
30.8 |
|
Reductivity |
ChCl |
Acetic acid |
NCM111 |
2 : 1 |
12 |
5 |
5 |
6 |
L-Ascorbic acid |
|
|
96.2 |
98.1 |
98.9 |
99.3 |
|
Hydrophobic DESs |
TOPO89 |
decA |
LCO |
1 : 1 |
93 |
90.6 |
— |
— |
HBTA |
LCO |
1 : 2 |
∼100 |
98 |
— |
— |
HBTA |
NCM111 |
1 : 2 |
98 |
97 |
98 |
99 |
HBTA |
Black mass |
1 : 2 |
92 |
97 |
98 |
78 |
DL-Menthol |
Octanoic acid |
LCO |
1 : 1 |
1.0 |
0.04 |
— |
— |
NCM523 |
|
4.7 |
0.05 |
— |
0.1 |
NCM811 |
|
9.9 |
|
0.1 |
— |
Decanoic acid |
LCO |
1 : 1 |
1.1 |
0.03 |
— |
— |
NCM523 |
|
5.2 |
0.04 |
— |
— |
NCM811 |
|
6.4 |
— |
— |
— |
Acetic acid |
LCO |
1 : 1 |
1.6 |
0.26 |
— |
— |
NCM523 |
|
8.7 |
1.8 |
1.9 |
2.1 |
NCM811 |
|
21.1 |
5.5 |
5.7 |
6.1 |
Formic acid |
LCO |
1 : 1 |
0.5 |
0.02 |
— |
— |
NCM523 |
|
0.1 |
— |
— |
— |
NCM811 |
|
0.1 |
— |
— |
0.3 |
Lactic acid |
LCO |
1 : 2 |
30.3 |
0.7 |
— |
— |
NCM523 |
|
67.4 |
1.2 |
2.5 |
14.7 |
NCM811 |
|
66.3 |
1.8 |
2.6 |
16.5 |
3.1.1 Adjusting HBA and HBD.
Based on HBA, DESs can reportedly be conveniently divided into chloride-based and alcohol-based, categories. For example, with chloride-based DESs using ChCl as HBA, ChCl–alcohol and ChCl–amine DESs require higher leaching temperature, whilst ChCl–carboxylic acid DESs operate at mild leaching conditions with no apparent corrosion. Alcohol-based DESs with EG or PEG as HBA reportedly need longer leaching times (≥6 h). Molar ratios for organic acids to ChCl are often higher than those for alcohols in corresponding DES type. This reported finding confirms capability of ChCl–carboxylic acid DESs for recycling cathode active materials.74
Amino acid-based DESs however exhibit Type 1 toxicity, and therefore the mole ratio reportedly needs careful control to minimize toxicity. Both HBA and HBD have an impact on the toxicity of DESs, with HBD the greater. In customizing DESs with low or no toxicity, it is advisable therefore to select Type 2 DESs over Type 1 and Type 3.75 Pateli et al.56 reported a comparison of ChCl-based DES systems with strong, or weak ligands as HBDs, in which those with stronger coordination exhibiting better leaching. The leaching performance of DESs is also influenced by acidity, as evidenced by the pKa for carboxylic acids, with more acidic DESs showing better leaching performance. DESs with stronger reducibility reportedly result in higher leaching efficiencies of spent LIBs.40,50 In addition to component regulation, the molar ratio of HBA to HBD reportedly needs to be carefully considered, and, additionally, the impact of additives in DESs including, ethanol and deionized water. In binary mixtures comprising alcohol and ChCl-based DES, viscosity increases with longer alcohol chains.76 These additives have practical potential therefore to modify the physicochemical characteristics of DESs, and affect leaching performance. Importantly, via a strategic selection and optimization of DES components affecting toxicity, complexity, acidity, reductivity and viscosity, DESs can be ‘tailored’ for specific LIBs chemistry.50,77
3.1.2 Controlling water content in DES.
In DES application to LIBs recycling, the presence of water has significant implication(s) as an additive, with reportedly the optimal water content of 5–10 w/w%.78 One advantage is the ability to reduce disproportionate viscosity, permitting a solid–liquid separation under lower temperature without the need for toxic diluents such as DMSO. By reducing viscosity, recycling becomes has less risk to safety and more environmentally benign.40,79 Additionally, low-concentration liquids, including DES with water, have a high ion diffusion rate. This is advantageous for leaching because it is controlled via diffusion. Significantly, water usage is more cost-effective compared with other reagents. Because of ready availability, affordability, and no (generally) logistical complexity, water contributes to economic feasibility of LIBs recycling.
However, disproportionate (excessive) water content disrupts the hydrogen bond structure of DESs, leading to changes in the composition and behaviour of metal ions that impact efficiency of leaching. For example, Amphlett and Choi (2021) reported the hydrolysis of Ni2+ and Co2+ metals in ChCl–urea solution and the replacement of EG ligands in EG-malonic acid system at >30 w/w% water.80 It is important therefore to maintain appropriate water to preserve the hydrogen bond structure and stability of metal complexes within DESs. This reportedly minimize potentially costly wastewater treatment following recycling.81
3.1.3 Considering hydrophobic DES.
Most reported findings on DES are for hydrophilic DES.82 Hydrophobic DESs (HDESs) were reported from 2015. The definition of hydrophilic DESs encompasses solvents capable of absorbing >0.2 w/w% of water, more viscous and less conductive; whilst hydrophobic ones have a low polarity and limited solubility in water.83,84 Ionic HDES is reportedly prepared via combining long-chain alkylammonium salt as HBA and hydrophobic organic solvent as HBD, whilst non-ionic HDESs are terpenoid-based that originated from menthol in combination with alkyl carboxylic acid with varying carbon chain lengths, from C1–C18.
Osch et al.85 apparently reported the first use of HDES containing decanoic acid (decA) and lidocaine for selective removal of transition metals from water. Ribeiro et al.86 reported the hydrophobic characteristic of DL-menthol and carboxylic acids. Significant research focussing on developing new HDESs and practical potential has been reported since.85 HDESs are however mainly used in solvent extraction to recover metals from solution. For example, Schaeffer et al.87 reported that the thymol-capric acid HDES selectively separates Cu2+ from Co2+ and Ni2+ in mildly acidic solutions. Chen et al.88 reported DES containing tetrabutylammonium chloride (TBAC) and oleic acid in extracting Li+ from Li2CO3 solution, with the highest efficiency of 76.8% by using 1
:
1 O/A, 70% TBP and 1.5 mol L−1 ammonia. A practical finding was HDES consists of TOPO and decA (1
:
1) that was highly efficient in leaching of 93.0% Li and 90.6% Co from LCO aided via addition of 0.1 M ascorbic acid (AsA, VC) and 2.5% water, followed by selective recovery by oxalic acid in aqueous and precipitation phase as Li2+ and cobalt oxalate, respectively, with high purity >99%.89 The HDES was readily regenerated without significant decomposition and versatility to both LCO and NCM reportedly made it more advantageous than hydrophilic DES in battery recycling. However, because of the strong effect of HBD and HBA on the hydrophobicity of the HDES, the components need to be carefully selected. Significantly, lidocaine as HBA was not recommended because of the large solubility of the protonated lidocaine in water that requires additional wastewater treatment.90,91 Recently, Paul et al.92 reported DFT to determine the formation and stability mechanism(s) for the hydrophobic DESs incorporating DL-menthol as HBD and selected carboxylic acids as HBA. The robustness of these DESs is attributed to a highly-interconnected network of hydrogen bonding and dispersion interactions. It is reported that longer chain fatty acids with 8–12 carbon are more chemically stable and less reactive than shorter chains (C 1–6) because of the stronger van der Waals interactions.
It is concluded that hydrophobic DESs have practical potential for application to recycling of LIBs, however, additional research is needed to design green and efficient hydrophobic DESs. This will involve identifying suitable components with hydrophobic properties, determining optimal eutectic composition, optimizing HDES properties, and establishing performance of HDES in the recycling.
3.1.4 Combining DES with another reagent/pretreatment.
Leaching selectivity is an advantage of DESs that is established either via adjusting HBA and HBD components, controlling water content or considering hydrophobic DES. Importantly, combination of DESs with another reagent or pretreatment has reportedly increased leaching selectivity of metal resources. Chen et al.93 reportedly proposed a two-step leaching system using formic acid (FA), (1) to selectively remove 99.8% Li at 90 °C for 12 h, followed by, (2) ChCl–FA DES leaching of 99.0% Co or Mn from the LCO or LMO (lithium manganese oxide) residue at 70 °C for 12 h. The design reportedly originates from excellent leaching selectivity of formic acid to Li due to the insolubility of Co(HCOO)2·2H2O and Mn(HCOO)2·2H2O. Both Li and Co in the separate leachate are recovered as Li2CO3 and CoCO3 products via adding saturated Na2CO3 under suitable conditions. Luo et al.94 reported a DES of ChCl–OA in leaching of Li and Co from LCO cathode at 110 °C for 2.5 h. Water was added to selectively separate Co from Li by precipitating Co as CoC2O4·2H2O. This combination of DES leaching and water addition was also reportedly applicable to NCM523 via coprecipitation of (Ni0.5Co0.2Mn0.3)C2O4·2H2O. Wang et al.95 reportedly designed a combination of ChCl–OAD DES with UV irradiation to recycle spent LiFePO4 cathodes. Initially, 95.3% Li and 85.2% Fe are leached into solution by DES at 106 °C for 110 min, and Fe then recovered in the form of FeC2O4·2H2O via irradiation under UV light for 8 h. The residual leachate was heated and reacted with NaOH to obtain Li3PO4. Tang et al.96 proposed a combination of 1ChCl–8EG with ozone in selective leaching of ≥92.2% Li from spent LiFePO4 at 40 °C for 6 h, with ≤1.6% Fe impurity. The leachate was heated at 150 °C for 0.5 h to obtain a pure Li solution, followed by vacuum distillation and calcination to produce LiCl.
It is concluded that design for DESs for LIBs recycling exhibits significant practical potential for efficient and sustainable processes. By carefully selecting HBD and HBA components with molar ratio, incorporating modifiers, controlling water content, exploring hydrophobic DESs, and combining with other reagent or pretreatment, significant advancements is likely in recycling with efficiency and selectivity. However, more research and development are required to fully unlock the benefits of DESs in terms of efficiency, sustainability, and environmental impact for practical recycling.
3.2 Leaching of metal oxides from spent LIBs
To maximize leaching efficiency of LIBs using a DES, optimization of leaching conditions including, molar ratio of HBD/HBA, water content, leaching temperature and duration, and solid to liquid ratio (S/L) need to be quantitatively established. The effects on leaching efficiency are shown as Fig. 3. External assistance including microwave can be applied to boost leaching.
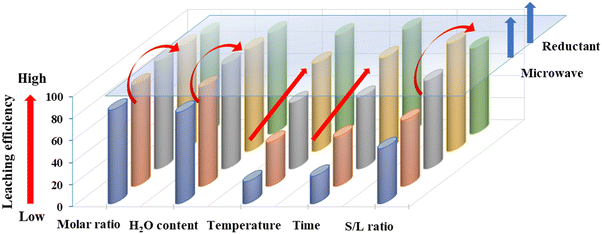 |
| Fig. 3 The effects of leaching parameters and external assistances on the DESs performance in leaching LIBs. (a) HBA/HBD molar ratio; (b) H2O content; (c) temperature; (d) leaching time; and (e) S/L ratio. | |
3.2.1 Leaching parameters.
The molar ratio of HBA to HBD in DES is important for efficient leaching because too high or too low ratio negatively affects leaching. Luo et al.97 found that metal leaching efficiency for NCM increased initially then decreased with increased ratio of BeCl to EG, and the optimal ratio was 1
:
5. Tang et al.98 reported a binary phase diagram for DESs with selected molar ratios of EG to SAD, and concluded that, more than 8
:
1 is required for successful formation of a DES. In determining the impact on leaching efficiency of LCO, it was found that constant leaching efficiency can be maintained with an increasing ratio from 10
:
1 to 12
:
1. This is because of the presence of carboxyl and sulfonic acid groups that provided sufficient protons and coordination constituents, respectively. However, subsequent decrease of efficiency was reported beyond a ratio of 16
:
1, because of reduced amounts of SAD.
For both hydrophilic and hydrophobic DESs, water content is important for leaching via maintaining viscosity of DES for improved DES characteristics and mass transfer. Additionally, water molecules generate hydrogen ions that disrupt the layered structure of the cathode, leading to the release of metal ions. Hammond et al.99 reportedly concluded that most DES maintain unique properties and functionality when water is added in quantities <42%. Roldán-Ruiz et al.42 compared DESs of ChCl and PSA with different water molar ratios for LCO dissolution, and reported that ChCl–PSA–2H2O with 1
:
2
:
1 exhibits highest leaching efficiency. Lu et al.77 reported the impact of water content in three (3) different DESs like ChCl–PSA, ChCl–Mal, and ChCl–PSA–Mal, and found that leaching efficiency decreased with increasing water content because the competition of water with DES molecules that weaken the DES affinity for the metal ions in LCO. Hanada and Goto89 reported the HDES for HBTA/TOPO with AsA in the leaching of LCO. Water addition significantly boosted Li and Co dissolution, which was reportedly ascribed to the water involvement in the reduction of Co(III) to Co(II) with the assistance of AsA.
Temperature and time are important parameters that significantly affect leaching between DES and LIBs. Higher temperature is favourable for metal extraction because of reduced viscosity and increased reaction kinetics. However, high temperature can lead to dissolution of impurity products and rapid volatilization of the solvents, which lowers L/S ratio and impedes complete reaction. Tran et al.39 reported that 1ChCl–2EG was highly practical for dissolving 99.3% of Co from spent LCO, and that, the ‘darkness’ of the eutectic solution was linearly correlated with Co concentration. The colour reportedly changed from transparent- to dark-blue when increasing temperature from 25 to 220 °C for 24 h, evidencing enhanced Co leaching from tetrachlorocobaltate(II) anion ([CoCl4]2−). Extending leaching time allows for more interactions, however a maximal is reached.81 Chen et al.100 reportedly investigated the influence of time on Co extraction from LCO in DESs. They found dissolution within 24 h as demonstrated by the increased Co concentration and deepened colour change from transparent to blue and finally black. Huang et al.101 reported the impact of leaching temperature and time (90 to 150 °C, 0.5 to 16 h) in a ternary ChCl–SA–EG DES, and found the leaching solution became darker with both increasing.
When the solid–liquid ratio is high with disproportionate amount of solid samples, the system becomes highly viscous, making it difficult to stir and ensure sufficient contact between spent LIBs and DES resulting in low leaching efficiency. Also, an ‘excess’ DES can result in wastage of reagents and restrict metal separation. Liu et al.102 studied the impact of the L/S ratio on leaching efficiency of Li and Co and found a (general) decreasing trend as DES amount increased from 3 to 9 g.
3.2.2 Boosted leaching via external assistances.
To increase dissolution performance of DESs, external assistances including, addition of extra reductants and microwave exposure have been reported. Peeters et al.41 reported a solvo-metallurgical recovery of Li and Co from spent LCO via a DES (2ChCl–1CA). They found that, (1) addition of Al and Cu current collectors as reducing agents significantly improved leaching efficiency from <50% to 90% in the presence of 12% Cu and 24% Al, and (2) the co-existence of Al and Cu exhibited higher efficiency compared with individual components. Cu metal reportedly increased leaching by reducing Co(III) to Co(II) and being oxidized to Cu(I), and; Cu(I/II) complexes were reduced by metallic Al to metallic Cu through cementation. Yan et al.55 reported the leaching of LCO and four (4) NCMs using a DES of GUC-LA, and found that the addition of 1 wt% VC improved the Li and Co leaching efficiency from LCO. However, NCMs exhibited no dependence on VC reductivity in DES due to the high solubility of Ni2+ and instability of Mn4+. These findings confirmed that the VC presence boosts ability of the system to dissolve metals with higher oxidation states. Xu et al.103 reported microwave assistance for recycling of LMO using a DES (1ChCl–1OAD). Both Li and Mn leaching efficiency reached 96% at a significantly shortened time of 15 min at a 1200 W microwave power and 2450 MHz frequency, which significantly contrasted with traditional leaching methods that took several days. Zhu et al.104 reported microwave assisted DES (ChCl–EG–urea/lactic acid) leaching of Li and Co from LCO, and concluded that this reduced leaching temperature from 105 °C, and >90% of Li and Co were leached in just 4 min and 160 W. The electric field generated by microwaves induces a dipole moment in the active components, leading to an ultra-fast leaching performance.
External assistances therefore improve reaction kinetics, reduce leaching time, lower leaching temperature, and increase overall efficiency of leaching.
3.2.3 Leaching mechanism.
The leaching mechanism remains poorly understood. However, it appears the mechanism is related to proton activity and reductivity of DESs, and coordination of metal intermediates, Fig. 4. Because of the high valence metal oxides contained in the waste batteries, reducibility is a more dominant parameter than is acidity and coordination, i.e. reducibility > acidity > coordination.
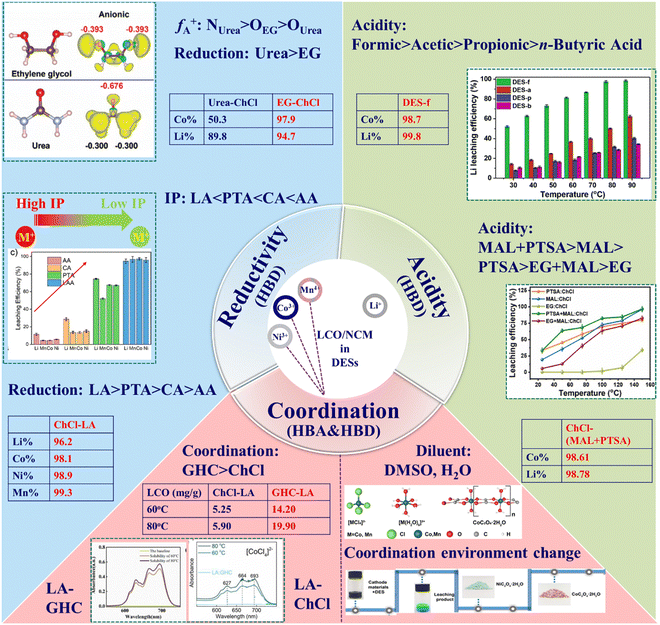 |
| Fig. 4 Leaching mechanism for DESs as a complex interaction of reductivity, acidity, and complexation. | |
Reducibility of DESs is the capability to convert high valence transition metals to a low valence state for ready dissolution, which is (mainly) dependent on HBD. Wang et al.40 compared the reducibility of urea and EG by Fukui function computation and CV testing. They evidenced that urea exhibited stronger reductivity than EG. Applying ChCl–urea DES at 180 °C for 12 h, Co and Li leaching efficiency from LCO was 97.9 and 94.7%, respectively. These findings were greater than for the leaching efficiency exhibited by ChCl–EG DES under harsher conditions, >24 h, 220 °C, as reported by Tran et al.,39 with 90% Co dissolved. It was also confirmed that the increased temperature from 140 to 180 °C boosted ion activities of ChCl–urea DES and promoted reduction, resulting in higher leaching efficiency for Li from 61.2 to 94.7%.40 Hua et al.50 reportedly investigated reduction ability of selected organic acids and inorganic acids based on IP computation with CV testing. The organic acids including AcA, CA, PSA and L-AsA exhibit a lower IP than inorganic ones such as HCl, HNO3, H2SO4, and H3PO4. Amongst the organic acids, the sequence of decreasing IP was, namely, AcA, CA, PTA, and with L-AsA. Reducibility was reportedly negatively related to IP, with L-AsA exhibiting greatest reductivity and AcA lowest. By mixing each of these four (4) organic acids with ChCl and water to synthesize DES, L-AsA based DES exhibited greatest leaching efficiency. These findings therefore confirmed that DES with stronger reducibility generates boosted leaching and/or requires milder conditions for dissolving metal oxides from LIBs.
The acidity of DESs is sourced from HBD, because H+ acts as an oxygen acceptor to dissolve metal oxides via cleaving their bonds.105 Chen et al.43 reportedly prepared acidic DESs by mixing ChCl with formic acid, acetic acid, propionic acid, n-butyric acid, at a molar ratio of 1
:
2, to leach LCO. Li and Co leaching efficiencies exhibited a decreasing order, DES-f, DES-a, DES-p, and DES-b. This finding of order is coincidental with reduced acidity of HBD in the DESs with increasing carbon chain, evidencing the importance of HBD acidity in leaching. Lu et al.77 reported a comparison of several DESs by varying HBDs with ChCl, and found that MAL and PSA exhibited better performance than urea and EG. The combination of MAL and PSA with ChCl (ChCl–MAL–PSA) however reportedly exhibited better leaching performance for Co and Li than using either acid as an individual HBD. This boost was attributed to the stronger acidity of MAL–PSA combination, leading to increased solubility of LIBs in ternary DESs compared with binary DESs. Recently Yan et al.55 reported that GUC based DES exhibited higher acidity compared with DES based on ChCl, and that LA is more acidic than GA when used as HBD for both types of DES. Amongst the four DESs, Li and Co leaching efficiencies increased with increasing acidity. Significantly, improving acidity of GUC-LA via addition of 1% VC improved LCO dissolution. Importantly it was found that acidity had no apparently quantitative relationship with leaching efficiency as reductivity, especially when there were sufficient hydrogen ions to leach metals, or the HBD had strong complexing ability.53
The coordination ability of DESs is to immobilize low valence metal ions such as Co, Ni or Mn from the solids to soluble form to the solution; it is related to both HBA and HBD. This is because oxygen in both HBA and HBD tends to be ligand as electron-donating atom, and is more likely to coordinate with metals as favourable central atom. Tian et al.106 reportedly compared ChCl–LA and GHC–LA performance in leaching of LCO under the same conditions with same amount of Cl−, and GHC–LA DES exhibited better performance. This finding they attributed to the stronger coordination ability of Cl− in GHC than in ChCl, resulting in more stable delocalized π-bonds and a greater likelihood of coordination with Co3+. The different coordination of DESs with metal cations provides practical opportunity to achieve leaching selectivity. Chang et al.79 reported selective separation of Ni and Co via regulation of coordination environment of these metal ions through diluting DES (1ChCl–1OAD) leachate with d-DMSO and water as diluent, respectively. By using DMSO as a diluent, nickel oxalate dihydrate was obtained and [Co/MnCl4]2− remained in the leachate. Subsequent filtration and water addition to the leachate allowed for precipitation of cobalt oxalate dihydrate. It is concluded that this finding has implications for practical, industrial application, including control of solubility of metal compounds in DES by dilution to impact coordination properties.
The leaching mechanism for DESs as dependent on acidity, reductivity and coordination has not been widely reported. Tang et al.98 proposed a new DES (12EG-1SAD) for dissolving LCO and NCM, where, initially, H+ (SA) leaches Li+ out of LCO and produces solid phase intermediate H-CoO2. The Co leaching encompasses a number of reaction pathways with competing behaviours between reduction and coordination. Tang et al.107 established an in situ separation of Li and Co from LCO in the DESs system (EG-OAD). This was composed of, extraction of Li+ ions by H+ ion substitution (OAD) together with H-CoO2 exposure, oxalic acid coordination with Co3+ ions to generate [Co(C2O4)3]3−, followed by EG reduction of Co3+ by DESs to form a final CoC2O4 solid. Luo et al.108 reported introduction of a natural DES (1BeCl–3CA–30% H2O) for leaching of NCM. Effectiveness was attributed to a synergistic effect(s) of strong coordination (Cl− from BeCl), acidity (ionized H+ from both BeCl and CA) and reducing ability (CA). The leaching mechanism is:
|  | (9) |
|  | (10) |
| Complexation Me2+ + Cl− → [MeCl4]2− | (11) |
The shrinking core model is a widely reported kinetic model that describes leaching of solid materials in a liquid phase that is suitable for kinetics of dissolution in DESs. The model assumes that leaching involves dissolution of the solid material from its surface into the liquid phase, and; that rate is governed by the chemical reaction at the surface of the solid, or by the diffusion of the reaction products away from the surface.109,110
3.3 Metal recovery and DES reuse
Following DES leaching of the LIBs, the majority of valuable metal(s) are transferred into the leachate. A final recovery of products and DES recyclability are important in practical application. Chemical precipitation and electrodeposition are two (2) widely reported methods. The coprecipitation of Ni, Co and Mn is (typically) conducted prior to Li. Precipitation agents including, NaOH,40 Na2CO3, and H2C2O4 are commonly reported, with H2C2O4 as more effective because of high selectivity between complexation and precipitation, better crystallinity of final product, no introduction of impurities and more ready regeneration of DES. With electrodeposition, the metal ions are deposited on a substrate under controlled electrochemical conditions, and; regenerated DES this way is reportedly purer than that from precipitation. Significantly, whilst solvent extraction is commonly used in traditional hydrometallurgy, there have been no reports of its individual use in DES leachate treatment for metal recovery from LIBs.
3.3.1 Chemical precipitation.
In the DES using oxalic acid as HBD, chemical precipitation can reportedly self-selectively separate transaction metals from Li without adding Na2CO3, or adjusting pH. Importantly, the system has wide applicability across major types of retired batteries, including LCO,94,111 NCM,107 LMO,112 and LFP.113 The transition metals are obtained in the form of (Co, Ni, Mn)C2O4·2H2O, which was roasted further to produce (Co, Ni, Mn)3O4 as a precursor for regenerating cathode material in battery assembly, which exhibited satisfactory stability in electrochemical tests. Li however is precipitated as LiHC2O4·4H2O, or Li2C2O4 for use as a precursor. In each cycle, because of the breakdown of hydrogen bonds in the DES caused by OA consumption and formation of Cl3− by esterification reaction, the residual DES solvent exhibited insufficient reductivity for subsequent leaching. However, this can reportedly be compensated by adding equal amount of lost OA to the filtrate – the reported maximum cycling number is 15.107 The mass loss of OA in DES (5EG-1OAD) is ca. half that the 1ChCl–1OA leaching of 7 vs. 14.2%.112
For DESs with HBDs other than oxalic acid including, ChCl–2urea,40 2ChCl–LA,114 ChCl–LA,50 and GUC-LA-VC,55 oxalic acid is reportedly also used as a stripping reagent to separate transition metals from Li following leaching. The first step was coprecipitation of all transition metals or precipitation of individual metal by OA, followed by precipitation of Li+ as Li2CO339 or LiPO3114via Na2CO3 or H3PO4, respectively. In addition, solvent extraction combined with (co)precipitation is an alternative to prepare battery precursors. The leachate is subjected to multiple extraction and stripping stages to selectively separate dissolved metals based on pH or solubilities. The final DES is treated by precipitation to realize DES reuse including, 2ChCl–CA–35%H2O,41 ChCl–2EG44,115 and ChCl–OxA.79 However, this method is tedious requiring multiple times for extraction and stripping, and pH adjustment from acidic pH 3.5 (leachate) to alkaline environment at ≥11 (solvent extraction), and; then back to acidic pH 1.5 (stripping). The regenerated cathode material is reportedly used to assemble new batteries, which exhibited excellent electrochemical performance.
3.3.2 Electrochemical deposition.
Electrodeposition is carried out using DES leachate as the electrolytic bath with a typical 3-electrode configuration to plate transition metal ions including, successful Co recovery from LCO leachate dissolved by ChCl–2EG39 and ChCl–2urea.40,116 Following electrodeposition, the three electrodes are washed by deionized water and ethanol prior to drying, and Co is obtained as Co(OH)2 whilst DES leachate is recycled. The mass difference of the electrodes during deposition was determined as the amount of obtained product. Substrates choice applied potentials and operating temperatures need to be controlled to achieve good electrochemical performance. Landa-Castro et al.117 reported electrodeposition of the reline DES solution following cathode dissolution from nickel metal hydride (Ni–MH) batteries, and Ni–Co alloy was obtained.
Despite that electrodeposition is not widely reported for recovering lithium, energy intensive and more expensive than DES itself, it offers benefits such as fast recovery, ready metal collection and substrate regeneration, high purity of product, and no secondary pollution. It is an advantageous choice therefore, particularly for selectively recovering a specific metal. However, precipitation is favoured for its low cost, simplicity, convenience, and high efficiency for industrialization, however it might not precipitate all metals. Coprecipitation, currently reportedly used via addition of defined amounts of desired metal, result in targeted final product but accompanied with impurity. A combination of precipitation with solvent extraction offers both higher extraction and precipitation efficiency, prioritizing multicomponent battery types. Nevertheless, this might be a basis for innovation with simplicity and flexibility to recover metals and regenerate DESs. A summary of the above discussed literature is presented as Table 2.
Table 2 Summary of LIBs recycling using different systems of DESs for metals recovery
DES types by HBA |
DES components |
Cathode material |
Reductant |
M
HBA/HBD
|
m
cat/mDES (g g−1) |
Temperature (°C) |
Time (h) |
Stirring rate (rpm) |
Leaching efficiency (%) |
DES recyclability |
Ref. |
Li |
Co |
Ni |
Mn |
Note: ChCl = choline chloride; EG = ethylene glycol; PSA = p-toluene sulfonic acid (sulfonic acid); OA = oxalic acid; OAD = oxalic acid dihydrate; CA = citric acid; AsA = ascorbic acid; FA = formic acid; PEG = polyethylene glycol 200; phytic acid = PHA; SAD = sulfosalicylic acid dihydrate; SA = succinic acid; GHC = guanidine hydrochloride; LA = lactic acid; L-(+)-tartaric acid = TA. |
ChCl type DES |
ChCl–EG |
LCO |
— |
1 : 2 |
0.1/5 |
220 |
24 |
|
94 |
90 |
— |
— |
Electrodeposition |
39
|
NCM |
|
|
0.2/50 |
180 |
|
|
71 |
32 |
7 |
60 |
|
|
NCM |
|
|
— |
180 |
24 |
— |
91.63 |
92.52 |
94.92 |
95.47 |
— |
115
|
NCM |
— |
|
0.5/25 |
180 |
24 |
— |
— |
— |
10 |
90 |
A partial make-up |
44
|
ChCl–urea |
LCO |
— |
1 : 2 |
1/50 |
180 |
12 |
125 |
94.7 |
97.9 |
— |
— |
— |
40
|
ChCl–PSA–H2O |
LCO |
— |
1 : 1 |
60 g L−1 |
90 |
0.25 |
— |
100 |
100 |
— |
— |
— |
42
|
ChCl–OA |
LCO |
— |
1 : 1 |
2/30 |
90 |
2 |
|
∼100 |
∼100 |
— |
— |
Water evaporation |
47
|
LCO |
— |
1 : 1 |
0.4/20 |
110 |
2.5 |
|
>99 |
0.14 |
— |
— |
Water evaporation and OA addition |
94
|
LCO |
— |
1 : 1 |
1/50 |
120 |
12 |
— |
96.1 |
96.3 |
— |
— |
OA addition |
112
|
ChCl–OAD |
NCM811;NCM111; NCM523;NCM622 |
— |
|
1/20 |
120 |
10 |
|
— |
95.1 |
99.1 |
95.3 |
— |
79
|
ChCl–CA/malic/Malonic |
LCO |
12 wt% Al |
2 : 1 |
1/50 |
40 |
1 |
— |
93 |
98 |
— |
— |
— |
41
|
|
24 wt% Cu |
|
|
|
|
|
|
|
|
|
|
|
|
35 wt% H2O |
|
|
|
|
|
|
|
|
|
|
|
ChCl–LA–H2O |
NCM111 |
— |
2 : 1 |
0.1/2.5 |
50 |
1 |
— |
96.2 |
98.1 |
98.9 |
99.3 |
Water and ethanol addition and evaporation |
50
|
ChCl–FA |
LCO |
— |
1 : 2 |
1/50 |
70 |
12 |
— |
99.8 |
99.1 |
— |
— |
— |
93
|
LCO |
— |
1 : 2 |
0.1/5.0 |
70 |
1/6 |
— |
100 |
>99 |
— |
— |
— |
102
|
ChCl–LA |
LMO |
— |
2 : 1 |
— |
70 |
10 |
— |
∼100 |
— |
— |
∼100 |
— |
114
|
LNMO |
|
|
|
100 |
5 |
|
75 |
— |
95 |
∼100 |
|
|
ChCl–TA–H2O |
NCM111 |
— |
1 : 1 |
0.1/5 |
70 |
12 |
150 |
96 |
97.1 |
98 |
96.7 |
Distillation of ethanol |
81
|
LCO |
|
|
|
80 |
24 |
|
∼100 |
∼100 |
— |
— |
|
|
ChCl–SA |
NCM |
— |
1 : 2 |
90 mL g−1 |
100 |
4/3 |
— |
97.7 |
97.0 |
96.4 |
93.0 |
— |
74
|
|
BeCl type DES |
BeCl–EG |
NCM |
|
1 : 5 |
0.5/20 |
140 |
1/6 |
200 |
99.5 |
99.6 |
99.7 |
99.1 |
— |
97
|
BeCl–CA–30% H2O |
NCM |
— |
1 : 3 |
0.4/20 |
80 |
0.5 |
— |
99.8 |
98.8 |
99.1 |
99.2 |
Heating of water |
108
|
|
Alcohol type DES-acidic |
PEG–thiourea |
LCO |
— |
2 : 1 |
0.1/5 |
80 |
24 |
— |
— |
15.1 |
— |
— |
— |
100
|
PEG–PSA |
LCO |
— |
1 : 1 |
0.1/5 |
80 |
24 |
— |
85.7 |
70.4 |
— |
— |
— |
153
|
PEG–AA |
LCO |
— |
6 : 1 |
0.1/30 |
80 |
24 |
— |
44.6 |
55.4 |
— |
— |
— |
154
|
PEG–PHA |
LCO |
— |
1 : 1 |
0.1/5 |
80 |
24 |
— |
96.9 |
98.7 |
— |
— |
— |
155
|
EG-SAD |
LCO |
— |
12 : 1 |
40 g L−1 |
110 |
6 |
60 |
98.3 |
93.5 |
— |
— |
— |
98
|
NCM1.7/8.5/1 |
|
|
|
|
|
|
100 |
94.8 |
99.1 |
100 |
|
|
EG-OAD |
LCO |
— |
5 : 1 |
16 g L−1 |
90 |
12 |
900 |
94.4 |
0 |
0 |
0 |
Addition of OAD |
107
|
NCM1.7/8.5/1 |
|
|
|
|
|
|
94.1 |
0 |
0 |
0 |
|
|
EG–TA |
LCO |
— |
5 : 1 |
20 g L−1 |
120 |
12 |
60 |
98.34 |
∼0 |
— |
— |
— |
147
|
Li3.2Ni2.4Co1.0Mn1.4O8.3 |
|
|
|
|
|
|
98.86 |
1.92 |
3.62 |
3.03 |
|
|
|
GUC based DES |
GUC–LA |
LCO |
1 wt% VC |
1 : 2 |
0.1/5 |
50 |
24/6 |
180/400 |
97 |
97 |
— |
— |
Supplement reducing agent |
55
|
NCM111 |
|
|
|
|
|
|
100 |
97 |
95 |
96 |
|
|
NCM523 |
|
|
|
|
|
|
100 |
89 |
87 |
85 |
|
|
NCM622 |
|
|
|
|
|
|
100 |
95 |
93 |
93 |
|
|
NCM811 |
|
|
|
|
|
|
100 |
93 |
100 |
100 |
|
|
GHC–LA |
LCO |
— |
|
— |
80 |
24 |
— |
99.5 |
99.5 |
— |
— |
Addition of oxalic acid |
106
|
4. Commercialization opportunities and challenges of DESs application in cathode recycling from LIBs
The recycling of spent LIBs cathodes using DESs appears practical for commercialization with potential for sustainable metal recovery because of advantages in metal selectivity, solvent recyclability and wide applicability to all cathode types. Whilst in an initial stage, the DES recycling exhibits practically promising outcomes and contributes significantly to a growing demand for critical metal resources. Therefore, it is important to consider how DES recycling can be developed for industry-scale.
To assess commercial relevance a critical review of patented DES processes was determined via a search on worldwide.espacenet.com, using ‘deep eutectic solvent’, ‘metal recovery’ and ‘lithium ion battery’ sorted by, ‘↑Priority date’ search terms. The earliest was from 2020, by Tran et al. (US20200399737A1).118 Following, some 20 patents have been granted with 19 from China. Taking a Japanese patent for example, Goto and Hanada (WO2023013714A1) proposed a metal recovery device using a hydrophobic DES.119 Significantly most are filed by academic institutions with a focus on laboratory-scale.
Despite an apparent absence of commercial-scale application, pilot projects are reported from collaboration between academic institutions and battery companies. For example, ‘Descycle’ leveraged a patented DES technology (not yet filed) and have announced a laboratory-scale e-waste recycling plant and a demonstrator-plant at Wilton on Teesside (UK) in late 2023 and, a commercial-scale plant in 2024 targeting ca. 5000 tonnes of waste.120 Additionally, Abbott et al. (2019)121 reported large-scale extraction of Zn and Pb from electric-arc-furnace-dust using a DES of ChCl–EG-urea, and; TECNALIA report progress toward pre-commercial scale of ca. 50 tonnes of platinum group metals (PGMs) recovery from spent autocatalysts.122
Through capitalizing on similarities with traditional hydrometallurgy, a commercialization recycling can be devised, starting from use of DESs, Fig. 5. However, there remain commercial challenges (CC) that will need to be addressed before application.
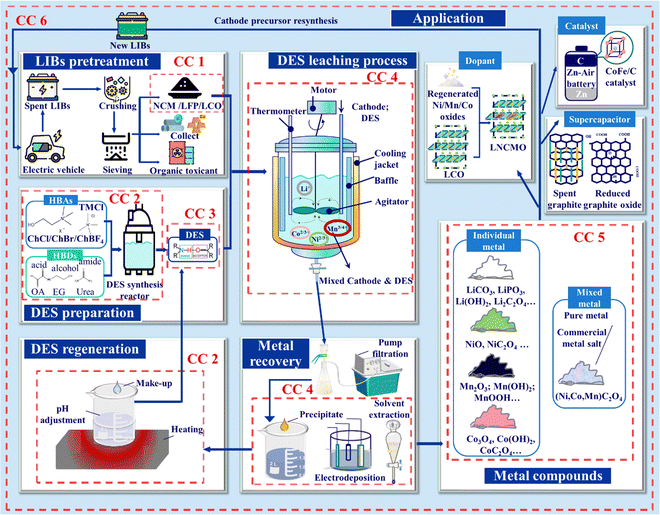 |
| Fig. 5 Schematic for commercialization recycling with DESs including, LIBs pretreatment, DES preparation, leaching, metal recovery, DES regeneration, product of metal compounds and post-application(s). | |
(CC 1) Variation in LIBs cathode materials
LIBs cathode materials used in EVs have various compositions including, LCO, LMO, LFP, NCA (lithium nickel cobalt aluminium oxide) and NCM,123–125 and, mixed cathode materials when various battery chemistries are collected for recycling.126 Amongst these LMO exists in different oxide forms, whilst NCM varies in Ni concentration, often shifting towards higher Ni content. Variability in cathode chemistry results in a waste stream containing different metal composition and morphology, which poses practical problems for recycling because of the limited flexibility of recycling.127 For example, a recycling method for LFP may not be applicable to NCM. To obviate this a ‘universal’ recycling scheme is necessary, focusing on recovering specific metals associated with each cathode chemistry. This requires implementation of efficient metal recovery tailored to the metals present in each battery type. The process involves optimizing DESs leaching and using a combination of techniques including, leaching, solvent extraction, precipitation, and electrochemical methods to enable recovery and purification of targeted metals from various cathode materials.
(CC 2) Designability, stability, and recyclability
Designability of DESs is important for efficient leaching of cathode chemistries present in different LIBs. Designing DESs includes selection of (appropriate) HBA and HBD components based on composition and structure characterization for better performance, evaluating DES formulations for hydrophobic, or hydrophilic properties, water addition to control solubility and address potential environmental risks, and; determining preparation methods.128 The designability of DESs directly affects long-term stability and recyclability of DESs that remain significant practical challenges on industrial-scale LIBs recycling. Ensuring the stability of DESs involves investigating structural changes and degradations that might occur during preparation, or leaching with cathode materials.129 Developing recycling methods for DESs is important for sustainability. Whilst back extraction via water addition, or pH adjustment is reportedly currently used for DES recycling, innovative approaches will be needed to boost DES recyclability.
(CC 3) Toxicity and biodegradability
For all DESs in various application, it is important to investigate toxicity and biodegradability to establish standardized regulations for human and environmental safety. Table S1 (ESI†) is a summary of toxicity and biodegradability levels for selected DESs that is a reference for proper selection of DESs in industrial applications.69,70 ChCl-based DESs exhibited an ascending toxicity sequence, namely, AcA < LA < GA < CA, whilst those ChCl-based DESs with glycerol, EG, 2-propanodiol or, urea as HBD are reportedly less toxic.130 According to the United Nations Globally Harmonized System of Classification and Labelling Chemicals, 30 min-EC50, values above 100 mg L−1 are considered harmless to the aquatic environment. It can be concluded from Table S1 (ESI†) therefore that alcohols are least toxic, followed by amides, and; organic acids are more toxic except for AcA.131 When comparing HBAs, ChCl-based DESs exhibit lower toxicity than N,N-diethylammonium chloride-based DESs.132 Additionally, the molar ratio of HBA to HBD and water content significantly influence toxicity.68 HBD and HBA can ‘indicate’ biodegradability but not predict toxicity of final DESs.75,133
(CC 4) Selective separation of transition metals and prioritized recovery of Li
Selective recovery of spent LIBs cathode materials is a significant practical challenge for commercial applications. The similar chemical properties for transition metals Co, Ni and Mn impede separation. Present coprecipitation for synthesis of new cathode materials has two (2) practical problems, (1) precise calculation of metal concentrations is inefficient and costly; and (2) purity of co-precipitated precursor is not predictably certain for reuse. Reportedly research is directed to selective separation of individual metal such as Li, Co or Ni, however separation efficiency varied significantly depending on complex cathode chemistry and difficult-to-control conditions.134 This method for metal selectivity is appears practical for commercialization because of universal applicability to all types of cathode materials and ready control of product purity. However Li recovery that is (generally) carried out as a last-step via chemical precipitation, reportedly exhibits significant Li loss during the repeated separation and precipitation steps involved. Despite reported attempts to first dissolve Li from LCO112,135 and LFP136,137 using DES together with pretreatments, there are no inconclusive findings evidencing that more is needed to prioritize Li recovery from NCM, or mixed cathode materials. Table S2 (ESI†) presents a quantitative summary of progress on selective recovery of Li, Ni and Co from spent LIBs using DESs.
(CC 5) Impurities and low product purity
Current recycling LIBs remains apparently complex, particularly pretreatment steps involved in obtaining cathode materials.138 Impurities present during recycling reportedly have significant implications for quality and performance of regenerated products. Common impurities including, Cu and Fe can be a risk to safety and decrease performance and overall quality of recycled products, whilst Al as a dopant, leads to low specific capacity and reversible capacity.139,140 With good design DES can be used to maximize selectivity and minimize impurity. Traditional methods might however be adopted/adapted that include, solvent extraction, chemical precipitation, electrodeposition, ion exchange and electrolysis for removal of these impurity ions with DES.141–143 Significantly in leaching however, separating transition metals such as Ni and Co with high purity is practically difficult because of similar chemical properties. Implementation for impurity control will therefore become important to ensure quality and safety of the regenerated products. DES-recycled products have been used for new batteries without fully confirming their high quality, and reportedly exhibit a comparative poorer performance and lifespan.111 However with development(s) in quality control of regenerated precursors, performance and lifespan of batteries made from DES-recycled materials are expected to improve.144
(CC 6) Need for a comprehensive DES recycling process
Present designability for DES for safe, efficient, and sustainable use in recycling of LIBs cathode materials is poorly understood. The relationship(s) between HBD/HBA components and specific leaching mechanism(s) is not clear. Present reported findings on LIBs recycling using DESs is restricted to leaching where the valuable metals are extracted into solvent.74,81,145
Optimization of leaching to maximize metal leaching efficiency has not been reported systematically. Subsequent recovery of metals from leaching liquor is reportedly via regeneration of cathode materials for new production, creating a closed-recycling-loop. Significantly, there are no reported patented technologies specific for obtaining individual metal products. It will be necessary therefore to establish a well-defined process for design of DESs, pretreatment, leaching, extraction, purification, and recovery stages tailored for specific LIBs batteries.
There are a number of practical difficulties in scaling-up from laboratory- to commercial-scale including, increased complexity in materials handling, process control, impurity management over larger volume, sensitivity to market fluctuation, and quality inconsistency. A comprehensive approach therefore is needed to implement DES recycling at commercial-scale.
5. Conclusions and future perspectives
Development of recycling is important for sustainable battery and automotive industry. DESs are a ‘new’ generation of ‘green’ solvents with unique properties that are practically promising for LIBs recycling. Accurate establishment of properties contributes to a flexible design for, importantly, biocompatibility and efficient DESs and permit improved design over the current narrow range of formulations. This will be used for a more selective recovery of critical metals and ready regeneration of DESs.
A present obstacle to continuing development of DESs is in finding a balance between, (1) achieving a high metal leaching efficiency, (2) ensuring greenness of DES, and (3) promoting reusability of DES. However, despite this and practical prospects for green-recycling of spent LIBs, a number of problems and difficulties remain, namely:
5.1. Technical
The integration of DES leaching into existing industrial large-scale applications is practical because of its similarities with traditional hydrometallurgy – with the main difference being leaching reagents used. Several technical problems however to be addressed obviated for practical implementation of DES on industrial-scale including, (1) the synthesis and storage of most DESs require heating during synthesis, and the resulting high viscosity and poor fluidity often necessitates continuous heating in storage,37,93 (2) waste treatment is needed to handle toxic gases generated during leaching,79 (3) commonly used coprecipitation for recycling transition metals is limited given the range of LIBs, and (4) in large-scale production where types of spent cathode materials vary significantly, repeated measurement of concentration of each element is necessary to ensure accurate synthesized NCM precursor(s), however, this is presently not practical.
Although selective separation and recovery of transition metals via DES is technically practical, it is a problem because of the use of significant organic solvents and requirement for multiple separation-conditions. It will be necessary to accurately establish economic feasibility and sustainability of the recycling involving DES.
5.2. Environmental
DESs exhibit favourable environmental characteristics including, high biodegradability, low toxicity and superior recyclability to traditional hydrometallurgical reagents. These contribute to a reduction in generation of hazardous waste, making DES leaching a sustainable option. However, DES formulations including, ChCl–urea DES, potentially produce NH4 and, chlorine-based DES Cl2 during leaching.79,116 The transformation of metal products to salt precursors to new cathode materials via calcination can result in hazardous waste such as CO2 released from oxalate precipitation.146
Despite reported attempts to reuse recovered DESs (often multiple times), there are limitations on leaching efficiency.107,147 The characterization of recovered DESs has not been established, and possible oxidation of DES during leaching can reportedly generate toxic ketones.98,107 Therefore it will be important to establish quantitatively the relationship between DESs properties and metal leaching efficiency. Waste management development will be needed, together with scaling of recycling within a closed-loop system. This includes reducing any environmental impact with production and disposal of DES components and by-products. Quantitative practices established in hydrometallurgy could be applied.
Computation of green chemistry metrics will be used to establish sustainability of DES recycling. This will involve parameters including, percentage yield, percentage conversion, selectivity, stoichiometric factor, atom economy, reaction mass efficiency, optimum efficiency, and process mass intensity.148
5.3. Economic
Economics are important to practical application of recycling and includes, raw materials, investment(s), energy consumption and management of potential by-product(s) and wastes. Because DES preparation and storage do not require ‘special’ conditions a comparison of DES with other leaching agents is not a logical category one. Overriding in commercialization of DESs is the impact of significant price variation ranging from 2 to USD$50 per kg depending on component(s), molar ratio, water content and recyclability. An analysis of price, Table S3 (ESI†), evidences that (in most cases), synthesizing DES, such as ChCl–urea, is more cost-effective than using organic acids alone.149,150 Particular DESs synthesized with organic acids as HBDs are, likely, not inherently cost-effective, however this can be obviated and boosted with design in a closed-loop that allows for recyclability. For example, ChCl–OA DES (1
:
1 molar ratio) is priced at 6.06 USD$ per kg which is greater than oxalic acid at 3.75, however, DES will be more cost-effective when reused for 2 or more cycles. Recyclable systems therefore significantly boost overall economic viability for industry.
There have been few reported estimations however of the cost for developed DES recycling based on a 1 tonne LIBs cathodes and current prices for consumed DESs components.108 This is a major weakness in reported findings. It can however be compared with recycling using mineral acids149 or organic acids.137,151 Economic costs will vary depending on recycling scale, initially, this might be done for laboratory-scale. Cost for chemicals is likely to determine overall economics of DES recycling. To boost economics, it would might be desirable to use computer simulation such as EverBatt model reported by Argonne National Laboratories in 2019.152 There is however no consistent and quantitative framework for benchmarking different recycling at present. Overall, it will be necessary to design biocompatible DESs via utilizing natural components and incorporate water to reduce viscosity for higher leaching efficiency. These strategies contribute to the economic feasibility of using DES in the recycling of LIBs.
It is concluded that DESs permit green-recycling of spent LIB cathodes with recovery of important metal resources in practical commercialization because of significant technical advantages in metal selectivity, solvent recyclability and wide applicability to all cathode types. Findings will benefit researchers and manufacturers in design for a circular and environmentally benign battery recycling.
Author contributions
Jingxiu Wang: conceptualization, methodology, software, writing—original draft preparation. Yanqiu Lyv: writing—original draft preparation, validation, data analysis. Rong Zeng and Shilin Zhang: software, validation, data analysis. Kenneth Davey: writing—review and editing; Jianfeng Mao: supervision, writing—review and editing. Zaiping Guo: supervision, project administration, writing—review and editing, funding acquisition.
Conflicts of interest
There are no conflicts to declare.
Acknowledgements
This work is supported by the Australian Research Council (ARC) (FL210100050, FT230100598, IC230100042).
References
- H. Shih, C. Chiang, H. Lai, M. Hsiao, L. Chen and H. Ma, Energy, 2023, 128828 Search PubMed.
- W. Qiu, Q. Hao, S. H. K. Annamareddy, B. Bin Xu, Z. Guo and Q. Jiang, Eng. Sci., 2022, 20, 100–109 Search PubMed.
- The global electric vehicle market in 2020: statistics & forecasts, https://www.virta.global/global-electric-vehicle-market.
- M. Chen, X. Ma, B. Chen, R. Arsenault, P. Karlson, N. Simon and Y. Wang, Joule, 2019, 3, 2622–2646 Search PubMed.
- Z. Yang, H. Huang and F. Lin, Adv. Energy Mater., 2022, 12, 2200383 Search PubMed.
- A. Zeng, W. Chen, K. D. Rasmussen, X. Zhu, M. Lundhaug, D. B. Müller, J. Tan, J. K. Keiding, L. Liu, T. Dai, A. Wang and G. Liu, Nat. Commun., 2022, 13, 1341 Search PubMed.
- J. Dunn, M. Slattery, A. Kendall, H. Ambrose and S. Shen, Environ. Sci. Technol., 2021, 55, 5189–5198 Search PubMed.
- C. Xu, Q. Dai, L. Gaines, M. Hu, A. Tukker and B. Steubing, Commun. Mater., 2020, 1, 99 Search PubMed.
- L.-Y. Yu, K.-J. Wu and C.-H. He, Sep. Purif. Technol., 2022, 281, 119928 Search PubMed.
- A. Chitre, D. Freake, L. Lander, J. Edge and M. Titirici, Batteries Supercaps, 2020, 3, 1126–1136 Search PubMed.
- J. Mao, C. Ye, S. Zhang, F. Xie, R. Zeng, K. Davey, Z. Guo and S. Qiao, Energy Environ. Sci., 2022, 15, 2732–2752 Search PubMed.
- G. Harper, R. Sommerville, E. Kendrick, L. Driscoll, P. Slater, R. Stolkin, A. Walton, P. Christensen, O. Heidrich, S. Lambert, A. Abbott, K. Ryder, L. Gaines and P. Anderson, Nature, 2019, 575, 75–86 Search PubMed.
- R. E. Ciez and J. F. Whitacre, Nat. Sustain., 2019, 2, 148–156 Search PubMed.
- J. Hu, J. Zhang, H. Li, Y. Chen and C. Wang, J. Power Sources, 2017, 351, 192–199 Search PubMed.
- J. Zhang, J. Hu, W. Zhang, Y. Chen and C. Wang, J. Cleaner Prod., 2018, 204, 437–446 Search PubMed.
- C. Yang, J. Zhang, Z. Cao, Q. Jing, Y. Chen and C. Wang, ACS Sustainable Chem. Eng., 2020, 8, 15732–15739 Search PubMed.
- R. Golmohammadzadeh, F. Faraji and F. Rashchi, Resour., Conserv. Recycl., 2018, 136, 418–435 Search PubMed.
- Z. Liang, C. Cai, G. Peng, J. Hu, H. Hou, B. Liu, S. Liang, K. Xiao, S. Yuan and J. Yang, ACS Sustainable Chem. Eng., 2021, 9, 5750–5767 Search PubMed.
- Y. Yao, M. Zhu, Z. Zhao, B. Tong, Y. Fan and Z. Hua, ACS Sustainable Chem. Eng., 2018, 6, 13611–13627 Search PubMed.
- T. Or, S. W. D. Gourley, K. Kaliyappan, A. Yu and Z. Chen, Carbon Energy, 2020, 2, 6–43 Search PubMed.
- P. Xu, Q. Dai, H. Gao, H. Liu, M. Zhang, M. Li, Y. Chen, K. An, Y. S. Meng, P. Liu, Y. Li, J. S. Spangenberger, L. Gaines, J. Lu and Z. Chen, Joule, 2020, 4, 2609–2626 Search PubMed.
- Y. Zhao, X. Yuan, L. Jiang, J. Wen, H. Wang, R. Guan, J. Zhang and G. Zeng, Chem. Eng. J., 2020, 383, 123089 Search PubMed.
- J. Wu, M. Zheng, T. Liu, Y. Wang, Y. Liu, J. Nai, L. Zhang, S. Zhang and X. Tao, Energy Storage Mater., 2023, 54, 120–134 Search PubMed.
- Y. Chen and T. Mu, Green Chem. Eng., 2021, 2, 174–186 Search PubMed.
- B. B. Hansen, S. Spittle, B. Chen, D. Poe, Y. Zhang, J. M. Klein, A. Horton, L. Adhikari, T. Zelovich, B. W. Doherty, B. Gurkan, E. J. Maginn, A. Ragauskas, M. Dadmun, T. A. Zawodzinski, G. A. Baker, M. E. Tuckerman, R. F. Savinell and J. R. Sangoro, Chem. Rev., 2021, 121, 1232–1285 Search PubMed.
- Z. Yuan, H. Liu, W. F. Yong, Q. She and J. Esteban, Green Chem., 2022, 24, 1895–1929 Search PubMed.
- X. Chen, H. Ma, C. Luo and T. Zhou, J. Hazard. Mater., 2017, 326, 77–86 Search PubMed.
- K. H. Chan, J. Anawati, M. Malik and G. Azimi, ACS Sustainable Chem. Eng., 2021, 9, 4398–4410 Search PubMed.
- S. P. Barik, G. Prabaharan and L. Kumar, J. Cleaner Prod., 2017, 147, 37–43 Search PubMed.
- P. Meshram, A. Mishra, Abhilash and R. Sahu, Chemosphere, 2020, 242, 125291 Search PubMed.
- R. Golmohammadzadeh, F. Rashchi and E. Vahidi, Waste Manag., 2017, 64, 244–254 Search PubMed.
- B. Musariri, G. Akdogan, C. Dorfling and S. Bradshaw, Miner. Eng., 2019, 137, 108–117 Search PubMed.
- A. P. Abbott, G. Capper, D. L. Davies, R. K. Rasheed and V. Tambyrajah, Chem. Commun., 2003, 70–71 Search PubMed.
- A. Prabhune and R. Dey, J. Mol. Liq., 2023, 379, 121676 Search PubMed.
- J. Wu, Q. Liang, X. Yu, L. Qiu-Feng, L. Ma, X. Qin, G. Chen and B. Li, Adv. Funct. Mater., 2021, 31, 1–25 Search PubMed.
- Z. Wang, S. Li, T. Li, T. Hu and X. Ge, Mining, Metall. Explor., 2022, 39, 2149–2165 Search PubMed.
- A. Zhu, X. Bian, W. Han, D. Cao, Y. Wen, K. Zhu and S. Wang, Resour., Conserv. Recycl., 2023, 188, 106690 Search PubMed.
- A. P. Abbott, G. Capper, D. L. Davies, K. J. McKenzie and S. U. Obi, J. Chem. Eng. Data, 2006, 51, 1280–1282 Search PubMed.
- M. K. Tran, M. T. F. Rodrigues, K. Kato, G. Babu and P. M. Ajayan, Nat. Energy, 2019, 4, 339–345 Search PubMed.
- S. Wang, Z. Zhang, Z. Lu and Z. Xu, Green Chem., 2020, 22, 4473–4482 Search PubMed.
- N. Peeters, K. Binnemans and S. Riaño, Green Chem., 2020, 22, 4210–4221 Search PubMed.
- M. J. Roldán-Ruiz, M. L. Ferrer, M. C. Gutiérrez and F. Del Monte, ACS Sustainable Chem. Eng., 2020, 8, 5437–5445 Search PubMed.
- L. Chen, Y. Chao, X. Li, G. Zhou, Q. Lu, M. Hua, H. Li, X. Ni, P. Wu and W. Zhu, Green Chem., 2021, 23, 2177–2184 Search PubMed.
- P. G. Schiavi, P. Altimari, M. Branchi, R. Zanoni, G. Simonetti, M. A. Navarra and F. Pagnanelli, Chem. Eng. J., 2021, 417, 129249 Search PubMed.
- T. R. Sekharan, R. M. Chandira, S. Tamilvanan, S. C. Rajesh and B. S. Venkateswarlu, Biointerface Res. Appl. Chem., 2022, 12, 847–860 Search PubMed.
- T. El Achkar, H. Greige-Gerges and S. Fourmentin, Environ. Chem. Lett., 2021, 19, 3397–3408 Search PubMed.
- B. Li, Q. Li, Q. Wang, X. Yan, M. Shi and C. Wu, Phys. Chem. Chem. Phys., 2022, 24, 19029–19051 Search PubMed.
- D. O. Abranches, M. A. R. Martins, L. P. Silva, N. Schaeffer, S. P. Pinho and J. A. P. Coutinho, Chem. Commun., 2019, 55, 10253–10256 Search PubMed.
- D. O. Abranches and J. A. P. Coutinho, Curr. Opin. Green Sustain. Chem., 2022, 35, 100612 Search PubMed.
- Y. Hua, Y. Sun, F. Yan, S. Wang, Z. Xu, B. Zhao and Z. Zhang, Chem. Eng. J., 2022, 436, 133200 Search PubMed.
- R. Pucci and G. G. N. Angilella, Found. Chem., 2022, 24, 59–71 Search PubMed.
- A. Skulcova, A. Russ, M. Jablonsky and J. Sima, BioResources, 2019, 13, 5042–5051 Search PubMed.
- W. Chen, J. Jiang, X. Lan, X. Zhao, H. Mou and T. Mu, Green Chem., 2019, 21, 4748–4756 Search PubMed.
- W. Chen, X. Bai, Z. Xue, H. Mou, J. Chen, Z. Liu and T. Mu, New J. Chem., 2019, 43, 8804–8810 Search PubMed.
- Q. Yan, A. Ding, M. Li, C. Liu and C. Xiao, Energy Fuels, 2023, 37, 1216–1224 Search PubMed.
- I. M. Pateli, D. Thompson, S. S. M. Alabdullah, A. P. Abbott, G. R. T. Jenkin and J. M. Hartley, Green Chem., 2020, 22, 5476–5486 Search PubMed.
- R. Hao, J. He, L. Zhao and Y. Zhang, ChemistrySelect, 2017, 2, 7918–7924 Search PubMed.
- Y. Cui, C. Li, J. Yin, S. Li, Y. Jia and M. Bao, J. Mol. Liq., 2017, 236, 338–343 Search PubMed.
- J. K. U. Ling and K. Hadinoto, Int. J. Mol. Sci., 2022, 23, 3381 Search PubMed.
- C. Florindo, F. S. Oliveira, L. P. N. Rebelo, A. M. Fernandes and I. M. Marrucho, ACS Sustainable Chem. Eng., 2014, 2, 2416–2425 Search PubMed.
- I. J. Ferreira, F. Oliveira, A. R. Jesus, A. Paiva and A. R. C. Duarte, J. Mol. Liq., 2022, 362, 119675 Search PubMed.
- J. Torregrosa-Crespo, X. Marset, G. Guillena, D. J. Ramón and R. María Martínez-Espinosa, Sci. Total Environ., 2020, 704, 135382 Search PubMed.
- K. Radošević, M. Cvjetko Bubalo, V. Gaurina Srček, D. Grgas, T. Landeka Dragičević and R. I. Redovniković, Ecotoxicol. Environ. Saf., 2015, 112, 46–53 Search PubMed.
- Q. Wen, J. X. Chen, Y. L. Tang, J. Wang and Z. Yang, Chemosphere, 2015, 132, 63–69 Search PubMed.
- B. Y. Zhao, P. Xu, F. X. Yang, H. Wu, M. H. Zong and W. Y. Lou, ACS Sustainable Chem. Eng., 2015, 3, 2746–2755 Search PubMed.
- Y. Huang, F. Feng, J. Jiang, Y. Qiao, T. Wu, J. Voglmeir and Z. G. Chen, Food Chem., 2017, 221, 1400–1405 Search PubMed.
- G. M. Martínez, G. G. Townley and R. M. Martínez-Espinosa, Heliyon, 2022, 8, e12567 Search PubMed.
- D. Lapeña, D. Errazquin, L. Lomba, C. Lafuente and B. Giner, Environ. Sci. Pollut. Res., 2021, 28, 8812–8821 Search PubMed.
- M. Marchel, H. Cieśliński and G. Boczkaj, J. Hazard. Mater., 2022, 425, 127963 Search PubMed.
- I. Juneidi, M. Hayyan and M. A. Hashim, RSC Adv., 2015, 5, 83636–83647 Search PubMed.
- P. De Morais, F. Gonçalves, J. A. P. Coutinho and S. P. M. Ventura, ACS Sustainable Chem. Eng., 2015, 3, 3398–3404 Search PubMed.
- I. P. E. Macário, S. P. M. Ventura, J. L. Pereira, A. M. M. Gonçalves, J. A. P. Coutinho and F. J. M. Gonçalves, Ecotoxicol. Environ. Saf., 2018, 165, 597–602 Search PubMed.
- L. Corte, P. Rellini, L. Roscini, F. Fatichenti and G. Cardinali, Anal. Chim. Acta, 2010, 659, 258–265 Search PubMed.
- X. He, Y. Wen, X. Wang, Y. Cui, L. Li and H. Ma, Waste Manage., 2023, 157, 8–16 Search PubMed.
- Y. Li, J. Luo, S. Shan and Y. Cao, J. Mol. Liq., 2023, 370, 121044 Search PubMed.
- V. P. Cotroneo-Figueroa, N. F. Gajardo-Parra, P. López-Porfiri, Á. Leiva, M. Gonzalez-Miquel, J. M. Garrido and R. I. Canales, J. Mol. Liq., 2022, 345, 116986 Search PubMed.
- B. Lu, R. Du, G. Wang, Y. Wang, S. Dong, D. Zhou, S. Wang and C. Li, Environ. Res., 2022, 212, 113286 Search PubMed.
-
E. Ostlund, Degree project E in Chemistry, Uppsala University, 2020 Search PubMed.
- X. Chang, M. Fan, C. F. Gu, W. H. He, Q. Meng, L. J. Wan and Y. G. Guo, Angew. Chem., Int. Ed., 2022, 61, 1–8 Search PubMed.
- J. T. M. Amphlett and S. Choi, J. Mol. Liq., 2021, 332, 115845 Search PubMed.
- C. Ma, M. Svärd and K. Forsberg, Resour., Conserv. Recycl., 2022, 186, 106579 Search PubMed.
- A. K. Dwamena, Separations, 2019, 6, 1–15 Search PubMed.
- P. Makoś-Chełstowska, M. Kaykhaii, J. Płotka-Wasylka and M. de la Guardia, J. Mol. Liq., 2022, 365, 120158 Search PubMed.
- H. Kivelä, M. Salomäki, P. Vainikka, E. Mäkilä, F. Poletti, S. Ruggeri, F. Terzi and J. Lukkari, J. Phys. Chem. B, 2022, 126, 513–527 Search PubMed.
- D. J. G. P. Van Osch, L. F. Zubeir, A. Van Den Bruinhorst, M. A. A. Rocha and M. C. Kroon, Green Chem., 2015, 17, 4518–4521 Search PubMed.
- B. D. Ribeiro, C. Florindo, L. C. Iff, M. A. Z. Coelho and I. M. Marrucho, ACS Sustainable Chem. Eng., 2015, 3, 2469–2477 Search PubMed.
- N. Schaeffer, M. A. R. Martins, C. M. S. S. Neves, S. P. Pinho and J. A. P. Coutinho, Chem. Commun., 2018, 54, 8104–8107 Search PubMed.
- W. Chen, X. Li, L. Chen, G. Zhou, Q. Lu, Y. Huang, Y. Chao and W. Zhu, Chem. Eng. J., 2021, 420, 127648 Search PubMed.
- T. Hanada and M. Goto, Green Chem., 2022, 24, 5107–5115 Search PubMed.
- D. J. G. P. Van Osch, D. Parmentier, C. H. J. T. Dietz, A. Van Den Bruinhorst, R. Tuinier and M. C. Kroon, Chem. Commun., 2016, 52, 11987–11990 Search PubMed.
- G. Zante and M. Boltoeva, Sustain. Chem., 2020, 1, 238–255 Search PubMed.
- N. Paul, G. Harish and T. Banerjee, ACS Sustainable Chem. Eng., 2023, 11, 3539–3556 Search PubMed.
- L. Chen, Y. Chao, X. Li, G. Zhou, Q. Lu, M. Hua, H. Li, X. Ni, P. Wu and W. Zhu, Green Chem., 2021, 23, 2177–2184 Search PubMed.
- Y. Luo, L. Ou and C. Yin, Sci. Total Environ., 2023, 875, 162567 Search PubMed.
- C. Wang, H. Yang, C. Yang, Y. Liu, L. Bai and S. Yang, J. Mater. Cycles Waste Manage., 2023, 25, 2077–2086 Search PubMed.
- S. Tang, Z. Yang, M. Zhang and M. Guo, Environ. Res., 2023, 239, 117393 Search PubMed.
- Y. Luo, C. Yin, L. Ou and C. Zhang, Green Chem., 2022, 24, 6562–6570 Search PubMed.
- S. Tang, M. Zhang and M. Guo, ACS Sustainable Chem. Eng., 2022, 10, 975–985 Search PubMed.
- O. S. Hammond, D. T. Bowron and K. J. Edler, Angew. Chemie, 2017, 129, 9914–9917 Search PubMed.
- Y. Chen, Y. Lu, Z. Liu, L. Zhou, Z. Li, J. Jiang, L. Wei, P. Ren and T. Mu, ACS Sustainable Chem. Eng., 2020, 8, 11713–11720 Search PubMed.
- F. Huang, T. Li, X. Yan, Y. Xiong, X. Zhang, S. Lu, N. An, W. Huang, Q. Guo and X. Ge, ACS Omega, 2022, 7, 11452–11459 Search PubMed.
- M. Liu, W. Ma, X. Zhang, Z. Liang and Q. Zhao, Mater. Chem. Phys., 2022, 289, 126466 Search PubMed.
- Z. Xu, H. Shao, Q. Zhao and Z. Liang, JOM, 2021, 73, 2104–2110 Search PubMed.
- A. Zhu, X. Bian, W. Han, Y. Wen, K. Ye, G. Wang, J. Yan, D. Cao, K. Zhu and S. Wang, Waste Manage., 2023, 156, 139–147 Search PubMed.
- Y. Fan, Y. Kong, P. Jiang, G. Zhang, J. Cong, X. Shi, Y. Liu, P. Zhang, R. Zhang and Y. Huang, Chem. Eng. J., 2023, 463, 142278 Search PubMed.
- Y. Tian, W. Chen, B. Zhang, Y. Chen, R. Shi, S. Liu, Z. Zhang and T. Mu, ChemSusChem, 2022, 15, 1–8 Search PubMed.
- S. Tang, J. Feng, R. Su, M. Zhang and M. Guo, ACS Sustainable Chem. Eng., 2022, 10, 8423–8432 Search PubMed.
- Y. Luo, L. Ou and C. Yin, Waste Manage., 2023, 164, 1–8 Search PubMed.
- J. Wang, F. Faraji and A. Ghahreman, J. Ind. Eng. Chem., 2021, 104, 333–344 Search PubMed.
- M. Jafari, S. Z. Shafaie, H. Abdollahi and A. Entezari-Zarandi, Miner. Process. Extr. Metall. Rev., 2022, 00, 1–13 Search PubMed.
- T. Li, Y. Xiong, X. Yan, T. Hu, S. Jing, Z. Wang and X. Ge, J. Energy Chem., 2022, 72, 532–538 Search PubMed.
- Q. Lu, L. Chen, X. Li, Y. Chao, J. Sun, H. Ji and W. Zhu, ACS Sustainable Chem. Eng., 2021, 9, 13851–13861 Search PubMed.
- W. Ma, M. Liu, X. Zhang, Q. Zhao and Z. Liang, Energy Fuels, 2023, 37, 724–734 Search PubMed.
- R. Morina, D. Callegari, D. Merli, G. Alberti, P. Mustarelli and E. Quartarone, ChemSusChem, 2022, 15, e202102080 Search PubMed.
- K. Wang, T. Hu, P. Shi, Y. Min, J. Wu and Q. Xu, ACS Sustainable Chem. Eng., 2022, 10, 1149–1159 Search PubMed.
- H. Wang, M. Li, S. Garg, Y. Wu, M. Nazmi Idros, R. Hocking, H. Duan, S. Gao, A. J. Yago, L. Zhuang and T. E. Rufford, ChemSusChem, 2021, 14, 2972–2983 Search PubMed.
- M. Landa-Castro, J. Aldana-González, M. G. Montes de Oca-Yemha, M. Romero-Romo, E. M. Arce-Estrada and M. Palomar-Pardavé, J. Alloys Compd., 2020, 830, 1–9 Search PubMed.
-
M. K. Tran, M. T. F. Rodrigues, G. Babu, H. Gullapalli and P. M. Ajayan, US20200399737A1, 2020.
-
M. Goto and T. Hanada, WO2023013714A1, 2023.
-
Tom Keighley, Recycling innovators Descycle secure £4.9m investment ahead of North East plant plans, https://www.business-live.co.uk/technology/recycling-innovators-descycle-secure-49m-26776431.
- A. P. Abbott, J. Collins, I. Dalrymple, R. C. Harris, R. Mistry, F. Qiu, J. Scheirer and W. R. Wise, Aust. J. Chem., 2009, 62, 341–347 Search PubMed.
-
PEACOC, Pre-commercial pilot for the efficient recovery of Precious Metals from European end of life resources with novel low-cost technologies, 2023 Search PubMed.
- M. Li, C. Wang, K. Davey, J. Li, G. Li, S. Zhang, J. Mao and Z. Guo, SmartMat, 2023, 4, e1185 Search PubMed.
- Y. Fan, E. Olsson, G. Liang, Z. Wang, A. M. D’Angelo, B. Johannessen, L. Thomsen, B. Cowie, J. Li, F. Zhang, Y. Zhao, W. K. Pang, Q. Cai and Z. Guo, Angew. Chem., Int. Ed., 2023, 62, e202213806 Search PubMed.
- F. Duarte Castro, L. Cutaia and M. Vaccari, Resour., Conserv. Recycl., 2021, 169, 105522 Search PubMed.
- J. Wu, Y. Cao, H. Zhao, J. Mao and Z. Guo, Carbon Energy, 2019, 1, 57–76 Search PubMed.
- D. L. Thompson, J. M. Hartley, S. M. Lambert, M. Shiref, G. D. J. Harper, E. Kendrick, P. Anderson, K. S. Ryder, L. Gaines and A. P. Abbott, Green Chem., 2020, 22, 7585–7603 Search PubMed.
- M. Zhang, R. Tian, H. Han, K. Wu, B. Wang, Y. Liu, Y. Zhu, H. Lu and B. Liang, J. Cleaner Prod., 2022, 345, 131028 Search PubMed.
- N. Rodriguez Rodriguez, A. Van Den Bruinhorst, L. J. B. M. Kollau, M. C. Kroon and K. Binnemans, ACS Sustainable Chem. Eng., 2019, 7, 11521–11528 Search PubMed.
- M. Hayyan, C. Y. Looi, A. Hayyan, W. F. Wong and M. A. Hashim, PLoS One, 2015, 10, 1–18 Search PubMed.
- A. K. Halder and M. N. D. S. Cordeiro, ACS Sustainable Chem. Eng., 2019, 7, 10649–10660 Search PubMed.
- Y. P. Mbous, M. Hayyan, W. F. Wong, A. Hayyan, C. Y. Looi and M. A. Hashim, J. Phys. Chem. B, 2020, 124, 9086–9094 Search PubMed.
- L. Lomba, M. P. Ribate, E. Sangüesa, J. Concha, M. P. Garralaga, D. Errazquin, C. B. Garc and B. Giner, Appl. Sci., 2021, 11, 10061 Search PubMed.
- Y. Luo, L. Ou and C. Yin, J. Cleaner Prod., 2023, 396, 136552 Search PubMed.
- A. Verma, G. H. Johnson, D. R. Corbin and M. B. Shiflett, ACS Sustainable Chem. Eng., 2020, 8, 6100–6108 Search PubMed.
- G. Ji, X. Ou, R. Zhao, J. Zhang, J. Zou, P. Li, D. Peng, L. Ye, B. Zhang and D. He, Resour., Conserv. Recycl., 2021, 174, 105802 Search PubMed.
- H. Mahandra and A. Ghahreman, Resour., Conserv. Recycl., 2021, 175, 1–11 Search PubMed.
- A. K. Vinayak, Z. Xu, G. Li and X. Wang, Renewables, 2023, 1, 294–315 Search PubMed.
- J. Ren, R. Li, Y. Liu, Y. Cheng, D. Mu, R. Zheng, J. Liu and C. Dai, New J. Chem., 2017, 41, 10959–10965 Search PubMed.
- M. T. Islam and U. Iyer-Raniga, Recycling, 2022, 7, 33 Search PubMed.
- Y. Pranolo, W. Zhang and C. Y. Cheng, Hydrometallurgy, 2010, 102, 37–42 Search PubMed.
- G. Granata, E. Moscardini, F. Pagnanelli, F. Trabucco and L. Toro, J. Power Sources, 2012, 206, 393–401 Search PubMed.
- F. Guo, S. Nishihama and K. Yoshizuka, Environ. Technol., 2013, 34, 1307–1317 Search PubMed.
- Y. Luo, C. Yin and L. Ou, Sci. Total Environ., 2023, 902, 166095 Search PubMed.
- M. Wang, K. Liu, Z. Xu, S. Dutta, M. Valix, D. S. Alessi, L. Huang, J. B. Zimmerman and D. C. W. Tsang, Environ. Sci. Technol., 2023, 57, 3940–3950 Search PubMed.
- V. Verma, J. R. Joseph, R. Chaudhary and M. Srinivasan, J. Environ. Chem. Eng., 2023, 11, 110216 Search PubMed.
- Z. Yang, S. Tang, X. Huo, M. Guo and M. Zhang, Environ. Res., 2023, 233, 116337 Search PubMed.
- C. R. McElroy, A. Constantinou, L. C. Jones, L. Summerton and J. H. Clark, Green Chem., 2015, 17, 3111–3121 Search PubMed.
- L. Li, Y. Bian, X. Zhang, Q. Xue, E. Fan, F. Wu and R. Chen, J. Power Sources, 2018, 377, 70–79 Search PubMed.
- J. Peng, H. Xu, W. Wang, Y. Kong, Z. Su and B. Li, Ind. Crops Prod., 2021, 172, 114036 Search PubMed.
- Y. Yang, X. Meng, H. Cao, X. Lin, C. Liu, Y. Sun, Y. Zhang and Z. Sun, Green Chem., 2018, 20, 3121–3133 Search PubMed.
- Q. Dai, J. Spangenberger, S. Ahmed, L. Gaines, J. C. Kelly and M. Wang, Argonne Natl. Lab., 2019, 1–88 Search PubMed.
- Y. Chen, Y. Wang, Y. Bai, Y. Duan, B. Zhang, C. Liu, X. Sun, M. Feng and T. Mu, ACS Sustainable Chem. Eng., 2021, 9, 12940–12948 Search PubMed.
- Y. Chen, Y. Wang, Y. Bai, M. Feng, F. Zhou, Y. Lu, Y. Guo, Y. Zhang and T. Mu, Green Chem. Eng., 2023, 4, 303–311 Search PubMed.
- Y. Chen, C. Liu, Y. Wang, Y. Tian, Y. Li, M. Feng, Y. Guo, J. Han and T. Mu, Energy Fuels, 2023, 37, 5361–5369 Search PubMed.
|
This journal is © The Royal Society of Chemistry 2024 |