DOI:
10.1039/D4DT02766C
(Communication)
Dalton Trans., 2024, Advance Article
Synthesis and relaxivity of gadolinium-based DOTAGA conjugated 3-phosphoglycerate†
Received
30th September 2024
, Accepted 24th October 2024
First published on 25th October 2024
Abstract
The synthesis and characterization of a gadolinium-based contrast agent conjugated to 3-phosphoglycerate (Gd-3PG) are reported. The synthetic steps are optimized to incorporate a selective deprotection strategy for a primary tert-butyl dimethyl silyl (TBS) hydroxyl over a secondary one. The relaxivity of Gd-3PG shows characteristic improvement, likely due to secondary sphere effects and/or an increase in molecular weight (5.39 ± 0.14 mM−1 s−1 at 1.4 T and 37 °C). Michaelis–Menten enzymatic kinetics was measured on the modified 3PG-arm and it showed similar activity to the native 3PG metabolite, Km = 240 ± 30 μM. This agent and future versions of this type of GBCA, which are conjugated to glycolytic metabolites, were designed to monitor in vivo allosteric regulatory events in glycolytic processes.
Introduction
Gadolinium-based contrast agents (GBCAs) are routinely used in the clinic for magnetic resonance imaging (MRI) and for molecular imaging in important discovery experiments.1–4 Conjugation or binding of GBCAs to a protein enhances the relaxivity (r1) by slowing down the rotational correlation time (τr), commonly known as a τr boost.5 The GBCAs are likewise investigated using electron nuclear double resonance (ENDOR) as alternatives for measuring the amino acid distances of proteins in situ,6 where typical crystallization techniques have difficulties elucidating the dynamic conformations.
Biomolecular chemistry is challenging to visualize in vivo, especially with its unique allosteric regulatory effects. For example, highly energetic metabolites like 1,3-bisphosphoglycerate (1,3-BPG) have been shown to non-enzymatically and covalently tag exposed lysine residues on nearby proteins in a metabolic regulatory process.7 Our work focuses on the synthesis and characterization of such an agent by conjugating 3-phosphoglycerate (3PG) to a GBCA. Gd-3PG shows an enhanced r1, most likely attributed to secondary sphere effects or an increase in molecular weight from the 3PG-arm.8 The 3PG-arm had a similar Michaelis–Menten constant (Km) to that of reported 3PG in the phosphorylation to 1,3-BPG by phosphoglycerate kinase (PGK).9
Experimental
General methods
Unless otherwise noted, all synthetic manipulations were performed under a dry nitrogen atmosphere using standard Schlenk techniques. Solvents were still dried using Glass Contour columns or over-activated molecular sieves (4 angstroms). Reactions were monitored by TLC using precoated plastic plates (0.20 mm silica gel 60, UV254, Macherey-Nagel GmbH & Co., Duren Germany). Spots were visualized using potassium permanganate stain, Dragendorff stain, CAM stain, or UV active methods. 1H NMR and proton-decoupled 13C NMR spectra were recorded on a Bruker Avance III spectrometer (499.37 MHz for 1H, 125.58 MHz for 13C, and 150 MHz for 31P) and were processed using Bruker TOPSPIN 2.1 software. 1H, 13C, and 31P chemical shifts are reported as δ values in parts per million downfield from tetramethylsilane (TMS) or external triethyl phosphate. High-resolution mass spectrometry (HRMS) was performed using an Agilent 6210 time-of-flight (TOF) LC/MS instrument using electrospray ionization (ESI). Deuterated solvents were purchased from Cambridge Isotope Labs or Sigma-Aldrich. For high-performance liquid chromatography, an Agilent 1160/1260 DAD was used for traces, with ESI for ionization and 6120 Quadrupole LC/MS for detection. The analytical column was a Synergi 4 μm Polar-RP 80 Å, 4.6 × 150 mm column, and the semipreparative column was a Synergi 4 μm Polar-RP 80 Å 21.2 × 150 mm column.
Synthesis
Precursors methyl (R)-2,3-dihydroxypropanoate (2) and tert-butyl (S)-2,5-dihydroxypentanoate (8) were synthesized using previous literature procedures.10–12
Methyl (R)-2,3-bis((tert-butyldimethylsilyl)oxy)propanoate (3). 2 (0.7990 g, 6.65 mmol) was dissolved in DMF (14 mL) and then imidazole (1.1784 g, 17.3 mmol) and TBSCl (2.3012 g, 15.2 mmol) were added sequentially. The reaction was allowed to stir at rt overnight. The reaction was diluted with water (20 mL), extracted with EtAc (3 × 100 mL), and concentrated before purification by flash column chromatography (20% DCM in Hex, rf = 0.2) to afford a clear oil (2.3561 g, 92% yield). 1H-NMR (500 MHz, CDCl3, δ): 4.26 (dd, 1H), 3.76 (dd, 2H), 3.70 (s, 3H), 0.88 (s, 9H), 0.86 (s, 9H), 0.07 (s, 3H), 0.06 (s, 3H), 0.03 (m, 6H). 13C-NMR (125 MHz, CDCl3, δ): 172.48, 74.01, 65.96, 51.76, 25.83, 25.72, 18.36, 18.31, −5.06, −5.08, −5.38, −5.46.
Methyl (R)-2-((tert-butyldimethylsilyl)oxy)-3-hydroxypropanoate (4). 3 (7.0814 g, 20.3 mmol) was dissolved in a mixture of acetone (35.4 mL), acetic acid (77.9 mL), and water (42.5 mL) and stirred for 24 h at rt. The solution was then slowly quenched on ice (0 °C) with solid NaHCO3, extracted with DCM (4 × 200 mL), dried over Na2SO4, and concentrated. The remaining oil was purified by flash column chromatography (10% EtAc in Hex, rf = 0.15) to afford a clear oil (3.2473 g, 68% yield). 1H-NMR (500 MHz, CDCl3, δ): 4.29 (dd, 1H), 3.78 (m, 2H), 3.73 (s, 3H), 2.14 (t, 1H), 0.90 (s, 9H), 0.12 (s, 3H), 0.07 (s, 3H). 13C-NMR (125 MHz, CDCl3, δ): 172.25, 72.77, 65.04, 52.09, 25.70, 18.33, −4.89, −5.39. HRMS ESI-MS: 257.118 m/z [M + H]+ calculated for C10H22O4Si, 257.126 found.
Methyl (2R)-3-((tert-butoxy(diisopropylamino)phosphaneyl)oxy)-2-((tert-butyldi methylsilyl)oxy)propanoate (5). Under argon, 4 (0.5456 g, 2.33 mmol) was dissolved in ACN (4.5 mL). Diisopropylammonium tetrazolide salt (0.7030 g, 4.09 mmol) and tert-butyl tetraisopropylphosphorodiamidite (0.9011 g, 2.96 mmol) were added rapidly and sequentially. The solution was stirred at rt for 3 h and concentrated. The residue was resuspended in ether, vacuum filtered, and concentrated. The clear oil was purified on a plug of deactivated silica (10% EtAc in Hex with 1% TEA, rf = 0.85). The silica was deactivated by passing the mobile phase through several plug volumes. A clear oil was collected (1.0189 g, 95% yield). 1H-NMR (500 MHz, CDCl3, δ): 4.33 (dd, 1H), 3.78 (m, 0.5H), 3.69 (m, 3/1H), 3.56 (m, 0.5H), 1.31 (d, 9H), 1.13 (m, 12H), 0.88 (d, 9H), 0.07 (s, 3H), 0.06 (d, 3H). 13C-NMR (125 MHz, CDCl3, δ): 172.42, 74.86, 74.81, 73.02, 72.96, 65.03, 64.92, 51.77, 43.02, 42.92, 30.86, 30.79, 25.71, 24.52, 24.20, 18.31, −5.06, −5.11. 31P-NMR (150 MHz, CDCl3, δ): 138.77, 138.26. HRMS ESI-MS: 438.280 m/z [M + H]+ calculated for C20H44NO5PSi, 438.341 found.
tert-Butyl (2S)-5-((tert-butoxy((R)-2-((tert-butyldimethylsilyl)oxy)-3-methoxy-3-oxopropoxy)phosphoryl)oxy)-2-hydroxypentanoate (9). Under argon, 5 (0.9333 g, 2.14 mmol) was dissolved in ACN (4 ml), and the temperature was adjusted to −41 °C. A solution of 8 (0.4060 g, 2.14 mmol) in ACN (3 mL) and 1H-tetrazole (6.75 mL of a 0.4 M solution in ACN, 2.70 mmol) were added. The solution was allowed to slowly warm while in an ice bath. TLC monitored at 5 h showed no starting material, and di-tert-butylperoxide (DTBP) was added (1.2 mL of a 5–6 M solution in decane). The solution was stirred at rt for 1.5 h and concentrated to remove some of the ACN. The remaining solution was dissolved in 200 mL of EtAc and washed with 150 mL of sat. NaHSO3, 100 mL of sat. NaHCO3, 100 mL of brine, dried over NaSO4, and concentrated. The remaining oil was purified by flash column chromatography (50% EtAc in Hex, rf = 0.2) to produce a clear oil (1.1573 g, 65% yield). 1H-NMR (500 MHz, CDCl3, δ): 4.40 (m, 1H), 4.21 (m, 1H), 4.05 (m, 4H), 3.71 (s, 3H), 2.83 (s, 1H), 1.83–1.64 (m, 4H), 1.47 (m, 18H), 0.89 (s, 9H), 0.09 (s, 3H), 0.07 (d, 3H). 13C-NMR (125 MHz, CDCl3, δ): 174.30, 174.28, 83.24, 82.63, 71.78, 70.04, 68.45, 67.05, 52.14, 30.63, 29.81, 28.03, 25.76, 25.64, 18.30, −0.00, −5.05, −5.22. 31P-NMR (150 MHz, CDCl3, δ): −5.11, −5.18. HRMS ESI-MS: 543.275 m/z [M + H]+ calculated for C23H47O10PSi, 543.279 found.
tert-Butyl (2S)-5-((tert-butoxy((R)-2-((tert-butyldimethylsilyl)oxy)-3-methoxy-3-oxopropoxy)phosphoryl)oxy)-2-(((4-nitrophenyl)sulfonyl)oxy)pentanoate (10). 9 (0.4900 g, 0.904 mmol) was dissolved in DCM (11.5 mL) on ice (0 °C). TEA (0.24 mL, 1.72 mmol) and NsCl (0.5860 g, 2.65 mmol) were added sequentially. The reaction mixture was stirred on ice for 1 h, allowed to warm to rt, and stirred for an additional 3 h. The reaction mixture was added to a separation funnel and washed with 50 mL of sat. NaHCO3, dried over Na2SO4, and concentrated. The crude yellow oil was purified by flash column chromatography (25 to 50% EtAc in Hex, rf = 0.5 in 50% EtAc in Hex) to produce a yellowish oil (0.6572 g, 75% yield). 1H-NMR (500 MHz, CDCl3, δ): 8.37 (d, 2H), 8.14 (d, 2H), 4.88 (m, 1H), 4.38 (m 1H), 4.19 (m, 1H), 4.07 (m, 1H), 3.97 (m, 1H), 3.72 (d, 3H), 1.93 (m, 2H), 1.73 (m, 2H), 1.46 (d, 9H), 1.38 (s, 9H), 0.88 (s, 9H), 0.09 (s, 3H), 0.07 (s, 3H). 13C-NMR (125 MHz, CDCl3, δ): 171.01, 166.87, 150.78, 142.32, 129.44, 124.29, 83.60, 78.63, 71.72, 68.55, 66.04, 52.17, 29.80, 28.55, 27.81, 25.63, 18.30, −5.04, −5.24. HRMS ESI-MS: 1455.499 m/z [2M + H]+ calculated for C29H50NO14PSSi, 1455.511 found.
tBuDOTAGA-3PG. 10 (0.1749 g, 0.241 mmol) was dissolved in ACN (4 mL) and then tBuDO3A13 (0.1415 g, 0.238 mmol) and K2CO3 (0.1882 g, 1.36 mmol) were added. The reaction was stirred at rt overnight. The solution was vacuum filtered, rinsed with ACN, and the filtrate concentrated. The remaining residue was suspended in ether and gravity filtered into a separation funnel. 50 mL of water was added, and the water was washed with ether (3 × 40 mL). The organic phase was dried over Na2SO4 and concentrated. The crude oil was purified by flash column chromatography (7.5% MeOH in DCM, rf = 0.4) to afford a brownish oil (0.0729 g, 26% yield; product showed a ½ salt with a nosylate). 1H-NMR (500 MHz, CDCl3, δ): 8.13 (d, 2H), 4.39 (m, 1H), 4.20 (m, 1H), 4.07–3.97 (m, 4H), 3.72 (s, 3H), 3.36 (m, 3H), 3.15 (m, 3H), 2.79 (m, 2H), 2.49 (m, 3H), 2.30 (m, 3H), 2.11 (m, 4H), 1.80–1.60 (m, 4H), 1.43 (m, 45H), 0.88 (s, 9H), 0.09 (s, 3H), 0.07 (s, 3H). 13C-NMR (125 MHz, CDCl3, δ): 173.08, 172.97, 172.95, 171.01, 168.51, 153.82, 147.79, 127.69, 123.11, 82.59, 82.29, 81.89, 71.67, 68.53, 66.88, 61.08, 55.91, 55.61, 52.57, 52.20, 48.50, 48.11, 47.39, 47.18, 44.58, 31.08, 29.84, 27.90, 27.85, 27.75, 25.64, 18.31, −5.02, −5.20. HRMS ESI-MS: 1061.619 m/z [M + Na]+ calculated for C29H50NO14PSSi, 1061.646 found.
Gd-3PG. tBuDO3A-3PG (54.3 mg, 52.3 μmol) was dissolved in 2 mL of THF and 2 mL of 3 M LiOH for 1 h. On ice (0 °C), 10 mL of TFA with 100 μL of TIPS was slowly added and stirred for 24 h. The solution was concentrated and redissolved in water, and the pH was adjusted to 6 using 1 M NaOH. GdCl3·6H2O (25 mg, 67.4 μmol) was then added and the pH was adjusted to 6 over 24 h. The solution was then filtered and purified by reverse-phase HPLC on a Synergi 4 μm Polar-RP 80 Å using an isocratic flow of 0.1% FA in water (rf = 4.957 min). The product was lyophilized down to afford a white powder (3 mg, 7% yield). HRMS ESI-MS: 783.100 m/z [M − H]− calculated for C22H35GdN4O15P, 783.100 found.
(2S)-5-((((R)-2-Carboxy-2-hydroxyethoxy)(hydroxy)phosphoryl)oxy)-2-hydroxy pentanoic acid (3PG-Arm). Intermediate 9 (48.9 mg, 0.090 mmol) was dissolved in 3 M LiOH (250 μL) and THF (250 μL) for 15 min, and then TFA with 5% TIPS (5 mL) was slowly added on ice (0 °C) for 1 h. The solution was concentrated, and the crude product was purified by reverse phase HPLC on an Atlantis C18 in 0.1% TFA with an isocratic flow to afford a clear liquid (15.0 mg, 55% yield). 1H-NMR (500 MHz, D2O, δ): 4.49 (m, 1H), 4.32 (m, 1H), 4.15 (m, 2H), 3.92 (m, 2H), 1.90 (m, 1H), 1.75 (m, 3H). 13C-NMR (125 MHz, D2O, δ): 177.71, 174.64, 69.91, 69.97, 67.25, 66.00, 29.68, 25.36. HRMS ESI-MS: 303.047 m/z [M + H]+ calculated for C8H15O10P, 303.050 found.
Results and discussion
Synthesis
The synthesis was designed similarly to previous albumin binding agents like MS-325, which have an arm composed of a phosphodiester with aromatic moieties.14,15 Our work focuses on installing an arm with a common glycolytic metabolite, 3-phosphoglycerate (3PG) (Scheme 1). Commercial sources of protected R enantiomeric pure glyceric acid 1 are derived from the carbohydrate oxidation of mannitol.12 A quantitative yield of compound 2 was obtained after deprotection of the acetal with Dowex 50WX8.12 The hydroxyl groups were protected with TBS-Cl and imidazole in DMF to yield 3.16 A selective deprotection of the primary over the secondary TBS protected hydroxyl was carried out using acetic acid in a single liquid phase with water and acetone as cosolvents.17 Care was taken during the workup as the acetic acid was slowly quenched with solid NaHCO3 on ice to prevent further deprotection. A liquid extraction with dichloromethane and water allowed for easy separation of acetate and product 4, which was further purified by column chromatography.
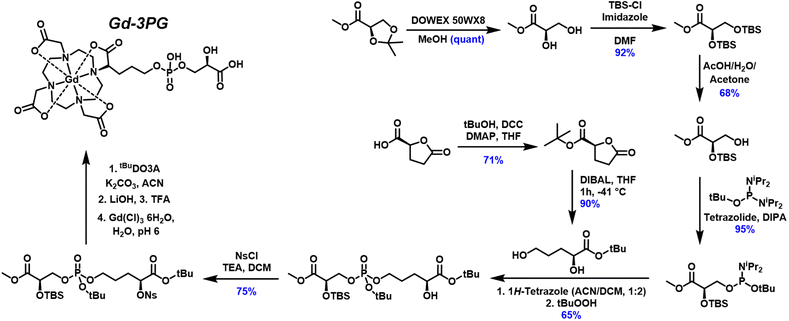 |
| Scheme 1 Synthetic scheme for the preparation of Gd-3PG. | |
The phosphodiester formation of the 3PG-arm is similar to the work done for synthetic DNA utilizing phosphorodiamidite substitution.18 The tert-butyl protected phosphorodiamidite was activated with tetrazolide and substituted with the primary hydroxyl of 4 to produce a high yield of 5. This intermediate was used relatively quickly because of its sensitivity to oxidation or hydrolysis. 5 was substantially non-polar compared to byproducts and was easily purified on a deactivated silica plug. The primary hydroxyl of intermediate 810,11 showed selective substitution over the secondary hydroxyl to yield 9. The secondary hydroxyl was then activated with nosyl chloride, 10, and used for an Sn2 reaction with tBuDO3A.13 Base deprotection with LiOH/THF was used to remove the methyl ester, and the reaction was adjusted to acidic conditions with TFA and TIPS as scavengers. It is essential to perform the base deprotection before adjusting to acidic conditions as the phosphodiester has a higher rate of hydrolysis under basic conditions, especially intramolecularly.19 The metalation was performed with GdCl3 and the resulting product was purified by HPLC to yield Gd-3PG. The polar endcap, phenyl ester (C18), required an isocratic method in acid buffer to retain Gd-3PG. Higher substitutions of Gd-3PG would require a form of anion exchange or ion-pairing to purify similar to oligonucleotides and mononucleotides.20
Relaxivity measurements
r1 measurements were taken under different magnetic fields and at different temperatures (1.4 T at 37 °C and 7 T at 21 °C). Typically, the r1 decreases with an increase in magnetic field strength, but since the temperature is lower at 7 T, the r1 of Gd-3PG appears the same (Table 1). The r1 of Gd-3PG is higher than that of common gadoterate and more similar to the trend of gadobutrol. The increase in Gd-3PG could either be due to the increase in molecular weight or attributed to the secondary sphere effects of the hydrogen bonding moieties, specifically the phosphodiester, hydroxyl, and carboxylic acid groups.
Table 1 The relaxivity of Gd-3PG in 1× PBS, gadoterate, and gadobutrol at 1.4 T and 7 T
GBCA |
1.4 T at 37 °C (mM−1 s−1) |
7 T (mM−1 s−1) |
Agents are in human plasma at 37 °C.21 At 21 °C. |
Gd-3PG |
5.39 ± 0.14 |
5.61 ± 0.08b |
Gadoteratea |
3.32 ± 0.13 |
2.84 ± 0.08 |
Gadobutrola |
4.78 ± 0.12 |
3.83 ± 0.24 |
Gd-3PG was then extrapolated with data from previously published phosphodiester incorporated GBCAs (Fig. 1b).14 While Gd-3PG is above the trendline, indicating a higher secondary sphere effect, there is significant uncertainty in the relationship between molecular weight and r1.
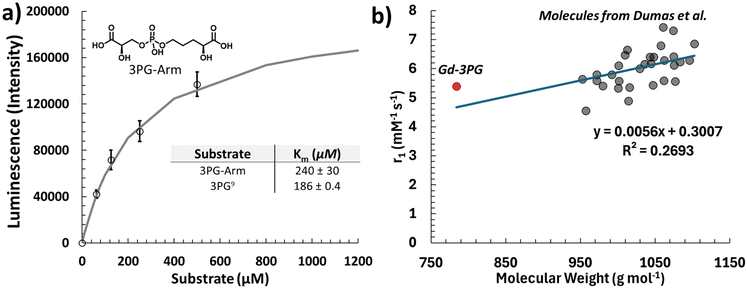 |
| Fig. 1 (a) Michaelis–Menten plot of the 3PG-arm being phosphorylated with PGK using an ADP-Glo Kinase assay. The kinetics of 3PG to 1,3-BPG is from the literature using an assay coupled to excess GAPDH tracking the loss of NADH.9 (b) Extrapolated plots showing the relationship between molecular weight and the r1 of similar phosphodiester GBCAs.14 With a relaxivity of 5.4 mM−1 s−1, Gd-3PG is above the trendline indicating a higher secondary sphere effect. However, based on the literature values (R2 = 0.2693), there is some uncertainty in the relationship between molecular weight and r1. | |
Enzymatic assays
Enzymatic kinetics was determined using the 3PG-Arm, which showed phosphorylation by PGK. A Michaelis–Menten constant of Km = 240 ± 30 μM was obtained, similar to that of the known 3PG metabolite9 (Fig. 1a). This demonstrates that even modified 3PG metabolites can be phosphorylated enzymatically. When compared to controls without PGK, Gd-3PG showed no change in r1 under active buffer conditions with PGK (Fig. S3†). This could be due to the low influence of phosphorylation on r1 or the decreased activity of PGK caused by the macrocyclic chelator. During these experiments, it was determined that ATP concentrations increase the r1 for both gadoterate and Gd-3PG with similar slopes (Fig. S2†). The effect of ATP on r1 is therefore independent of the 3PG-arm.
Conclusion
The synthesis of a GBCA conjugated to a glycolytic metabolite (3PG) is reported to afford a relatively high yield of the synthetic 3PG-arm (10, 29% linear yield). The r1 of Gd-3PG was higher than that of common gadoterate due most likely to secondary sphere effects or molecular weight enhancement from the 3PG-arm (5.39 ± 0.14 mM−1 s−1 at 1.4 T and 37 °C). The 3PG-arm demonstrated enzymatic activity, showing the possibility of modifying metabolites for future MRI probes. These results demonstrate the potential to use glycolytic metabolites conjugated to GBCAs for investigating metabolic function.
Data availability
The authors confirm that the data supporting the findings of this study are available within the article and its ESI.† Raw data that support the findings of this study are available from the corresponding author, upon reasonable request.
Conflicts of interest
The authors declare no competing financial interests.
Acknowledgements
A. R. B. gratefully acknowledges the NSF Graduate Research Fellowship Grant no. DGE-2234667. This work made use of the IMSERC MS facility at Northwestern University, which has received support from the Soft and Hybrid Nanotechnology Experimental (SHyNE) Resource (NSF ECCS-2025633), the State of Illinois, and the International Institute for Nanotechnology (IIN). This work employed the facilities of the High Throughput Analysis Laboratory (HTAL, NU).
References
- H. Li and T. J. Meade, Molecular Magnetic Resonance Imaging with Gd(III)-Based Contrast Agents: Challenges and Key Advances, J. Am. Chem. Soc., 2019, 141(43), 17025–17041 CrossRef CAS PubMed.
- J. Wahsner, E. M. Gale, A. Rodriguez-Rodriguez and P. Caravan, Chemistry of MRI Contrast Agents: Current Challenges and New Frontiers, Chem. Rev., 2019, 119(2), 957–1057 CrossRef CAS PubMed.
- J. H. Tang, H. Li, C. N. Yuan, G. Parigi, C. Luchinat and T. J. Meade, Molecular Engineering of Self-Immolative Bioresponsive MR Probes, J. Am. Chem. Soc., 2023, 145(18), 10045–10050 CrossRef CAS PubMed.
- L. M. Lilley, S. Kamper, M. Caldwell, Z. K. Chia, D. Ballweg, L. Vistain, J. Krimmel, T. A. Mills, K. MacRenaris, P. Lee, E. A. Waters and T. J. Meade, Self-Immolative Activation of beta-Galactosidase-Responsive Probes for In Vivo MR Imaging in Mouse Models, Angew. Chem., Int. Ed., 2020, 59(1), 388–394 CrossRef CAS PubMed.
- A. Merbach, L. Helm and E. Toth, The Chemistry of Contrast Agents in Medical Magnetic Resonance Imaging, Wiley, 2nd edn, 2013 Search PubMed.
- M. Judd, E. H. Abdelkader, M. Qi, J. R. Harmer, T. Huber, A. Godt, A. Savitsky, G. Otting and N. Cox, Short-range ENDOR distance measurements between Gd(iii) and trifluoromethyl labels in proteins, Phys. Chem. Chem. Phys., 2022, 24(41), 25214–25226 RSC.
- R. E. Moellering and B. F. Cravatt, Functional Lysine Modification by an Intrinsically Reactive Primary Glycolytic Metabolite, Science, 2013, 341(6145), 549–553 CrossRef CAS PubMed.
- M. Botta, Second Coordination Sphere Water Molecules and Relaxivity of Gadolinium(III) Complexes: Implications for MRI Contrast Agents, Eur. J. Inorg. Chem., 2000, 3, 399–407 CrossRef.
- C. M. Jin, X. B. Zhu, H. Wu, Y. Q. Wang and X. Hu, Perturbation of Phosphoglycerate Kinase 1 Only Marginally Affects Glycolysis in Cancer Cells, J. Biol. Chem., 2020, 295(19), 6425–6446 CrossRef CAS PubMed.
- J. Garcia-Calvo, T. Torroba, V. Branas-Fresnillo, G. Perdomo, I. Cozar-Castellano, Y. H. Li, Y. M. Legrand and M. Barboiu, Manipulation of Transmembrane Transport by Synthetic K+ Ionophore Depsipeptides and Its Implications in Glucose-Stimulated Insulin Secretion in β-Cells, Chem. – Eur. J., 2019, 25(39), 9287–9294 CrossRef CAS PubMed.
- U. Schmidt, C. Braun and H. Sutoris, Enantioselective syntheses of (R)- and (S)-hexahydropyridazine-3-carboxylic acid derivatives, Synthesis, 1996, 2, 223–229 CrossRef.
- N. R. Lees, L. C. Han, M. J. Byrne, J. A. Davies, A. E. Parnell, P. E. J. Moreland, J. E. M. Stach, M. W. van der Kamp, C. L. Willis and P. R. Race, An Esterase-like Lyase Catalyzes Acetate Elimination in Spirotetronate/Spirotetramate Biosynthesis, Angew. Chem., Int. Ed., 2019, 58(8), 2305–2309 CrossRef CAS PubMed.
- D. J. Mastarone, V. S. R. Harrison, A. L. Eckermann, G. Parigi, C. Luchinat and T. J. Meade, A Modular System for the Synthesis of Multiplexed Magnetic Resonance Probes, J. Am. Chem. Soc., 2011, 133(14), 5329–5337 CrossRef CAS PubMed.
- S. Dumas, V. Jacques, W. C. Sun, J. S. Troughton, J. T. Welch, J. M. Chasse, H. Schmitt-Willich and P. Caravan, High Relaxivity Magnetic Resonance Imaging Contrast Agents Part 1 Impact of Single Donor Atom Substitution on Relaxivity of Serum Albumin-Bound Gadolinium Complexes, Invest. Radiol., 2010, 45(10), 600–612 CrossRef CAS PubMed.
- P. Caravan, N. J. Cloutier, M. T. Greenfield, S. A. McDermid, S. U. Dunham, J. W. M. Bulte, J. C. Amedio, R. J. Looby, R. M. Supkowski, W. D. Horrocks, T. J. McMurry and R. B. Lauffer, The Interaction of MS-325 with Human Serum Albumin and Its Effect on Proton Relaxation Rates, J. Am. Chem. Soc., 2002, 124(12), 3152–3162 CrossRef CAS PubMed.
- M. Ikubo, A. Inoue, S. Nakamura, S. J. Jung, M. Sayama, Y. Otani, A. Uwamizu, K. Suzuki, T. Kishi, A. Shuto, J. Ishiguro, M. Okudaira, K. Kano, K. Makide, J. Aoki and T. Ohwada, Structure-Activity Relationships of Lysophosphatidylserine Analogs as Agonists of G-Protein-Coupled Receptors GPR34, P2Y10, and GPR174, J. Med. Chem., 2015, 58(10), 4204–4219 CrossRef CAS PubMed.
- A. Kawai, O. Hara, Y. Hamada and T. Shioiri, Stereoselective Synthesis of the Hydroxy Amino-Acid Moiety of AI-77-B, A Gastroprotective Substance from Bacillus-Pumilus AI-77, Tetrahedron Lett., 1988, 29(48), 6331–6334 CrossRef CAS.
- N. D. Sinha, J. Biernat, J. McManus and H. Koster, Polymer Support Iligonucleotides Synthesis. 18. Use of Beta-cyanoethyl-N,N-dialkyamino-/N-Morpholino Phosphoramidite of Deoxynucleosides for the Synthesis of DNA Fragments Simplifying Deprotection and Isolation of the Final Product, Nucleic Acids Res., 1984, 12(11), 4539–4557 CrossRef CAS PubMed.
- J. R. Cox and O. B. Ramsay, Mechanisms of Nucleophilic Substitution in Phosphate Esters, Chem. Rev., 1964, 64(4), 317–351 CrossRef CAS.
- D. R. W. Hodgson, Physicochemical Aspects of Aqueous and Nonaqueous Approaches to the Preparation of Nucleosides, Nucleotides and Phosphate Ester Mimics, in Advances in Physical Organic Chemistry, ed. I. H. Williams and N. H. Williams, 2017, vol. 51, pp. 187–219 Search PubMed.
- P. Szomolanyi, M. Rohrer, T. Frenzel, I. M. Noebauer-Hohmann, G. Jost, J. Endrikat, S. Trattnig and H. Pietsch, Comparison of the Relaxivities of Macrocyclic Gadolinium-Based Contrast Agents in Human Plasma at 1.5, 3, and 7 T, and Blood at 3 T, Invest. Radiol., 2019, 54(9), 559–564 CrossRef CAS PubMed.
|
This journal is © The Royal Society of Chemistry 2024 |
Click here to see how this site uses Cookies. View our privacy policy here.