DOI:
10.1039/D4DT02022G
(Paper)
Dalton Trans., 2024,
53, 13831-13836
Novel ligands from direct benzylic functionalisation of tris(2-pyridylmethyl)amine†
Received
13th July 2024
, Accepted 1st August 2024
First published on 2nd August 2024
Abstract
Tris-(2-pyridylmethyl)amines (TPA or TPMA) are polipyrydine-based ligands extensively used in catalysis and supramolecular chemistry due their capability to form stable tetradentate complexes with a large variety of metals. The unsubstituted ligand, which is also commercially available, can be synthesised by consecutive alkylation of a picoline or by reductive amination of a pyridine aldehyde. In this article, we report a novel synthetic method which opens to the post-functionalisation of these ligands in the benzylic position. This novel derivatization strategy, beside providing synthetic access to novel structures and functions, has been used to prepare a series of metal complexes which have been tested in photochemical hydrogen evolution.
Introduction
Among the large variety of polydentate ligands present in literature, tris(2-pyridylmethyl)amines (TPA or TPMA) are emerging as one of the most versatile molecular systems due to their ability to bind effectively a wide array of transition metals with a precise tripodal geometry.1 This capability has been largely and efficiently exploited in catalysis and in supramolecular chemistry. For instance, Cu(II) complexes are the reference systems for Atom Transfer Radical Polymerisation,2–5 Co(II) complexes have been used for promoting the Hydrogen Evolution Reaction (HER),6–9 and Zn(II) complexes have been extensively used in chiral sensing.10–14
Unsubstituted tris(2-pyridylmethyl)amine (1) is commercially available ligand, which can be synthesized by performing an alkylation of primary (2-pyridyl)methylamine with two equivalents of 2-(chloromethyl)pyridine in aqueous NaOH solution, this strategy is inspired by the work of Anderegg and Wenk.15 We recently introduced the use of reductive amination of an aldehyde precursors with sodium triacetoxyborohydride for the synthesis of 1 and its analogues.10 This strategy allows mild reaction conditions and high yields. The introduction of additional functional groups is usually performed before the formation of the scaffold or by including bromide functions in the pyridines followed by cross-coupling reactions.16
While a large variety of “decorations” on the pyridine rings have been performed, either to change the electronic characteristic of the amines,2 or to develop more complex architectures,17–19 the functionalisation of the methylene bridges has been less studied.20 This position has been used to control the helical wrapping of the ligand around the metal,21 and is usually accessed by the alkylation of an α-substituted secondary amine.
In this paper we report an original synthetic strategy for the functionalisation of the benzylic position of TPMA using a strong base on the full ligand followed by the reaction with an electrophile. This has allowed the preparation of six novel ligands characterised by different arms at the benzylic position, and has also led to discovery of a reaction for the synthesis of a TPMA “dimer”. Co(II) complexes of selected ligands have been used in photocatalytic hydrogen production to test the unaltered performances of the novel systems.
Results and discussion
Synthesis of the functionalised ligands
We discovered the novel functionalization reaction starting from an attempt of a lithiation/alkylation of the bromo-functionalised TPMA ligand 1-Br (Fig. 1), which displayed unusual reactivity. Rather than the expected aromatic substitution product, TPMA derivative 2a methylated at the benzylic position was observed as the only identifiable product. The formation of 2a was evident by 1H-NMR analysis. In particular, the methylene groups signal turned from a broad singlet in the starting ligand 1-Br to a distinct AB system with a large geminal coupling constant in 2a. A similar change in the NMR spectrum of TPMA derivatives after introducing a stereocenter on the ligand backbone was also reported by Canary.22
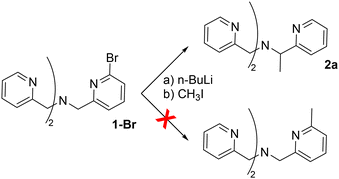 |
| Fig. 1 Addition of butyl lithium to 1-Br followed by iodomethane addition lead to benzyl functionalisation. | |
Interestingly, when we applied the same reaction conditions on the parent ligand 1, we obtained 2a in high yields (94%). This suggests for both 1 and 1-Br the formation of a carbanionic intermediate at the benzylic position, that allows the subsequent functionalization by reaction with an electrophile. As a first indication of a deprotonation of a methylene groups, we probed the H/D exchange by performing the reaction of 1 with butyllithium and quenching with deuterium oxide. The 1H NMR analysis of the reaction crude confirmed a complete substitution of one methylene hydrogen of the starting ligand with a deuterium atom (see ESI†).
To the best of our knowledge, a similar post-synthetic transformation on a TPMA species has no precedent in the literature. Therefore, we decided to study the extent of this new functionalization strategy, which can give access to new ligands and expand further the rich coordination chemistry of TPMA derivatives. We performed a screening of different electrophiles (alkyl halides, epoxides, aldehydes, esters and ketones) that are generally suitable for reactions with a carbanion by nucleophilic substitution or nucleophilic addition.
All the reactions were carried out in similar experimental conditions, under inert atmosphere, using n-butyllithium as a base at −78 °C and allowing the reaction mixture to slowly warm up to room temperature after the electrophile addition.
In this screening, we were able to select the most promising electrophile classes for a successful functionalization (Fig. 2). Epoxides and esters were found to be ineffective, as only the starting material was recovered even after prolonged reaction times in the presence of styrene oxide and ethyl acetate. Ketones, such as acetone and cyclopentanone, gave only low conversions, with traces of product detected by NMR analysis on the reaction crude. On the other hand, the reaction protocol was particularly suitable for TPMA functionalization with primary alkyl iodides and aldehydes. Secondary alkyl iodides with limited steric hindrance (such as 2-iodopropane) also gave access to the desired product 2b.
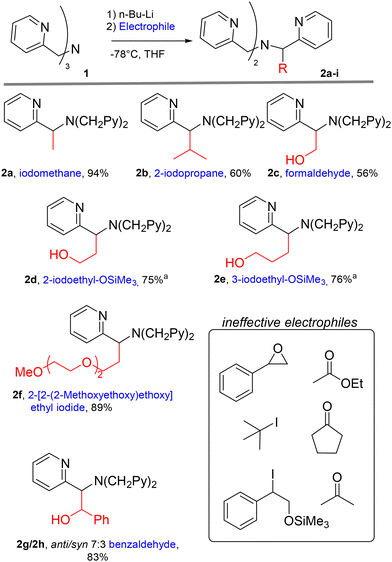 |
| Fig. 2 TPMA derivatives obtained through the deprotonation of 1 followed by the addiction of an electrophile. a Products obtained after the trimethylsilyl group cleavage. | |
Interestingly, by using aldehydes or trimethylsilyl-protected iodoalcohols as electrophiles, it is possible to insert an additional coordination site on the TPMA backbone with an oxygen donor.
The length of this fourth coordination arm can be tuned by the electrophile choice. The functionalization was successful using the trimethylsilyl (TMS) protecting group for the hydroxyl group and led to the synthesis of derivatives 2d and 2e. Derivative 2c, equipped with a hydroxymethyl moiety, was obtained by bubbling gaseous formaldehyde generated by thermal decomposition of p-formaldehyde in a solution containing deprotonated 1.
The functionalization of TPMA species with hydrophilic PEG units can be interesting for the synthesis of complexes suitable for catalysis in water. This was achieved by synthesising derivative 2f carrying a 3-unit PEG chain.
The use of substituted aldehydes as electrophiles is feasible, however the generation of two stereocenters on the final product leads to purification issues. For instance, by the reaction with benzaldehyde we obtained a diastereoisomeric anti/syn mixture in 70
:
30 ratio. After chromatographic separation, the major diastereoisomer 2g was unequivocally identified as the anti one through X-ray diffraction analysis of suitable single crystals (Fig. 3).
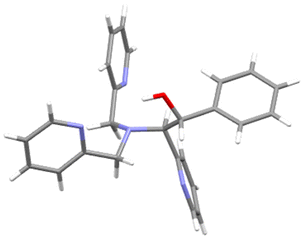 |
| Fig. 3 X-ray structure TPMA derivative 2f arising from benzaldeyde addition to the benzylic position. | |
Synthesis of the metal complexes and catalysis
The general procedure for the synthesis of metal complexes consisted in the mixing of an equimolar amount of TPMA derivative with a cobalt(II) or zinc(II) perchlorate salt in acetonitrile. The so-obtained solution was stirred at room temperature for 1 hour, and the desired compounds were obtained by recrystallization with diethyl ether (Fig. 4).
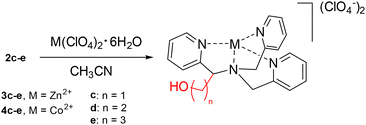 |
| Fig. 4 Synthesis of the cobalt and zinc complexes of the functionalised TPMA ligands. | |
We focused on the synthesis of complexes of ligands 2c–e, which bear an alkyll hydroxy group of different lengths. Zinc complexes 3c–e were studied by NMR spectroscopy which evidenced in all cases a loss of symmetry of the ligands upon complexation (2 sets of signals for the originally equivalent pyridine rings) and showed the presence of a distinct OH proton signal.
This additional hydroxy functionality can be a useful proton relay in close proximity to the metal centre which could enhance the activity of HER catalysts.44 For this reason, we synthesized also the corresponding Co(II) complexes of ligands 2c–e. All the cobalt complexes were stable in their original oxidation state and the obtained products were characterized by ESI-MS and elemental analysis (see ESI†).
The cobalt complexes 4c–e were tested as catalysts for the HER under light-driven conditions in 1 M acetate buffer solution at pH 5 in combination with 0.5 mM [Ru(bpy)3]2+ (bpy = 2,2′-bipyridine) as the sensitizer and 0.1 M ascorbate as the sacrificial electron acceptor. These experimental conditions were chosen for comparison with previous experiments performed using related cobalt(II) TPMA complexes.23 The kinetics of hydrogen production at a concentration of 0.1 mM cobalt complex are reported in Fig. 5a. In Fig. 5b are represented for each sample the maximum turnover number (TON), defined as the ratio between the moles of H2 at the plateau and the moles of catalyst, and the quantum efficiency (QE), calculated as two-times the ratio between the initial rate of H2 production and the photon flux.24,25
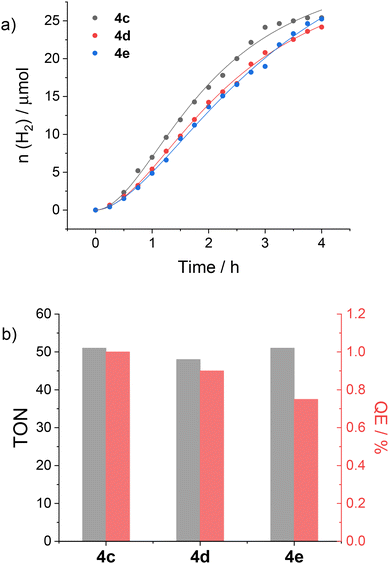 |
| Fig. 5 (a) Kinetics of hydrogen evolution upon irradiation (460 nm LED) of 1 M acetate buffer (pH 5) solutions containing 0.5 mM [Ru(bpy)3]Cl2·6H2O, 0.1 M ascorbic acid and 0.1 mM 4c–e; (b) maximum TONs and QEs. | |
Inspection of the kinetic data shows that, for all complexes, hydrogen generation occurs upon photoirradiation and levels off after approximately 4 h. Similar amounts of H2 are produced at plateau, leading to comparable maximum TONs (in the range 48–51). Similar rates and QE are also recorded, only slightly decreasing in the order 4c > 4d > 4e, i.e., with increasing the length and flexibility of the substituent. These results clearly point to a minor, if any, role of the hydroxyl group in assisting HER catalysis, most likely associated with the geometric constrains imparted by the molecular structure. Interestingly, the performances are perfectly in line with those reported for cobalt(II) TPMA analogues,6,24,26 thus highlighting that functionalization of the α position does not appreciably alter the catalytic ability of the metal complexes. This evidence is particularly relevant in view of the potential use of this class of complexes within more complex supramolecular systems as well as for the functionalization of (photo)electrode surfaces.23,27,28
Mechanistic considerations
As reported in the literature, the deprotonation at the α position of ortho/para alkyl pyridine species gives an anionic species where the negative charge is shared between the α-carbon and the pyridine nitrogen.29–31 We can therefore assume the formation of a species with a carbanionic character also from the treatment of 1 with butyllithium. However, to further prove the formation of this intermediate, we tried to trap the supposed carbanionic species through oxidative coupling, a well-documented transformation of carbanions.32–36 This reaction leads to the formation of homodimers by the formal oxidation of the carbon bearing the negative charge.
We attempted the coupling using some common oxidants such as iodine and potassium permanganate, but we observed no conversion and decomposition respectively.
Interestingly, when we attempted the functionalization of 1 with p-nitrobenzaldehyde we obtained the expected product (2i) in low yields and a new TPMA derivative (2l) was observed as a side product (Fig. 6). 2l was found to be a homodimer in which two TPMA units are linked through a C–C bond between two α carbons. We obtained suitable crystals for X-ray analysis from the reaction crude which showed that 2i and 2l co-crystallized in the same unit cell. We then repeated our newly developed protocol using nitrobenzene as electrophile and 2i was obtained in a 51% yield. Apparently, the nitro group mild oxidant activity37,38 was sufficient for triggering the oxidative coupling of the TPMA carbanion. Interestingly, although the dimerization involved the formation of two new stereocenters, 2i was obtained exclusively as the anti diastereoisomer.
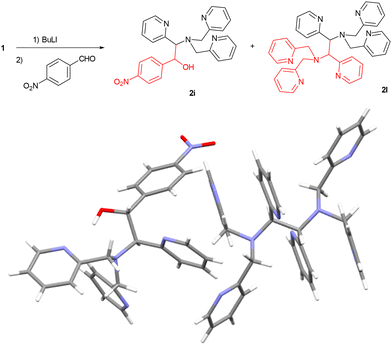 |
| Fig. 6 (up) Functionalization of 1 with p-nitrobenzaldehyde, (down) crystal structure of 2i and 2l. | |
Experimental
Materials and instrumentation
THF was distilled over Na/benzophenone and kept in inert atmosphere. TMS-protected iodoalcohols39,40 and 2-[2-(2-methoxyethoxy) ethoxy]ethyl iodide41 are known compounds obtained by reported procedures. All other chemicals were purchased from Merck, TCI or Fluorochem and used as received. NMR spectra were recorded on Bruker a Av-Neo 400 instrument or on a Bruker Avance III 600 HD instrument. All 1H NMR spectra were referenced to the residual protiated impurity of CDCl3 (7.26 ppm), or d6-DMSO (2.50 ppm). 13C NMR spectra were referenced to the CDCl3 peak (77.16 ppm) or d6-DMSO peak (39.52 ppm). GC-MS analysis was performed on an Agilent 6850 Network GC System coupled to an Agilent 5975 Series MSD. High resolution mass analyses were performed on a Q Exactive Hybrid Quadrupole-Orbitrap Mass Spectrometer. Elemental analyses were performed with a Thermo Scientific Flash 2000 (CHNS analyser) instrument.
General procedure for TMS-protected iodoalcohols
The required bromoalcohol (7.0 mmol) and HMDS (0.88 mL, 0.42 mmol) were mixed in a round bottom flask. After one hour the crude was filtered over Celite and washed with CH2Cl2. The solution was evaporated and the crude was directly used for the subsequent step. The silylation crude was dissolved in dry acetone (10 mL), a solution of NaI (1.70 g, 11 mmol) in dry acetone (10 mL) was added. The mixture was refluxed overnight with a CaCl2 valve. CH2Cl2 (50 mL) was added and the inorganic salts were filtered off over Celite. The solution was evaporated to dryness to give the product as a colorless oil in a 50–60% overall yield.
General procedure for TPMA functionalisation
Under argon atmosphere, TPMA (200 mg, 0.69 mmol) was dissolved in dry THF (7 mL) and cooled at −78 °C. BuLi 2.5 M in hexanes was added (330 μL, 0.83 mmol). After 30 minutes the required electrophile was added (0.83 mmol) and the obtained mixture was slowly brought to room temperature and stirred overnight. The reaction was quenched with a saturated NH4Cl aqueous solution, extracted with CH2Cl2 (15 mL × 3) and washed with water. The organic phase was dried over Na2SO4 and evaporated to dryness. When TMS group cleavage was required, the crude was dissolved in 5 mL of THF, then a 1.0 M solution of TBAF in THF (1.1 mL) was added. After stirring for 2 hours at room temperature, 50 mL of CH2Cl2 was added and washed with a saturated NH4Cl aqueous solution and water. The organic phase was dried over Na2SO4 and evaporated to dryness. Finally, functionalized TPMAs were purified by chromatography over basic alumina using CH2Cl2/MeOH 100
:
1 as eluent.
General procedure for cobalt and zinc complexes synthesis
The required ligand (0.15 mmol) and metal salt (Zn(ClO4)2·6H2O or Co(ClO4)2·6H2O) (0.15 mmol) were dissolved in acetonitrile (3 mL) and stirred for 1 hour at room temperature. Then, ethyl ether (10 mL) was carefully added to the reaction flask. After a few hours a dark precipitate was filtered and dried under vacuum affording the desired zinc or cobalt complex in a 70–80% yield.
Photochemical hydrogen evolution
Light-driven hydrogen evolution experiments were performed upon irradiation using a 50 mW 460 nm LED of a reactor containing the solution (a 1 cm diameter cylindrical cell with headspace obtained from a round-bottom flask). In a typical photochemical experiment, samples were prepared in 20 mL scintillation vials by mixing stock solutions of [Ru(bpy)3]Cl2·6H2O and the catalysts, followed by the addition of buffer (1 M acetic acid buffer). Ascorbic acid was added as a solid. The solution was then placed in the reactor, purged with argon for 20 minutes, and continuously stirred. Experiments were run in duplicate and the results are reported as the average of two independent experiments. H2 was detected and quantified using a GC. The photoreactor cell was sealed during the reaction, with the headspace comprising four ports closed with Swagelok® connections. Two ports were part of a closed loop involving GC gas inlet and sample vent to analyse the headspace content without significant gas consumption, while the other two were for the degassing procedure (input and output). The gas phase of the reaction vessel was analysed using an Agilent Technologies 490 microGC equipped with a 5 Å molecular sieve column (10 m), a thermal conductivity detector, and argon as the carrier gas. The portion of gas not injected into the column was reintroduced into the headspace, ensuring minimal gas consumption throughout the catalytic experiment. Calibration of the system to quantify the exact amount of H2 was performed using the external calibration method, conducting galvanostatic electrolysis (current of 1 mA) of a 1 M H2SO4 solution (assuming 100% faradaic efficiency). Quantum efficiency (QE) of H2 production was calculated via actinometry using the reaction between [Ru(bpy)3]Cl2·6H2O and 9,10-diphenylanthracene (DPA) in aerated acetonitrile.42,43
Conclusions
Here we reported a new post-synthetic functionalization of tris-(2-pyridylmethyl) amine ligands. This methodology was suitable for the insertion of useful functional groups on the TPMA groups by its reaction with alkyl iodides or aldehydes. We employed the Co(II) complexes of a series of modified ligands to investigate the efficiency of an additional hydroxy group on the catalyst activity in HER. Finally, we were able to trap the carbanion intermediate as its homodimer through a usual reactivity of the nitroarenes as oxidants.
Data availability
The data supporting this article have been included as part of the ESI.†
Crystallographic data for 2f and 2l has been deposited at the CCDC Depository under 2369954–6† and can be obtained from https://www.ccdc.cam.ac.uk.
Conflicts of interest
There are no conflicts to declare.
Acknowledgements
Financial support from the Italian MUR (PRIN 2020927WY3_001 ElectroLight4Value, to M. N.), from the European Union – Next Generation UE (PRIN2022 PNRR P2022ZSPWF PHOTOCORE to M. N., 2022CXHY3A_003 INVESTCPE to C. Z.), from the University of Ferrara (FAR2023, to M. N.), from the University of Modena and Reggio Emilia (to P. Z and A. Z.), and from the Fondazione CARIPARO (Chiralspace, to C. Z.) is gratefully acknowledged.
References
- C. Bravin, E. Badetti, G. Licini and C. Zonta, Coord. Chem. Rev., 2021, 427, 213558 CrossRef CAS.
- N. A. Carmo dos Santos, F. Lorandi, E. Badetti, K. Wurst, A. A. Isse, A. Gennaro, G. Licini and C. Zonta, Polymer, 2017, 128, 169–176 CrossRef CAS.
- K. Schröder, R. T. Mathers, J. Buback, D. Konkolewicz, A. J. D. Magenau and K. Matyjaszewski, ACS Macro Lett., 2012, 1, 1037–1040 CrossRef PubMed.
- A. Kaur, T. G. Ribelli, K. Schröder, K. Matyjaszewski and T. Pintauer, Inorg. Chem., 2015, 54, 1474–1486 CrossRef CAS PubMed.
- M. Fantin, A. A. Isse, N. Bortolamei, K. Matyjaszewski and A. Gennaro, Electrochim. Acta, 2016, 222, 393–401 CrossRef CAS.
- M. Natali, E. Badetti, E. Deponti, M. Gamberoni, F. A. Scaramuzzo, A. Sartorel and C. Zonta, Dalton Trans., 2016, 45, 14764–14773 RSC.
- J. Wang, C. Li, Q. Zhou, W. Wang, Y. Hou, B. Zhang and X. Wang, Catal. Sci. Technol., 2016, 6, 8482–8489 RSC.
- X. Guo, C. Li, W. Wang, Y. Hou, B. Zhang, X. Wang and Q. Zhou, Dalton Trans., 2021, 50, 2042–2049 RSC.
- P. Wang, G. Liang, M. R. Reddy, M. Long, K. Driskill, C. Lyons, B. Donnadieu, J. C. Bollinger, C. E. Webster and X. Zhao, J. Am. Chem. Soc., 2018, 140, 9219–9229 CrossRef CAS PubMed.
- F. A. Scaramuzzo, G. Licini and C. Zonta, Chem. – Eur. J., 2013, 19, 16809–16813 CrossRef CAS PubMed.
- F. Begato, R. Penasa, G. Licini and C. Zonta, ACS Sens., 2022, 7, 1390–1394 CrossRef CAS PubMed.
- C. Bravin, G. Mason, G. Licini and C. Zonta, J. Am. Chem. Soc., 2019, 141, 11963–11969 CrossRef CAS PubMed.
- R. Penasa, F. Begato, G. Licini, K. Wurst, S. Abbate, G. Longhi and C. Zonta, Chem. Commun., 2023, 59, 6714–6717 RSC.
- E. Badetti, K. Wurst, G. Licini and C. Zonta, Chem. – Eur. J., 2016, 22, 6515–6518 CrossRef CAS PubMed.
- G. Anderegg and F. Wenk, Helv. Chim. Acta, 1967, 50, 2330–2332 CrossRef CAS.
- C. Bravin, E. Badetti, F. A. Scaramuzzo, G. Licini and C. Zonta, J. Am. Chem. Soc., 2017, 139, 6456–6460 CrossRef CAS PubMed.
- C. Bravin, J. A. Piękoś, G. Licini, C. A. Hunter and C. Zonta, Angew. Chem., Int. Ed., 2021, 60, 23871–23877 CrossRef CAS PubMed.
- C. Bravin, A. Guidetti, G. Licini and C. Zonta, Chem. Sci., 2019, 10, 3523–3528 RSC.
- F. Begato, R. Penasa, K. Wurst, G. Licini and C. Zonta, Angew. Chem., Int. Ed., 2023, 62, e202304490 CrossRef CAS PubMed.
- H. Kotani, T. Sugiyama, T. Ishizuka, Y. Shiota, K. Yoshizawa and T. Kojima, J. Am. Chem. Soc., 2015, 137, 11222–11225 CrossRef CAS PubMed.
- F. Begato, R. Penasa, G. Licini and C. Zonta, Chem. Commun., 2021, 57, 10019–10022 RSC.
- J. W. Canary, C. S. Allen, J. M. Castagnetto and Y. Wang, J. Am. Chem. Soc., 1995, 117, 8484–8485 CrossRef CAS.
- E. Benazzi, F. Begato, A. Niorettini, L. Destro, K. Wurst, G. Licini, S. Agnoli, C. Zonta and M. Natali, J. Mater. Chem. A, 2021, 9, 20032–20039 RSC.
- F. Droghetti, F. Lucarini, A. Molinari, A. Ruggi and M. Natali, Dalton Trans., 2022, 51, 10658–10673 RSC.
- F. Droghetti, A. Amati, A. Ruggi and M. Natali, Chem. Commun., 2024, 60, 658–673 RSC.
- N. A. Carmo dos Santos, M. Natali, E. Badetti, K. Wurst, G. Licini and C. Zonta, Dalton Trans., 2017, 46, 16455–16464 RSC.
- E. S. Andreiadis, P.-A. Jacques, P. D. Tran, A. Leyris, M. Chavarot-Kerlidou, B. Jousselme, M. Matheron, J. Pécaut, S. Palacin, M. Fontecave and V. Artero, Nat. Chem., 2013, 5, 48–53 CrossRef CAS PubMed.
- N. M. Muresan, J. Willkomm, D. Mersch, Y. Vaynzof and E. Reisner, Angew. Chem., Int. Ed., 2012, 51, 12749–12753 CrossRef CAS PubMed.
- E. M. Kaiser, Tetrahedron, 1983, 39, 2055–2064 CrossRef CAS.
- M. Hasyeoui, P. M. Chapple, F. Lassagne, T. Roisnel, M. Cordier, A. Samarat, Y. Sarazin and F. Mongin, Eur. J. Org. Chem., 2023, e202300555, DOI:10.1002/ejoc.202300555.
- A. R. Kennedy, R. E. Mulvey, R. I. Urquhart and S. D. Robertson, Dalton Trans., 2014, 43, 14265–14274 RSC.
- A. Y. Nuriye and J. Barr, Results Chem., 2022, 4, 100407 CrossRef CAS.
- E. M. Kaiser, J. Am. Chem. Soc., 1967, 89, 3659–3660 CrossRef CAS.
- A. V. Zorin, A. R. Chanysheva, A. O. Lenkova and V. V. Zorin, Russ. J. Gen. Chem., 2019, 89, 148–150 CrossRef CAS.
- W. G. Kofron and C. R. Hauser, J. Org. Chem., 1970, 35, 2085–2086 CrossRef CAS.
- R. A. Kemp, L. Chen, I. Guzei and A. L. Rheingold, J. Organomet. Chem., 2000, 596, 70–76 CrossRef CAS.
- J. Castells, M. Moreno-Mañas and F. Pujol, Tetrahedron Lett., 1978, 19, 385–388 CrossRef.
- A. Miyashita, Y. Suzuki, I. Nagasaki, C. Ishiguro, K.-i. Iwamoto and T. Higashino, Chem. Pharm. Bull., 1997, 45, 1254–1258 CrossRef CAS.
- A. R. Usera, P. M. Dolan, T. W. Kensler and G. H. Posner, Bioorg. Med. Chem., 2007, 15, 5509–5518 CrossRef CAS PubMed.
- U. Huynh, S. L. McDonald, D. Lim, M. N. Uddin, S. E. Wengryniuk, S. Dey and D. M. Coltart, J. Org. Chem., 2018, 83, 12951–12964 CrossRef CAS PubMed.
- C. Roma-Rodrigues, G. Malta, D. Peixoto, L. M. Ferreira, P. V. Baptista, A. R. Fernandes and P. S. Branco, Bioorg. Chem., 2020, 99, 103849 CrossRef CAS PubMed.
- S. P. Pitre, C. D. McTiernan, W. Vine, R. DiPucchio, M. Grenier and J. C. Scaiano, Sci. Rep., 2015, 5, 16397 CrossRef CAS PubMed.
- F. Lucarini, M. Pastore, S. Vasylevskyi, M. Varisco, E. Solari, A. Crochet, K. M. Fromm, F. Zobi and A. Ruggi, Chem. – Eur. J., 2017, 23, 6768–6771 CrossRef CAS PubMed.
- F. Droghetti, F. Begato, M. Raulin, G. Musiu, G. Licini, M. Natali and C. Zonta, Angew. Chem., Int. Ed., 2024, e202408316 Search PubMed.
Footnote |
† Electronic supplementary information (ESI) available: Characterization data of the synthesized ligand and complexes. CCDC 2369954–2369956. For ESI and crystallographic data in CIF or other electronic format see DOI: https://doi.org/10.1039/d4dt02022g |
|
This journal is © The Royal Society of Chemistry 2024 |