DOI:
10.1039/D4DT01006J
(Paper)
Dalton Trans., 2024,
53, 9301-9305
Revisiting the role of octahedral symmetry in the interpretation of spectroscopic properties of [OsF6]2− and PtF6 complexes
Received
5th April 2024
, Accepted 8th May 2024
First published on 8th May 2024
Abstract
The electronic structure of [OsF6]2− and PtF6 complexes was studied by means of CASSCF/NEVPT2 multiconfigurational calculations, including spin–orbital coupling, which is very relevant in the case of these metals. From these calculations, it is possible to establish that in the octahedral symmetry (Oh), the ground state is non-magnetic (Jeff = 0) because of the strong ligand field, and the interaction with paramagnetic excited states is almost negligible, resulting in a non-magnetic behavior, which is in agreement with the experimental evidence.
Introduction
The electronic structure and molecular properties of discrete hexafluorides of late transition metals have been a central topic for both experimentalists and theoreticians for a long period of time.1–6 The central discussion had turned around molecular symmetry and its role in the interpretation of their spectroscopic properties in a series of Re, Os, Ir, and Pt hexafluorides, among others.1–6 The classical matrix isolation work of Holloway et al., using high-resolution spectroscopy techniques, showed that the neutral hexafluorides of those metals are all octahedral (Oh) with two characteristic vibrational modes between 200 and 300 cm−1 and 700 and 750 cm−1, which are minimally affected by Jahn–Teller distortion.1 Similar results were obtained, more recently, by Richardson et al. using gas-phase electron diffraction.4 Moreover, important works have been performed in the past to assign principal absorption bands in these complexes. At this point, the relativistic effect on 5d electrons cannot be negligible and spin–orbit coupling plays a key role in electron redistribution after the splitting of t2g levels.7–11 In an earlier work from 1959, Moffitt et al. interpreted the absorption spectra from a theoretical point of view, explicitly considering the relativistic effects together with ligand-field terms in an intermediate j–j coupling scheme.3 Some years later, Warren et al. performed a similar study considering a strong ligand field with similar results.5 In both cases, good qualitative agreement with experimental results was obtained but not with numerical accuracy.
More recently, with the possibility of solving the four component (4C) Dirac equation to obtain the electronic structure in molecular systems with a relatively large number of electrons, this problem has been occasionally revisited to obtain further insights into the role of relativistic effects on the ground state geometries and electronic configuration in these types of complexes. In 2008, Alvarez-Thon et al. and Restrepo et al. reported a series of comparative theoretical studies of group ten hexafluoride metals, where scalar and spin–orbit relativistic effects were introduced via two and four component Hamiltonian in the framework of mono-determinantal density functional theory (DFT).10,11 In particular, the single determinant two (2C) and four (4C) component approaches in PtF6 predicted a diamagnetic octahedral molecule with a closed-shell ground state, in accordance with the observation of the 19F and 195Pt high-resolution nuclear magnetic resonance (NMR) spectra and its undisturbed IR and Raman spectra of PtF6.10–14
However, the central discussion was centered on nd4 electronic configuration and how spin–orbit coupling is responsible for a non-magnetic ground state, interpreted, within the limitations of the used levels of theory, as a singlet state (strictly in terms of an effective spin, it should be correctly named as a pseudo singlet). The limitations of the mono-configurational approaches were highlighted in the early works mentioned above. For instance, Moffitt et al. suggest in their original work to consider the configuration interaction between both Γ8 states derived from 2Eg and 2T2g electronic states in an nd1 model complex with the aim of improving the models to describe the magnetic properties in MF6 complexes (M = Re, Os, Ir, Pt).3 Similar strategy was used by Eisenstein et al. to improve the theoretically obtained values for 10Dq and spin–orbit coupling constant in ReF6.15 Recently Pedersen et al. reported a detailed study about two hexafluorides of Os (IV and V) in order to disentangle the role of spin–orbit coupling (SOC) on the added electronic states, this time, the electron correlation as a fundamental effect to get a more complete picture about the molecular structure and electronic configuration of the ground state of these complexes.7
Thus, due to the relevance of this topic, a series of ab initio calculations are presented in the present work to obtain a deeper insight into the physicochemical properties of 5d4 hexafluoride metal complexes. The aim of this work is to show that not only relativistic effects but also electron correlation phenomena are fundamental to obtaining a more complete view of the molecular structures and properties of these 5d4 coordination compounds.
Computational details
All the calculations were performed in the ORCA 5.0 code. In order to compute the electronic structure and spectroscopic properties, we used the state-average complete active space self-consistent field (SA-CASSCF)16 method with all-electron basis sets. Def2-TZVPP was used for the fluorine atoms while an all-electron DKH3-QZP-Sapporo basis set was used for Osmium and Platinum.17 The d4 configuration was modeled considering an active space CAS(4,5), four electrons in five d-type orbitals. The calculations include all quintuplet (5), triplet (45), and singlet (50) states derived from the ion configuration. The inclusion of this number of states is related firstly to the need to properly evaluate the effect of spin–orbit coupling in a second stage of the calculation and secondly to the exploration of a higher energy zone in the spectrum of both systems under study directly related to the electronic states derived from the electronic transitions between the ligand-field electronic states transitions (optical spectrum). During the execution of the calculations, different combinations of roots were considered and there were no significant differences between the results obtained, for this reason and appealing to the completeness of the exploration it was decided to use the number of states mentioned above. A second d-shell (6d′) was included in the calculations, which can be useful to make the wave function more flexible and to introduce radial correlation, which is important to obtain accurate results. In the same sense, the 6s shell was also included in the optimization. Dynamical correlation was also included via the N-electron valence perturbation theory at the second order (NEVPT2).18,19 The traditional two-step procedure was used in all calculations. In the first step, spin–orbit-free Hamiltonian calculations were performed, in which scalar relativistic effect and electron correlation are included via the CASSCF/NEVPT2 level of theory. In the second step, the spin–orbit effect was incorporated by the quasi-degenerate perturbation theory (QDPT) approach.20–23
The geometries of both complexes were optimized at the NEVPT2 level of theory, step by step, following the a1g vibrational mode (breathing mode) in the Oh point group. The potential energy surface (PES) was explored starting from 1.700 to 2.000 Å with a step of 0.2 Å. The obtained PES trace was fitted to a second-degree polynomial and the equilibrium distance was obtained as the minimum. In all the optimization procedures together with 5d + 6d′, the 6s orbital of metal ion was included in the calculations. The halide p-orbitals were not included in the optimization because, in all test calculations, they remain double occupied showing no important contribution to the electronic configuration of the ground and excited states.
Results and discussion
In the first step the geometry of both complexes was optimized at the NEVPT2 level of theory, the calculations were performed by scanning the M–F distance following the breathing vibrational mode and considering an octahedral (Oh) symmetry point group. The geometries were obtained in a state-average procedure including all the quintuplets, triplets and singlets derived from the metal ion configuration. As observed from Table 1, the obtained bond distances have a good quality when compared against the experimentally reported values.4,7Table 1 shows, additionally, the importance of the radial correlation in the numerical accuracy of the calculations, especially in the PtF6 complex. The inclusion of the 6s shell is also relevant and, as was explained above, non-significant changes were found when different symmetry-adapted ligand orbitals combinations were included in the active space.
Table 1 Calculated M–F distances at the NEVPT2 level of theory considering different sizes of the active space
Orbitals in active space |
[OsF6]2− |
PtF6 |
See ref. 7 (the value presented here was obtained as an average between the six Os–F distances from the crystallographic data experimentally obtained).
See ref. 4.
|
5d |
1.9576 |
1.8070 |
5d + 6d′ |
1.9558 |
1.8075 |
5d + 6d′+6s |
1.9524 |
1.8528 |
Exp |
1.9476a |
1.850(4)b |
The analysis of the electronic structure shows that the spin orbit-free ground state corresponds to a 3T1g with a configuration (t2g)4(eg)0, well isolated from the first excited state 1T2g in both complexes and it is significantly multiconfigurational in the [OsF6]2− complex (see Table 2). Fig. 1 shows the relative energetic position of the spin–orbit free states up to ∼30
000 cm−1. The ab initio ligand field analysis (AILF)24,25 shows a 10Dq parameter for both complexes in the same range that was previously reported7,10 and is larger in PtF6, because of the shortest Pt–F bond distance as can be seen in Table 2.
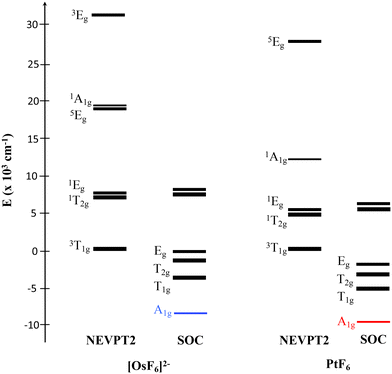 |
| Fig. 1 Electronic state diagram obtained at spin–orbit free NEVPT2 level of theory and considering the spin–orbit coupling (SOC) effect for [OsF6]2− and PtF6 complexes. | |
Table 2 Assignment of the spin–orbit free (SF) states obtained via CAS(4,5)SCF calculations for [OsF6]2− and PtF6 complexes. The active orbitals are ordered as follows: |dxy dyz dxz dz2 dx2−y2|
a
SF-stateb |
Configuration |
[OsF6]2− |
[PtF6] |
The average occupation numbers of 5d orbitals are: [OsF6]2−: t2g 0.847, eg 0.729; PtF6: t2g 0.853, eg 0.721.
The ligand field parameter 10Dq is: this work [OsF6]2−: 32 725 cm−1 (ref. 7. ∼34 000 cm−1). This work PtF6: 36 789 cm−1 (ref. 10 among 28 149 cm−1 (BLYP) – 49 280 cm−1 (B3LYP) obtained from relativistic DFT calculations and different functionals).
|
3T1g (t2g)4(eg)0 |
50%|21 100| + 26%|11 200| + 21%|12 100| |
100%|21 100| |
77%|12 100| + 20%|21 100| |
100%|11 200| |
67%|11 200| + 32%|21 100| |
100%|12 100| |
1T1g (t2g)4(eg)0 |
64%|21 100| + 32%|11 200| |
98%|11 200| |
95%|12 100| |
98%|12 100| |
64%|11 200| + 32%|21 100| |
98%|21 100| |
1Eg (t2g)4(eg)0 |
63%|22 000| + 28%|02 200| |
66%|02 200| + 16%|20 200| + 16%|22 000| |
58%|20 200| + 40%|02 200| |
50%|22 000| + 50%|20 200| |
5Eg (t2g)3(eg)1 |
100%|11 101| |
100%|11 101| |
100%|11 110| |
100%|21 110| |
1A1g (t2g)4(eg)0 |
32%|20 200| + 32%|02 200| + 32%|22 000| |
31%|20 200| + 31%|02 200| + 31%|22 000| |
3Eg (t2g)3(eg)1 |
85%|11 101| + 15%|11110| |
87%|11 101| + 12%|11 110| |
85%|11 110| + 15%|11101| |
87%|11 110| + 12%|11 101| |
From the symmetry point of view when spin–orbit coupling interaction is considered the irreducible representations of the resulting state can be obtained from the direct product ΓS=1 ⊗ T1g which results in A1g ⊕ T1g ⊕ T2g ⊕ Eg (see Fig. 1). The ground state for both molecules studied in this work corresponds to a formally non-magnetic A1g with an effective total angular momentum Jeff = 0. As can be seen from Table 3, the resulting wavefunctions in terms of spin–orbit-free states have a predominant contribution of the triplet states, but non-negligible contributions of singlets and quintuplets were also found. This proves, as mentioned before, that the non-magnetic character of the ground state should not be confused with a singlet assignment for this state. As is well known due to spin–orbit coupling, (SOC) spin is not a good quantum number to characterize molecular electronic states. From the point of view of the analysis of the magnetic properties using effective Hamiltonians, it is usual to use the concept of pseudospin. This does not represent the actual state of the electron spin but also a pseudospin acting in the model space of |MS〉 wavefunctions of the pseudospin projection onto a quantization axis. In the present case, the ground state can be characterized as a pseudo singlet state (Jeff = 0) followed by a first excited pseudo triplet (Jeff = 1). This state, even when magnetic, has an almost negligible population in the temperature range up to 300 K explaining the non-magnetic behavior observed in these complexes. However, the χT product, presented in Fig. 2, formally has a linear dependence with T reflecting a temperature-independent (Van-Vleck) paramagnetism depending on the mixing of the ground Jeff = 0 state with the lowest excited spin–orbit coupling state Jeff = 1. In Fig. 2 it is also possible to appreciate the small variations in χT vs. T in all the temperature range as a result of the negligible population of the excited spin–orbit Jeff = 1 state.
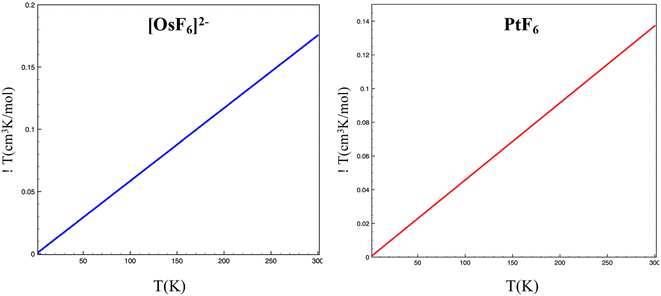 |
| Fig. 2
χT products for [OsF6]2− and PtF6 obtained at the NEVPT2 level of theory. | |
Table 3 Assignment of the spin–orbit (SO) states obtained via CAS(4,5) SCF calculations for [OsF6]2− and PtF6 complexes
SO-statea |
Configuration |
[OsF6]2− |
[PtF6] |
Calculated spin–orbit coupling constants from AILF theory were: [OsF6]2− 3840 cm−1 and PtF6 4162 cm−1.
|
A1g |
81%|3T1g〉 + 12%|1A1g〉 + 4%|5Eg〉 |
89%|3T1g〉 + 10%|1A1g〉 |
T1g |
90%|3T1g〉 + 7%|5Eg〉 |
92%|3T1g〉 + 7%|5Eg〉 |
T2g |
82%|3T1g〉 + 12%|1T2g〉 + 3%|5Eg〉 |
71%|3T1g〉 + 29%|1T2g〉 |
Eg |
84%|3T1g〉 + 9%|1Eg〉 + 3%|5Eg〉 |
77%|3T1g〉 + 22%|1Eg〉 |
Conclusions
From the study of the electronic structure using multiconfigurational methods with the inclusion of spin–orbit coupling it is shown that the molecular structure in these complexes is closely related to the distribution of the electronic states resulting from the cooperative effect of the ligand field, which stabilizes the 3T1g triplet states, and the spin–orbit coupling that lifts the degeneracy of this state leading to a non-degenerate (non-magnetic) molecular ground state A1g with a high multiconfigurational character. In this sense, we can conclude, based on previous works, that defining the metal center in these complexes as a closed shell is not an adequate interpretation and that there is no violation of the Jan-Teller principle in the sense that the ground state is non-degenerate. This justifies the experimental evidence, which concludes a non-magnetic behavior and an octahedral molecular symmetry.
Author contributions
All authors of this manuscript have contributed to the calculations presented and to the discussion of the results. Eduardo Solis-Cespedes: geometry optimization and CASCSF/NEVPT2 calculations. Luis Alvares-Thon: literature review and analysis of results related to spin–orbit coupling. Ramiro Arratia-Pérez: literature review, discussion and analysis of results. Dayán Páez-Hernández: performance of magnetic properties and electronic structure calculations. Review and update the bibliography, analysis of results and writing.
Conflicts of interest
The authors have no conflict of interest to declare.
Acknowledgements
The authors thank DPH. ANID FONDECYT 1220442, LAT. ANID FONDECYT 1191019.
References
- J. H. Holloway, G. Stanger, E. G. Hope, W. Levason and J. S. Ogden, J. Chem. Soc., Dalton Trans., 1988,(5), 1341–1345 RSC
.
- M. V. Korobov, S. V. Kuznetsov, L. N. Sidorov, V. A. Shipachev and V. N. Mit'kin, Int. J. Mass Spectrom. Ion Processes, 1989, 87(1), 13–27 CrossRef CAS
.
- W. Moffitt, G. L. Goodman, M. Fred and B. Weinstock, Mol. Phys., 1959, 2(2), 109–122 CrossRef CAS
.
- A. D. Richardson, K. Hedberg and G. M. Lucier, Inorg. Chem., 2000, 39(13), 2787–2793 CrossRef CAS PubMed
.
-
G. C. Allen and K. D. Warren, Chemical Bonding in Solids, Springer, Berlin, Heidelberg, 1974, pp. 105–165 Search PubMed
.
- R. Craciun, D. Picone, R. T. Long, S. Li, D. A. Dixon, K. A. Peterson and K. O. Christe, Inorg. Chem., 2010, 49(3), 1056–1070 CrossRef CAS PubMed
.
- K. S. Pedersen, D. N. Woodruff, S. K. Singh, A. Tressaud, E. Durand, M. Atanasov, P. Perlepe, K. Ollefs, F. Wilhelm, C. Mathonière, F. Neese, A. Rogalev, J. Bendix and R. Clérac, Chem. – Eur. J., 2017, 23(47)), 11244–11248 CrossRef CAS PubMed
.
- J. L. Pascual, Z. Barandiarán and L. Seijo, J. Chem. Phys., 2006, 124(12), 124315 CrossRef PubMed
.
- S. P. Gabuda and S. G. Kozlova, Phys. Rev. A: At., Mol., Opt. Phys., 2009, 79(5), 056501 CrossRef
.
- L. Alvarez-Thon, J. David, R. Arratia-Pérez and K. Seppelt, Phys. Rev. A: At., Mol., Opt. Phys., 2008, 77, 034502 CrossRef
.
- J. David, P. Fuentealba and A. Restrepo, Chem. Phys. Lett., 2008, 457(1–3), 42–44 CrossRef CAS
.
- R. Fernandes De Farias, Inorg. Chem., 2016, 55(23), 12126–12127 CrossRef CAS PubMed
.
- K. Seppelt and N. Bartlett, Z. Anorg. Allg. Chem., 1977, 436(1), 122–126 CrossRef CAS
.
- T. Drews, J. Supeł, A. Hagenbach and K. Seppelt, Inorg. Chem., 2006, 45(9), 3782–3788 CrossRef CAS PubMed
.
- J. C. Eisenstein, J. Chem. Phys., 1960, 33(5), 1530–1531 CrossRef CAS
.
- B. O. Roos, P. R. Taylor and P. E. M. Sigbahn, Chem. Phys., 1980, 48(2), 157–173 CrossRef CAS
.
- T. Noro, M. Sekiya and T. Koga, Theor. Chem. Acc., 2013, 132(5), 1–5 Search PubMed
.
- C. Angeli, R. Cimiraglia, S. Evangelisti, T. Leininger and J. P. Malrieu, J. Chem. Phys., 2001, 114(23), 10252 CrossRef CAS
.
- C. Angeli, R. Cimiraglia and J. P. Malrieu, Chem. Phys. Lett., 2001, 350(3–4), 297–305 CrossRef CAS
.
- D. Ganyushin and F. Neese, J. Chem. Phys., 2013, 138(10), 104113 CrossRef PubMed
.
- P.Å Malmqvist, B. O. Roos and B. Schimmelpfennig, Chem. Phys. Lett., 2002, 357(3–4), 230–240 CrossRef CAS
.
- B. A. Heß, C. M. Marian, U. Wahlgren and O. Gropen, Chem. Phys. Lett., 1996, 251(5–6), 365–371 CrossRef
.
- F. Neese, J. Chem. Phys., 2005, 122(3), 34107 CrossRef PubMed
.
-
M. Atanasov, D. Ganyushin, K. Sivalingam and F. Neese, Molecular Electronic Structure of Transition Metal Complexes II, Struct. Bonding, 2012, vol. 143, pp. 149–220 Search PubMed
.
- S. K. Singh, J. Eng, M. Atanasov and F. Neese, Coord. Chem. Rev., 2017, 344, 2–25 CrossRef CAS
.
|
This journal is © The Royal Society of Chemistry 2024 |