DOI:
10.1039/D4DT00713A
(Paper)
Dalton Trans., 2024,
53, 12064-12072
Controlled crystallisation of porous crystals of luminescent platinum(II) complexes by electronic tuning of ancillary ligands†
Received
9th March 2024
, Accepted 9th April 2024
First published on 9th April 2024
Abstract
Porous molecular crystals (PMCs) have gained significant importance as next-generation functional porous materials. However, the selective crystallisation of the PMC phase remains a challenge. Herein, we have systematically controlled the stability of the luminescent PMC phase prepared using the luminescent Pt(II) complex [Pt(pbim)(N^O)] (pbim = 2-phenylbenzimidazolate, N^O = N-heteroaryl carboxylate) with Pt⋯Pt electronic interactions. The PMC phase formation varied significantly among the complexes depending on the heteroaryl group of the ancillary N^O ligand; the oxazolyl-bearing complex did not form a PMC phase, whereas the pyrazyl- and 5-fluoropyridyl-bearing complexes spontaneously formed a porous structure. This difference was rationalised by the π-stacking capability of the heteroaryl group of the ancillary ligand. Furthermore, owing to the presence of the one-dimensional Pt⋯Pt chains in this PMC phase, the photophysical properties of PMCs resulting from the Pt⋯Pt interactions were also significantly changed by the ancillary ligands.
Introduction
Porous crystals built from organic or metal–organic units have attracted increasing attention as precisely designable porous materials with diverse functionalities. In particular, porous molecular crystals (PMCs), including hydrogen-bonded organic frameworks (HOFs), have recently gained significant importance as third generation molecular-based porous crystals,1,2 after metal–organic frameworks (MOFs) and covalent organic frameworks (COFs).3 For example, PMCs have been employed as adsorbents,1,2 functional channels,4 sensing/switchable materials,5,6 and catalysts/nanoreactors.7 Notably, in contrast to the poor solubility of most MOFs and COFs, PMCs can be easily dissolved and recrystallised in common solvents. Therefore, PMCs can be easily fabricated via solution processes and reused by recrystallisation.1 However, compared with MOFs and COFs, designing the PMCs is often challenging owing to their construction through weak and diverse intermolecular interactions (e.g., hydrogen bonding and π/π stacking). Despite the meticulous design of building unit molecules, the desired PMC phase may not always be obtained, resulting in unexpected nonporous phases.1 Thus, developing design principles for building unit molecules for controlled crystallisation of the PMC phase remains a challenge.
In this context, we have recently reported the controlled crystallisation of the PMC phase of a luminescent Pt(II) complex at a liquid–liquid interface.8 A Pt(II) complex, [Pt(pbim)(pic)] (Pt-py in Fig. 1(a)), which features a protic 2-phenylbenzimidazolate (pbim) ligand and an ancillary α-picolinate (pic) ligand, was crystallised as a densely packed nonporous phase under conventional crystallisation conditions. In contrast, Pt-py was selectively crystallised as the PMC phase with a one-dimensional hexagonal porous channel (Fig. 1(b)) at the interface between a MeOH/H2O mixture and an alkane via the inclusion of alkane molecules into the hydrophobic pores of the PMC. Notably, this PMC has a short Pt⋯Pt distance (3.4994(4) Å) that facilitates electronic interaction, resulting in the metal–metal-to-ligand charge transfer (MMLCT) absorption and emission. Thus, this PMC is a potential candidate for photofunctional porous materials with a parallel orientation of one-dimensional porous channels and metallophilic chains (Fig. 1(c)). However, crystallisation at the liquid–liquid interface in large-scale preparation is difficult and does not allow for solution processability, which is a typical advantage of PMCs.1 Therefore, further essential improvements are required to obtain the PMC phase more easily.
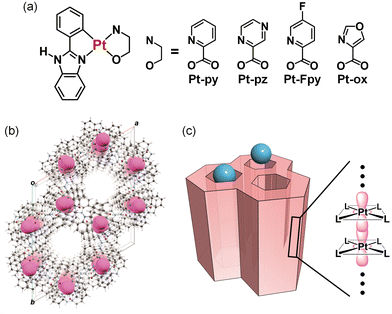 |
| Fig. 1 (a) Structural formulas of Pt-py, Pt-pz, Pt-Fpy, and Pt-ox. (b) Perspective view and (c) schematic representation of the porous structure of the Pt-py crystal. For clarity, Pt atoms in (b) are shown with a space-filling model. Blue balls in (c) represent the guest molecules. | |
In this work, we have developed an easy design to obtain the PMC phase of Pt(II)-pbim complexes based on the electronic tuning of the ancillary ligand. The new Pt(II)-pbim complexes Pt-pz, Pt-Fpy, and Pt-ox (Fig. 1(a)) were synthesised by replacing the pyridyl group of the ancillary ligand of Pt-py with pyrazyl, 5-fluoropyridyl, and oxazolyl groups, respectively. Among the complexes, Pt-pz and Pt-Fpy crystallised as PMC phases without using any special crystallisation methods, such as crystallisation at the liquid–liquid interface. However, Pt-ox never crystallised as a PMC phase. This difference was rationalised by the π/π stacking capability of the ancillary ligand. Furthermore, the absorption/emission properties derived from the intermolecular Pt⋯Pt interactions were also significantly modified by the ancillary ligands.
Experimental Section
Materials
Caution! Although we experienced no difficulties, the chemicals used in this study are potentially harmful and should be used in small quantities and handled with care inside a fume hood. Pyrazinecarboxylic acid (Hpzc), 5-fluoro-α-picolinic acid (HFpic), and oxazole-4-carboxylic acid (Hoxc) were purchased from Tokyo Chemical Industry Co., Ltd. All solvents were purchased from Kanto Chemical Co., Inc. [Pt(pbim)Cl(DMSO)] and [Pt(pbim)(pic)] (Pt-py) were synthesised according to previously reported method.8
Synthesis of [Pt(pbim)(pzc)] (Pt-pz).
A solution of Hpzc (12.5 mg, 0.101 mmol) in water (30 mL) was added to a suspension of [Pt(pbim)Cl(DMSO)] (50.0 mg, 0.0996 mmol) in ethanol (30 mL), and the mixture was refluxed with stirring for 24 h. After cooling to room temperature, the green precipitate was collected by filtration, washed with hot water (ca. 70 °C) and acetone, and dried in vacuo under heating (50 °C) to yield a title compound as a green powder. Yield, 34.4 mg (0.0673 mmol, 68%). 1H NMR (400 MHz, DMF-d7, δ): 9.55 (d, J = 3.2 Hz, 1H), 9.20–9.18 (m, 2H), 8.42 (d, J = 6.9 Hz, 1H), 7.85 (d, J = 6.9 Hz, 1H), 7.78 (d, J = 6.4 Hz, 1H), 7.67 (d, J = 6.9 Hz, 1H), 7.45–7.38 (m, 2H), 7.27–7.24 (m, 2H). Elemental analysis calculated (%) for C18H12N4O2Pt·0.7H2O: C 41.26, H 2.58, N 10.69; Found: C 41.32, H 2.26, N 10.33. Single crystals of Pt-pz were prepared by the slow vapour diffusion of tBuOMe into a solution of Pt-pz in DMF at 4 °C.
Synthesis of [Pt(pbim)(Fpic)] (Pt-Fpy).
This complex was prepared similarly as Pt-pz, except that HFpic (28.1 mg, 0.199 mmol) was used as a precursor and synthesised on a two-fold scale (99.5 mg (0.198 mmol) of [Pt(pbim)Cl(DMSO)]). The compound was obtained as a red-orange powder. Yield, 60.8 mg (0.129 mmol, 65%). 1H NMR (400 MHz, DMF-d7, δ): 9.32 (d, J = 3.2 Hz, 1H), 8.46–8.43 (m, 2H), 8.27–8.20 (m, 1H), 7.83 (dd, J = 1.5, 7.6 Hz, 1H), 7.79–7.73 (m, 1H), 7.64 (d, J = 7.4 Hz, 1H), 7.42–7.39 (m, 2H), 7.30 (td, J = 1.5, 7.5 Hz 1H), 7.24 (t, J = 7.3 Hz, 1H). Elemental analysis calculated (%) for C19H12N3O2FPt: C 43.19, H 2.29, N 7.95; Found: C 43.20, H 2.24, N 7.70. Single crystals of Pt-Fpy were prepared by the slow vapour diffusion of tBuOMe into a solution of Pt-Fpy in DMF at 4 °C.
Synthesis of [Pt(pbim)(oxc)] (Pt-ox).
This complex was prepared similarly as Pt-pz, except that Hoxc (11.9 mg, 0.105 mmol) was used as the precursor. The compound was obtained as an off-white powder. Yield, 31.2 mg (0.0624 mmol, 63%). 1H NMR (400 MHz, DMSO-d6, δ): 9.86 (s, 1H), 8.88 (s, 1H), 8.20 (d, J = 5.0 Hz, 1H), 7.77–7.62 (m, 3H), 7.39–7.36 (m, 2H), 7.20 (t, J = 7.4 Hz, 1H), 7.11 (t, J = 7.5 Hz, 1H). Elemental analysis calculated (%) for C17H11N3O3Pt: C 40.81, H 2.21, N 8.39; Found: C 40.50, H 2.09, N 8.19. Single crystals of Pt-ox were prepared by the slow vapour diffusion of tBuOMe into a solution of Pt-ox in DMF at 4 °C.
Apparatus
Single crystal X-ray structure analysis.
Single crystal X-ray diffraction measurements were performed using a Rigaku XtaLAB-Synergy diffractometer with a HyPix-6000HE area detector and a multilayer mirror-monochromated Cu Kα radiation (λ = 1.54184 Å) (for Pt-pz and Pt-ox) or a Rigaku XtaLAB AFC11 (RCD3) diffractometer with a multilayer mirror-monochromated Mo Kα radiation (λ = 0.71073 Å) (for Pt-Fpy). Each crystal was mounted on a MicroMount using Paratone-N oil and cooled using an N2-flow type temperature controller. Diffraction data were collected and processed using CrysAlisPro.9 The structures were solved using SHELXT-201810 by the intrinsic phasing method. Structural refinements were conducted using the full-matrix least-squares technique in SHELXL-2018.11 All non-hydrogen atoms were refined anisotropically, and the hydrogen atoms were refined using the riding model. For Pt-pz and Pt-Fpy, the diffuse electron densities resulting from the residual solvent molecules were removed from the dataset using the SQUEEZE routine12a of PLATON12b and further refined using the generated data. The crystallographic data for each complex are listed in Table S1.† Full crystallographic data have been deposited with the Cambridge Crystallographic Data Centre (CCDC 2333937 for Pt-pz, 2333938 for Pt-Fpy, and 2333939 for Pt-ox
†).
Absorption and emission measurements.
The UV-vis absorption spectra were recorded using a Hitachi U-3000 or Shimadzu UV-2500PC spectrophotometers. The UV-vis diffuse reflectance spectra were recorded using a Hitachi U-3000 spectrophotometer equipped with an integrating sphere apparatus. For diffuse reflectance spectroscopy, the solid samples were diluted with MgO, and the measured reflectivity was converted using the Kubelka–Munk function. The emission spectra were acquired using a JASCO FP-8600 or FP-6600 spectrofluorometers. The typical slit width of the excitation and emission lights was 5 nm. The luminescence quantum yields were recorded using a Hamamatsu Photonics C9920-02 absolute photoluminescence quantum yield measurement system equipped with an integrating sphere apparatus and a 150 W CW xenon light source. The accuracy of the instrument was confirmed based on a measurement of the quantum yield of anthracene in ethanol (Φ = 0.27).13 Emission lifetime measurements were conducted using a Hamamatsu Photonics Quantaurus-Tau C11367 fluorescence lifetime spectrometer excited by an internal light-emitting diode (LED) light source (λex = 405 nm). The emission decays were analysed using two exponentials: I = A1exp
(–t/τ1) + A2exp
(–t/τ2), where Ai (i = 1, 2) denotes the pre-exponential factors for lifetimes τi. The average emission lifetimes (τav) were calculated using the following equation: τav = (A1τ12 + A2τ22)/(A1τ1 + A2τ2).14
Other measurements.
The 1H NMR spectra were measured using a JEOL JNM-ECZ400S NMR spectrometer at room temperature, where chemical shifts were referenced to the internal tetramethylsilane. Thermogravimetric (TG) analyses were conducted using Rigaku Thermoplus EVO TG-DTA8120 or Thermoplus EVO2 TG-DTA8122 instruments under an Ar flow (0.3 L min−1). The nitrogen adsorption was measured using a MicrotracBEL BELSORP-max automatic volumetric adsorption apparatus. The t-plot method was used to determine the micropore diameter. Powder X-ray diffraction (PXRD) measurements were conducted using Cu Kα radiation (λ = 1.5418 Å) on a Bruker D8 Advance diffractometer equipped with a graphite monochromator and a one-dimensional LynxEye detector. Elemental analysis was performed using an Exeter Analytical CE440 elemental analyser at the Analysis Centre at Hokkaido University.
Computational methods
The density functional theory (DFT) and the time-dependent DFT (TDDFT) calculations were performed using the Gaussian16 program.15 The B3LYP-D3BJ functional16 was used for ground-state structure optimisations. The SDD basis set17 and its associated effective core potentials were applied for Pt, and the 6-311+G(d,p) basis set18 was used for the other atoms. All structure optimisations were performed without any constraints. The solvation model density (SMD)19 continuum model was employed as the implicit solvation model with methanol as the solvent for the monomers. Vibrational frequency calculations were performed to confirm the nature of the optimised ground state minima (i.e., no imaginary frequencies). For the dimers and trimers, single-point DFT calculations were performed in the gas phase for the experimental crystal geometries without any optimisations. The interaction energies (IE) were calculated as the difference between the total energy of the two molecules (EAB) and that of each molecule in an isolated state (EA, EB) as follows: IE = EAB − (EA + EB). Correction for the basis set superposition errors (BSSE) was performed using the counterpoise method.20
Results and discussion
Synthesis and crystal structures
The desired complexes Pt-pz, Pt-Fpy, and Pt-ox were obtained by reacting [Pt(pbim)Cl(DMSO)] with the corresponding N-heteroaryl carboxylic acids in a water/ethanol mixture. Although Pt-py and most other related Pt(II)-pic complexes are primarily synthesised in aprotic solvents (e.g., acetonitrile or acetone) using Na2CO3 as the base,8,21Pt-ox could not be purely obtained using this method. Thus, the synthetic procedures for Pt-ox, Pt-pz, and Pt-Fpy were improved by employing a water/ethanol mixed solvent, which has proton-accepting ability. The single crystals of the complexes were obtained by recrystallising from DMF/tBuOMe in a refrigerator.
The molecular and packing structures were investigated by X-ray crystallographic analyses, as shown in Fig. 2(a–c) and Fig. S1.† For all complexes, the Pt atoms adopted a typical four-coordinate square-planar geometry coordinated by a pbim ligand and an ancillary heteroaryl carboxylate ligand. Although their molecular structures are similar, their packing structures differ significantly depending on the ancillary ligand. Notably, in contrast to a previous Pt-py, which yielded different crystal pseudo-polymorphs depending on the crystallisation methods,8 the packing structures of Pt-pz, Pt-Fpy, and Pt-ox were independent of the crystallisation method.
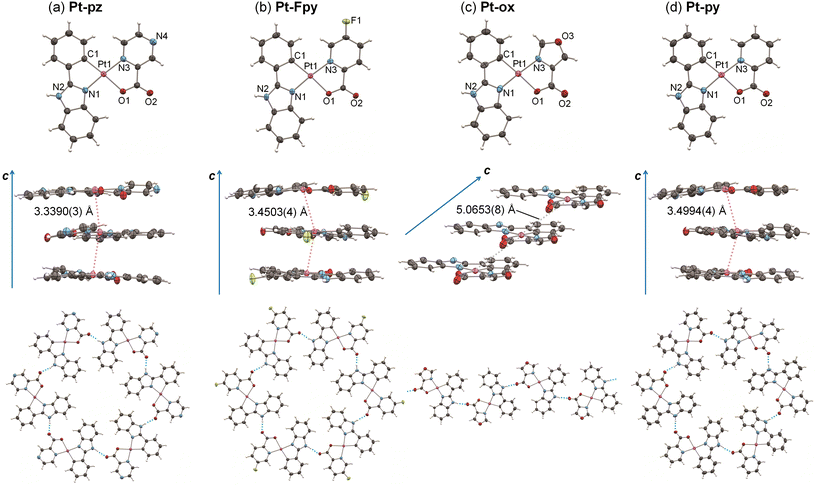 |
| Fig. 2 Molecular (top), stacking (middle), and hydrogen-bonded (bottom) structures of (a) Pt-pz, (b) Pt-Fpy, (c) Pt-ox, and (d) Pt-py (cyclohexane-included porous form; reported in ref. 8). Thermal ellipsoids are displayed at the 50% probability level. | |
Among the present complexes, Pt-pz and Pt-Fpy crystallised in the trigonal R
space group (Fig. 2(a and b) and Table 1), same as the porous form of Pt-py (Fig. 2(d)). In these crystals, the Pt(II) complex molecules are stacked one-dimensionally in parallel to a 3-fold screw axis (Fig. S1(a) and (b)†). The intermolecular Pt⋯Pt distances of Pt-pz (3.3390(3) Å) and Pt-Fpy (3.4503(4) Å) were shorter than twice the van der Waals radius of Pt (3.50 Å),22 revealing the presence of the Pt⋯Pt interactions. On the other hand, these complexes formed self-assembled cyclic hexamers through hydrogen bonding (bottom of Fig. 2(a and b)), and this hexamer was aligned in the ab plane to form a honeycomb-like sheet (Fig. S1(c)†). In addition, F⋯F interactions and CH⋯F weak hydrogen bonds in the ab-plane were also observed for Pt-Fpy (Fig. S1(d)†). As a result, one-dimensional hexagonal channels were formed in both Pt-pz and Pt-Fpy crystals with pore diameters of 7.2 Å and 7.1 Å and void fractions of 17.5% and 17.0%, respectively (Fig. 3 and Fig. S2†). Thus, Pt-pz and Pt-Fpy crystals could be categorised as PMCs, despite their porosity not being as large as that of typical PMCs.1 Notably, whereas several PMCs collapse their porous structures upon desorption of solvent molecules,1 in the present case, the porous structure was retained even after the desorption of guest molecules from the porous channel (that is, permanent porosity; Fig. S3†). Therefore, the template effect of the solvent molecules should not be critical in obtaining the present PMC structure. In addition, the Pt⋯Pt distance decreased, and the Pt⋯Pt⋯Pt angle increased in the order of Pt-py, Pt-Fpy, and Pt-pz (Table 1). Among the complexes, Pt-pz is considered to have the strongest Pt⋯Pt interaction, followed by Pt-Fpy and Pt-py.
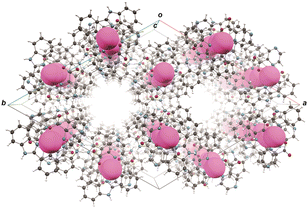 |
| Fig. 3 Porous structure of Pt-pz. Thermal ellipsoids are displayed at the 50% probability level. For clarity, Pt atoms are shown using a space-filling model. | |
Table 1 Lattice constants and important parameters of Pt-pz, Pt-Fpy, and Pt-py (cyclohexane-included porous form)
|
Pt-pz
|
Pt-Fpy
|
Pt-py
|
Reported in ref. 8.
|
Crystal system |
Trigonal |
Trigonal |
Trigonal |
Space group |
R (#148) |
R (#148) |
R (#148) |
a, b/Å |
30.2788(4) |
30.568(1) |
30.2896(3) |
c/Å |
9.8954(1) |
9.9905(4) |
10.0570(1) |
V/Å3 |
7856.7(2) |
8084.6(6) |
7990.7(2) |
Pt⋯Pt distance/Å |
3.3390(3) |
3.4503(4) |
3.4994(4) |
Pt⋯Pt⋯Pt angle/deg |
164.53(2) |
153.81(2) |
151.23(2) |
Void fraction/% |
17.5 |
17.0 |
16.9 |
Pore diameter/Å |
7.2 |
7.1 |
7.0 |
In contrast, Pt-ox crystallised in the orthorhombic P212121 space group (Fig. 2(c)). In this crystal, the Pt-ox molecules were stacked along the c-axis in an oblique manner, wherein the dihedral angle between the molecular plane of Pt-ox and the (0 0 1) plane was 50.1°. The intermolecular Pt⋯Pt distance of Pt-ox (5.0653(8) Å) was significantly longer than twice the van der Waals radius of Pt, indicating the negligible Pt⋯Pt electronic interactions. In contrast to Pt-pz and Pt-Fpy, which formed porous structures built from supramolecular cyclic hexamers, Pt-ox adopted a nonporous structure composed of a hydrogen-bonded one-dimensional ribbon-like structure (bottom of Fig. 2(c)). Notably, all attempts to obtain porous crystals of Pt-ox were unsuccessful, even when the complex crystallised at the liquid–liquid interface, as in the case of Pt-py.
Selectivity of crystallisation
We then evaluated the selectivity of crystallisation between the porous and nonporous forms. As mentioned in the Introduction, the PMC phase of Pt-py could only be obtained on the liquid–liquid interface between alkanes and a water/methanol mixture, whereas a nonporous structure was obtained as-synthesised or by conventional crystallisation8 (red line in Fig. 4). In contrast, packing structures of all the present complexes Pt-pz, Pt-Fpy, and Pt-ox were independent of the crystallisation method. Therefore, the as-synthesised crystal structures of the complexes were investigated using PXRD (blue, green, and orange lines in Fig. 4). The PXRD patterns of the as-synthesised Pt-pz, Pt-Fpy, and Pt-ox agreed with the patterns simulated from the single-crystal X-ray structures. This implies that the crystalline components of the bulk samples of the as-synthesised Pt-pz, Pt-Fpy, and Pt-ox should have the same structures as the X-ray structures.
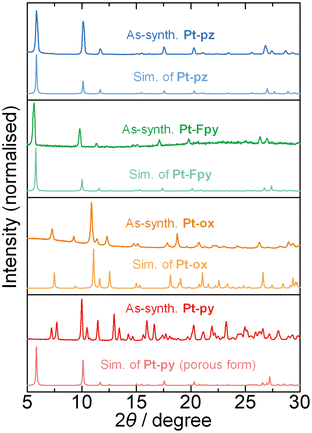 |
| Fig. 4 PXRD patterns of as-synthesised Pt-pz (blue), Pt-Fpy (green), Pt-ox (orange), and Pt-py (red), as well as the simulated PXRD patterns based on the corresponding single crystal X-ray structures of Pt-pz (pale blue), Pt-Fpy (pale green), Pt-ox (pale orange), and Pt-py (pale red). | |
The nitrogen adsorption isotherms of Pt-pz and Pt-Fpy were measured to evaluate the porosity and bulk purity of the as-synthesised forms. As shown in Fig. 5(a), the as-synthesised Pt-pz displayed type-I adsorption isotherm,23 indicating the presence of micropores even in the as-synthesised form. The Brunauer–Emmett–Teller (BET) surface area of the as-synthesised Pt-pz was estimated to be 130 m2 g−1, and the calculated pore size of 7.5 Å was consistent with the X-ray crystallographic result. In contrast, almost no N2 adsorption was observed for the as-synthesised Pt-Fpy (Fig. 5(b)), suggesting almost no porosity in this form. Notably, although the PXRD peaks of the as-synthesised Pt-Fpy were consistent with the simulated ones, the presence of a halo at around 20–30° indicated the co-existence of an amorphous component (Fig. 4 and Fig. S4†). Thus, the porous crystalline phase should be a minor component of the as-synthesised Pt-Fpy, with the majority in the nonporous amorphous form. Indeed, the recrystallised sample of Pt-Fpy showed type-I adsorption behaviour similar to that of Pt-pz (Fig. 5(b)), with a calculated BET surface area of 169 m2 g−1 and a pore size of 9.8 Å.
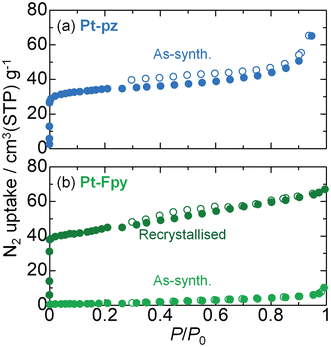 |
| Fig. 5 Nitrogen adsorption isotherms of (a) Pt-pz and (b) Pt-Fpy at 77 K (filled circles: adsorption. Open circles: desorption). The samples were evacuated at 150 °C for 24 h before the measurements. | |
Based on these results, the crystallisation selectivity of the four complexes can be summarised as follows: Pt-pz spontaneously formed a porous crystal structure even in its as-synthesised form. Pt-Fpy contained a significant amount of amorphous powder in the as-synthesised form; however, a porous structure was easily obtained by recrystallisation. In contrast, Pt-py could not be obtained as a porous structure by conventional crystallisation methods and required the inclusion of alkane molecules as guests on the liquid–liquid interface.8Pt-ox could not adopt a porous structure, and only the nonporous crystal structure was selectively obtained. Why does the ancillary ligand alter the selectivity of the obtained crystal structure?
To discuss the origin of this selectivity, the stacked structures of the complexes were compared. First, the number of pairs of atoms in the ligand whose interatomic distances were shorter than the sum of the van der Waals radii was compared between the neighbouring complexes (Fig. S5†). As a result, the number of close atoms increased in the order of Pt-py (3 pairs) < Pt-Fpy (6 pairs) < Pt-pz (9 pairs), indicating the enhanced π/π interactions from Pt-py to Pt-pz. Indeed, the calculated interaction energies (IEs) between the stacked molecules increased in the order Pt-py (IE = −151 kJ mol−1) < Pt-Fpy (−164 kJ mol−1) ≤ Pt-pz (−166 kJ mol−1). The stronger intermolecular interactions in Pt-pz and Pt-Fpy crystals compared to Pt-py were further confirmed by the higher decomposition temperatures of Pt-pz (ca. 318 °C) and Pt-Fpy (ca. 316 °C) than that of Pt-py (ca. 225 °C) (Fig. S6†). As summarised in Fig. 6, the pyrazine ring on the ancillary ligand of Pt-pz formed π/π and anion/π interactions with electron-rich substituents, such as phenyl and carboxylate groups, where these π/π interactions were relatively weaker in Pt-py (Fig. S5†). Among these complexes, the N-heteroaryl ring on the ancillary ligand was the most electron deficient in Pt-pz, followed by Pt-Fpy and Pt-py (Fig. S7 and S8†), where such electron deficiency of the aromatic ring enhances the π/π interaction with the electron-rich substituent.24,25 Therefore, the electron-deficient pyrazine ring on Pt-pz would increase the π/π interactions, leading to the preferential formation of the porous structure. Although the electron deficiency of Pt-Fpy is relatively weaker than that of Pt-pz, the presence of F⋯F and CH⋯F interactions (Fig. S1(d)†) also supports the formation of porous structures during conventional recrystallisation. For Pt-ox, the oxazolyl group is too small to form the π/π stacking with neighbouring molecules (Fig. S5(d)†); this prevents the formation of this porous structure and results in another packing structure. The relationship between the ancillary ligand and formation of the target PMC phase is summarised in Scheme 1.
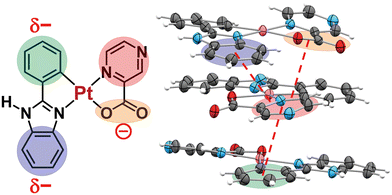 |
| Fig. 6 Stacking structure of Pt-pz, where the parts indicated in red, green, blue, and orange correspond to pyrazyl, phenyl, benzimidazolyl, and carboxylate groups, respectively. | |
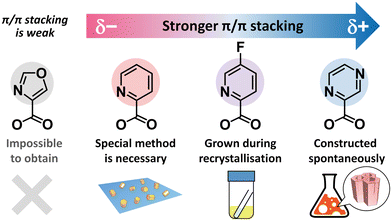 |
| Scheme 1 Summary of the tendency of the crystallisation of the PMC phase of the present complexes depending on the ancillary ligand. | |
Absorption and emission properties
The above-mentioned differences in the intermolecular interactions between complexes also affect the photophysical properties. As shown in Fig. 7(a), the absorption edges in the solid state were red-shifted in the following order: Pt-pz (850 nm), Pt-Fpy (590 nm), Pt-py (525 nm; porous form), and Pt-ox (405 nm). For Pt-pz, Pt-Fpy, and Pt-py, the broad absorption bands at longer wavelengths in the solid state were not observed in the absorption spectra of the methanol solution (Fig. 7(b) and Fig. S9†). Thus, these broad absorption bands in the solid state could be attributable to the 1MMLCT band arising from the intermolecular Pt⋯Pt interactions in the crystal, while the lowest-energy absorption bands in the solution could be assignable to the monomer-based singlet metal-to-ligand charge transfer (1MLCT) bands. These assignments are also supported by theoretical calculations (Fig. S10 and S11†). The absorption edges of Pt-pz, Pt-Fpy, and Pt-py in the solid state are considered to be shifted by the strength of the Pt⋯Pt interactions and the π* energy of the ancillary ligands. In particular, the absorption edge of Pt-pz in the solid state extends to the near-infrared (NIR) region. Conversely, the absorption edge of Pt-ox, which has no Pt⋯Pt interaction in the crystal (5.0653(8) Å), hardly changed between the solid state and solution. As a result, the colour of the crystals changed drastically from dark green (Pt-pz) to red-orange (Pt-Fpy), orange (Pt-py), and off-white (Pt-ox), depending on the ancillary ligand (Fig. 7(c)).
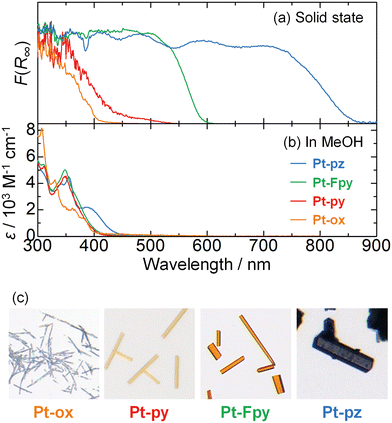 |
| Fig. 7 (a and b) UV-vis absorption spectra of Pt-pz (blue), Pt-Fpy (green), Pt-ox (orange), and Pt-py (red; porous form) in the (a) solid state and (b) methanol solution (2.0 × 10−5 M). (c) Photographs of the crystals of the present complexes. | |
Among the present complexes, Pt-Fpy, Pt-py, and Pt-ox exhibited observable emission in the solid state at ambient temperature (293 K), whereas the Pt-pz crystals did not show any emission, even at 77 K. As shown in Fig. 8(a), crystals of Pt-Fpy (λem = 670 nm) and Pt-py (λem = 690 nm; porous form) exhibited broad, structureless emission bands, whereas Pt-ox displayed a vibronically structured emission band in the solid state (λem = 480, 520, 560 nm). In contrast, under dilute conditions (i.e., rigid MeOH/EtOH (v/v = 1/1) glass at 77 K; Fig. 8(c)), all complexes exhibited essentially similar emission spectra with vibronic structures, which could be attributed to the emission from the ligand-centred 3ππ* excited state. Thus, the emission origin of Pt-ox can also be assignable to the ligand-centred 3ππ* excited state, and the emission bands of Pt-Fpy and Pt-py can be attributed to the 3MMLCT emissions. Indeed, a large radiative rate constant (kr) of Pt-Fpy (1.3 × 106 s−1; Table 2) is a typical value of the 3MMLCT emission of self-assembled Pt(II) complexes,26 while a moderate kr value of Pt-ox (1.0 × 104 s−1; Table 2) is characteristic of the purer ligand-centred 3ππ* emission of discrete Pt(II) complexes.27 In addition, although the absorption edge of Pt-Fpy was observed at longer wavelengths than that of Pt-py (Fig. 7(a)), the emission maximum of Pt-Fpy (670 nm) was slightly shifted to a higher energy than that of Pt-py (690 nm), indicating a smaller Stokes shift for Pt-Fpy. Thus, the structural deformation during the excitation was slightly suppressed in Pt-Fpy compared with Pt-py, probably due to the enhancement of π/π interactions and the presence of CH⋯F and F⋯F interactions in the crystals (see above). Notably, these 3MMLCT emission bands exhibited a significant red-shift at 77 K (Fig. 8(b)). Such thermochromic shift was widely observed in the 3MMLCT emission bands of one-dimensionally stacked Pt(II) complexes due to the shortening of the Pt⋯Pt distance at 77 K.28 Overall, although Pt-pz, Pt-Fpy, and Pt-py were found to have similar porous structures, their photophysical properties based on the Pt⋯Pt interactions could be drastically tuned by the ancillary ligand.
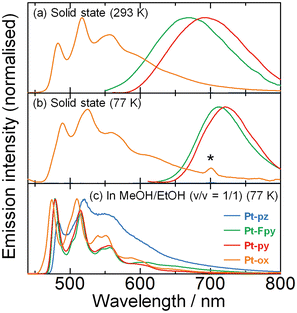 |
| Fig. 8 Emission spectra (λex = 350 nm) of Pt-pz (blue), Pt-Fpy (green), Pt-ox (orange), and Pt-py (red; porous form) in the solid state (a) at 293 K, (b) at 77 K, and (c) in the MeOH/EtOH (v/v = 1/1) glass at 77 K (2.0 × 10−5 M). The peak indicated with an asterisk (*) is an artifact from the spectrometer. | |
Table 2 Photophysical data of Pt-ox, Pt-Fpy, and Pt-py (porous form) in the solid state at 293 K
|
Pt-ox
|
Pt-Fpy
|
Pt-py (porous form)a |
Reported in ref. 8.
Emission maximum wavelengths.
Emission quantum yields.
Emission lifetimes.
Pre-exponential factors.
Averaged emission lifetimes.
Radiative rate constants, kr = Φ/τav.
Nonradiative rate constants, knr = kr(1 − Φ)/Φ.
|
λ
em
/nm |
480, 520, 560 |
670 |
690 |
Φ
|
0.01 |
0.01 |
<0.01 |
τ
/ns (A e) |
3.4 × 102 (0.94), 2.4 × 103 (0.06) |
3.5 (0.68), 10.4 (0.32) |
3.3 (0.77), 6.7 (0.22) |
τ
av
/ns |
9.8 × 102 |
7.6 |
4.5 |
k
r
/s−1 |
1.0 × 104 |
1.3 × 106 |
— |
k
nr
/s−1 |
1.0 × 106 |
1.3 × 108 |
— |
Conclusions
In conclusion, we have successfully controlled the crystallisation of the PMC phase of self-assembled Pt(II)-pbim complexes based on an ancillary ligand. Among the present complexes, Pt-pz and Pt-Fpy were successfully assembled into porous frameworks with one-dimensional channels without special crystallisation techniques, unlike Pt-py. However, Pt-ox did not form such a PMC phase and instead resulted in a densely packed nonporous structure. This difference in crystallisation selectivity is due to the enhanced π/π stacking resulting from the electron deficiency of the ancillary ligand, especially for Pt-pz. The obtained porous structure possessed a one-dimensional Pt⋯Pt chain, where the strength of the interaction increased from Pt-py to Pt-Fpy and Pt-pz. Due to the differences in the Pt⋯Pt interactions and the π*-levels of the ancillary ligand, these PMCs exhibit distinct colours and emissions. These results provide design guidelines for efficiently and selectively obtaining luminescent PMCs. Furthermore, the present PMCs should be potential candidates for the stimuli-responsive functional “soft crystals”,29 including sensing materials for guest molecules and photoconductive materials based on the photoelectron transfer from the Pt⋯Pt chain to the guest molecules in the pore. Further studies of Pt(II)-based photofunctional PMCs for these applications are currently underway.
Conflicts of interest
There are no conflicts to declare.
Acknowledgements
This work was supported by JSPS KAKENHI, grant numbers JP17H06367, JP20H05082, JP21K05094, and JP22H02098, and also the Hyogo Science and Technology Association. The authors are grateful to Ms. M. Kimura (Hokkaido Univ.) for her contributions in the synthesis of Pt-pz at the initial stage of this work. The authors are also grateful to Prof. H. Ito and Dr M. Jin (Hokkaido Univ.) for the support regarding the X-ray crystallographic analysis of Pt-Fpy. Supercomputing resources at the Research Centre for Computational Science, Okazaki, Japan are also acknowledged (Project: 23-IMS-C036).
References
-
(a) M. W. Hosseini, Acc. Chem. Res., 2015, 38, 313–323 CrossRef PubMed;
(b) M. I. Hashim, C.-W. Hsu, H. T. M. Le and O. Š. Miljanić, Synlett, 2016, 1907–1918 CAS;
(c) T. Adachi and M. D. Ward, Acc. Chem. Res., 2016, 49, 2669–2679 CrossRef CAS PubMed;
(d) Y.-F. Han, Y.-X. Yuan and H.-B. Wang, Molecules, 2017, 22, 266 CrossRef PubMed;
(e) I. Hisaki, C. Xin, K. Takahashi and T. Nakamura, Angew. Chem., Int. Ed., 2019, 58, 11160–11170 CrossRef CAS PubMed;
(f) R.-B. Lin, Y. He, P. Li, H. Wang, W. Zhou and B. Chen, Chem. Soc. Rev., 2019, 48, 1362–1389 RSC;
(g) L. Chen, B. Zhang, L. Chen, H. Liu, Y. Hu and S. Qiao, Mater. Adv., 2022, 3, 3680–3708 RSC;
(h) Z. Zhang, Y. Ye, S. Xiang and B. Chen, Acc. Chem. Res., 2022, 55, 3752–3766 CrossRef CAS PubMed.
-
(a) P. Sozzani, S. Bracco, A. Comotti, L. Ferretti and R. Simonutti, Angew. Chem., Int. Ed., 2005, 44, 1816–1820 CrossRef CAS PubMed;
(b) P. Dechambenoit, S. Ferlay, N. Kyritsakas and M. W. Hosseini, J. Am. Chem. Soc., 2008, 130, 17106–17113 CrossRef CAS PubMed;
(c) Y. He, S. Xiang and B. Chen, J. Am. Chem. Soc., 2011, 133, 14570–14573 CrossRef CAS PubMed;
(d) M. Mastalerz and I. M. Oppel, Angew. Chem., Int. Ed., 2012, 51, 5252–5255 CrossRef CAS PubMed;
(e) K. Raatikainen and K. Rissanen, Chem. Sci., 2012, 3, 1235–1239 RSC;
(f) I. Bassanetti, A. Comotti, P. Sozzani, S. Bracco, G. Calestani, F. Mezzadri and L. Marchio, J. Am. Chem. Soc., 2014, 136, 14883–14895 CrossRef CAS PubMed;
(g) A. Karmakar, R. Illathvalappil, B. Anothumakkool, A. Sen, P. Samanta, A. V. Desai, S. Kurungot and S. K. Ghosh, Angew. Chem., Int. Ed., 2016, 55, 10667–10671 CrossRef CAS PubMed;
(h) F. Hu, C. Liu, M. Wu, J. Pang, F. Jiang, D. Yuan and M. Hong, Angew. Chem., Int. Ed., 2017, 56, 2101–2104 CrossRef CAS PubMed;
(i) H. Yamagishi, H. Sato, A. Hori, Y. Sato, R. Matsuda, K. Kato and T. Aida, Science, 2018, 361, 1242–1246 CrossRef CAS PubMed.
-
(a) S. Kitagawa, R. Kitaura and S. Noro, Angew. Chem., Int. Ed., 2004, 43, 2334–2375 CrossRef CAS PubMed;
(b) O. M. Yaghi, M. O'Keeffe, N. W. Ockwig, H. K. Chae, M. Eddaoudi and J. Kim, Nature, 2003, 423, 705–714 CrossRef CAS PubMed;
(c) X. Feng, X. Ding and D. Jiang, Chem. Soc. Rev., 2012, 41, 6010–6022 RSC;
(d) P. J. Waller, F. Gándara and O. M. Yaghi, Acc. Chem. Res., 2015, 48, 3053–3063 CrossRef CAS PubMed.
-
(a) S. Takamizawa, T. Akatsuka and U. Ueda, Angew. Chem., Int. Ed., 2008, 47, 1689–1692 CrossRef CAS PubMed;
(b) S. Tashiro, R. Kubota and M. Shionoya, J. Am. Chem. Soc., 2012, 134, 2461–2464 CrossRef CAS PubMed;
(c) M. Tadokoro, Y. Ohata, Y. Shimazaki, S. Ishimaru, T. Yamada, Y. Nagao, T. Sugaya, K. Isoda, Y. Suzuki, H. Kitagawa and H. Matsui, Chem. – Eur. J., 2014, 20, 13698–13709 CrossRef CAS PubMed;
(d) S. Chand, S. C. Pal, A. Pal, Y. Ye, Q. Lin, Z. Zhang, S. Xiang and M. C. Das, Chem. – Eur. J., 2019, 25, 1691–1695 CrossRef CAS PubMed;
(e) T. Takeda, M. Ozawa and T. Akutagawa, Angew. Chem., Int. Ed., 2019, 58, 10345–10352 CrossRef CAS PubMed;
(f) T. Seki, K. Ida, H. Sato, S. Aono, S. Sakaki and H. Ito, Chem. – Eur. J., 2020, 26, 735–744 CrossRef CAS PubMed.
-
(a) C. H. Hendon, K. E. Wittering, T.-H. Chen, W. Kaveevivitchai, I. Popov, K. T. Butler, C. C. Wilson, D. L. Cruickshank, O. Š. Miljanić and A. Walsh, Nano Lett., 2015, 15, 2149–2154 CrossRef CAS PubMed;
(b) T. Ogoshi, Y. Shimada, Y. Sakata, S. Akine and T. Yamagishi, J. Am. Chem. Soc., 2017, 139, 5664–5667 CrossRef CAS PubMed;
(c) I. Hisaki, Y. Suzuki, E. Gomez, Q. Ji, N. Tohnai, T. Nakamura and A. Douhal, J. Am. Chem. Soc., 2019, 141, 2111–2121 CrossRef CAS PubMed;
(d) H. Sasaki, H. Imoto, T. Kitao, T. Uemura, T. Yumura and K. Naka, Chem. Commun., 2019, 55, 6487–6490 RSC;
(e) M. Nakaya, W. Kosaka, H. Miyasaka, Y. Komatsumaru, S. Kawaguchi, K. Sugimoto, Y. Zhang, M. Nakamura, L. F. Lindoy and S. Hayami, Angew. Chem., Int. Ed., 2020, 59, 10658–10665 CrossRef CAS PubMed;
(f) B. Wang, R. He, L.-H. Xie, Z.-J. Lin, X. Zhang, J. Wang, H. Huang, Z. Zhang, K. S. Schanze, J. Zhang, S. Xiang and B. Chen, J. Am. Chem. Soc., 2020, 142, 12478–12485 CrossRef CAS PubMed;
(g) Y. Wang, D. Liu, J. Yin, Y. Shang, J. Du, Z. Kang, R. Wang, Y. Chen, D. Sun and J. Jiang, Chem. Commun., 2020, 56, 703–706 RSC;
(h) C. Wang, X. Song, Y. Wang, R. Xu, X. Gao, C. Shang, P. Lei, Q. Zeng, Y. Zhou, B. Chen and P. Li, Angew. Chem., Int. Ed., 2023, 62, e202311482 CrossRef CAS PubMed.
-
(a) M. Kato, S. Kishi, Y. Wakamatsu, Y. Sugi, Y. Osamura, T. Koshiyama and M. Hasegawa, Chem. Lett., 2005, 34, 1368–1369 CrossRef CAS;
(b) Y. Shigeta, A. Kobayashi, T. Ohba, M. Yoshida, T. Matsumoto, H.-C. Chang and M. Kato, Chem. – Eur. J., 2016, 22, 2682–2690 CrossRef CAS PubMed;
(c) Y. Shigeta, A. Kobayashi, M. Yoshida and M. Kato, Cryst. Growth Des., 2018, 18, 3419–3427 CrossRef CAS.
-
(a) P. Chinapang, M. Okamura, T. Itoh, M. Kondo and S. Masaoka, Chem. Commun., 2018, 54, 1174–1177 RSC;
(b) H. Yonezawa, S. Tashiro, T. Shiraogawa, M. Ehara, R. Shimada, T. Ozawa and M. Shionoya, J. Am. Chem. Soc., 2018, 140, 16610–16614 CrossRef CAS PubMed;
(c) W. He, S. Tashiro and M. Shionoya, Chem. Sci., 2022, 13, 8752–8758 RSC;
(d) W. Liang, F. Carraro, M. B. Solomon, S. G. Bell, H. Amenitsch, C. J. Sumby, N. G. White, P. Falcaro and C. J. Doonan, J. Am. Chem. Soc., 2019, 141, 14298–14305 CrossRef CAS PubMed;
(e) B. Han, H. Wang, C. Wang, H. Wu, W. Zhou, B. Chen and J. Jiang, J. Am. Chem. Soc., 2019, 141, 8737–8740 CrossRef CAS PubMed;
(f) K. Kosugi, C. Akatsuka, H. Iwami, M. Kondo and S. Masaoka, J. Am. Chem. Soc., 2023, 145, 10451–10457 CrossRef CAS PubMed;
(g) G. Li, X. Lv, W. Ji, Y. Zhou, Z. Lin, H. Cao and T. Tan, J. Mater. Chem. C, 2023, 11, 7411–7418 RSC.
- M. Kimura, M. Yoshida, S. Fujii, A. Miura, K. Ueno, Y. Shigeta, A. Kobayashi and M. Kato, Chem. Commun., 2020, 56, 12989–12992 RSC.
-
CrysAlisPro, Rigaku Corporation, Tokyo, Japan, 2015 Search PubMed.
- G. M. Sheldrick, Acta Crystallogr., Sect. A: Found. Adv., 2015, 71, 3–8 CrossRef PubMed.
- G. M. Sheldrick, Acta Crystallogr., Sect. C: Struct. Chem., 2015, 71, 3–8 Search PubMed.
-
(a) P. van der Sluis and A. L. Spek, Acta Crystallogr., Sect. A: Found. Crystallogr., 1990, 46, 194–201 CrossRef;
(b) A. L. Spek, Acta Crystallogr., Sect. A: Found. Crystallogr., 1990, 46, C34 Search PubMed.
-
(a) W. R. Dawson and M. W. Windsor, J. Phys. Chem., 1968, 72, 3251–3260 CrossRef CAS;
(b) W. H. Melhuish, J. Phys. Chem., 1961, 65, 229–235 CrossRef CAS.
-
J. R. Lakowicz, Principles of Fluorescence Spectroscopy, Springer, New York, 2006, pp. 141–142 Search PubMed.
-
Gaussian 16, Revision C.01, M. J. Frisch, G. W. Trucks, H. B. Schlegel, G. E. Scuseria, M. A. Robb, J. R. Cheeseman, G. Scalmani, V. Barone, G. A. Petersson, H. Nakatsuji, X. Li, M. Caricato, A. V. Marenich, J. Bloino, B. G. Janesko, R. Gomperts, B. Mennucci, H. P. Hratchian, J. V. Ortiz, A. F. Izmaylov, J. L. Sonnenberg, D. Williams-Young, F. Ding, F. Lipparini, F. Egidi, J. Goings, B. Peng, A. Petrone, T. Henderson, D. Ranasinghe, V. G. Zakrzewski, J. Gao, N. Rega, G. Zheng, W. Liang, M. Hada, M. Ehara, K. Toyota, R. Fukuda, J. Hasegawa, M. Ishida, T. Nakajima, Y. Honda, O. Kitao, H. Nakai, T. Vreven, K. Throssell, J. A. Montgomery Jr., J. E. Peralta, F. Ogliaro, M. J. Bearpark, J. J. Heyd, E. N. Brothers, K. N. Kudin, V. N. Staroverov, T. A. Keith, R. Kobayashi, J. Normand, K. Raghavachari, A. P. Rendell, J. C. Burant, S. S. Iyengar, J. Tomasi, M. Cossi, J. M. Millam, M. Klene, C. Adamo, R. Cammi, J. W. Ochterski, R. L. Martin, K. Morokuma, O. Farkas, J. B. Foresman and D. J. Fox, Gaussian, Inc., Wallingford CT, 2019 Search PubMed.
-
(a) C. Lee, W. Yang and R. G. Parr, Phys. Rev. B: Condens. Matter Mater. Phys., 1988, 37, 785–789 CrossRef CAS PubMed;
(b) A. D. Becke, J. Chem. Phys., 1993, 98, 5648–5652 CrossRef CAS;
(c) S. Grimme, S. Ehrlich and L. Goerigk, J. Comput. Chem., 2011, 32, 1456–1465 CrossRef CAS PubMed.
- D. Andrae, U. Häussermann, M. Dolg, H. Stoll and H. Preuss, Theor. Chim. Acta, 1990, 77, 123–141 CrossRef CAS.
- R. Krishnan, J. S. Binkley, R. Seeger and J. A. Pople, J. Chem. Phys., 1980, 72, 650–654 CrossRef CAS.
- A. V. Marenich, C. J. Cramer and D. G. Truhlar, J. Phys. Chem. B, 2009, 113, 6378–6396 CrossRef CAS PubMed.
- S. F. Boys and F. Bernardi, Mol. Phys., 1970, 19, 553–566 CrossRef CAS.
-
(a) Y. Wang, X. Deng, Y. Liu, M. Ni, M. Liu, H. Tan, X. Li, W. Zhu and Y. Cao, Tetrahedron, 2011, 67, 2118–2124 CrossRef CAS;
(b) J. Yu, K. He, Y. Li, H. Tan, M. Zhu, Y. Wang, Y. Liu, W. Zhu and H. Wu, Dyes Pigm., 2014, 107, 146–152 CrossRef CAS;
(c) M. Ebina, A. Kobayashi, T. Ogawa, M. Yoshida and M. Kato, Inorg. Chem., 2015, 54, 8878–8880 CrossRef CAS PubMed;
(d) K. Ohno, M. Hasebe, A. Nagasawa and T. Fujihara, Inorg. Chem., 2017, 56, 12158–12168 CrossRef CAS PubMed;
(e) J. Ni, W.-J. Qi, S.-Q. Liu and J.-J. Zhang, Dyes Pigm., 2022, 205, 110567 CrossRef CAS;
(f) D. Gómez de Segura, E. Lalinde and M. T. Moreno, Inorg. Chem., 2022, 61, 20043–20056 CrossRef PubMed;
(g) Y. Makino, M. Yoshida, S. Hayashi, T. Sasaki, S. Takamizawa, A. Kobayashi and M. Kato, Dalton Trans., 2023, 52, 8864–8872 RSC.
- A. Bondi, J. Phys. Chem., 1964, 68, 441–451 CrossRef CAS.
- K. S. W. Sing, D. H. Everett, R. A. W. Haul, L. Moscou, R. A. Pierotti, J. Rouquérol and T. Siemieniewska, Pure Appl. Chem., 1985, 57, 603–619 CrossRef CAS.
-
(a) C. R. Patrick and G. S. Prosser, Nature, 1960, 187, 1021 CrossRef CAS;
(b) J. H. Williams, Acc. Chem. Res., 1993, 26, 593–598 CrossRef CAS;
(c) L. M. Salonen, M. Ellermann and F. Diederich, Angew. Chem., Int. Ed., 2011, 50, 4808–4842 CrossRef CAS PubMed.
-
(a) R. Xu, W. B. Schweizer and H. Frauenrath, J. Am. Chem. Soc., 2008, 130, 11437–11144 CrossRef CAS PubMed;
(b) T. Itoh, M. Kondo, M. Kanaike and S. Masaoka, CrystEngComm, 2013, 15, 6122–6126 RSC;
(c) T. Itoh, M. Kondo, H. Sakamoto, K. Wakabayashi, M. Kanaike, K. Itami and S. Masaoka, Dalton Trans., 2015, 44, 15334–15342 RSC;
(d) H. Zhang, J. Han, X. Jin and P. Duan, Angew. Chem., Int. Ed., 2021, 60, 4575–4580 CrossRef CAS PubMed.
-
(a) A. F. Rausch, U. V. Monkowius, M. Zabel and H. Yersin, Inorg. Chem., 2010, 49, 7818–7825 CrossRef CAS PubMed;
(b) A. Bossi, A. F. Rausch, M. J. Leitl, R. Czerwieniec, M. T. Whited, P. I. Djurovich, H. Yersin and M. E. Thompson, Inorg. Chem., 2013, 52, 12403–12415 CrossRef CAS PubMed;
(c) A. M. Prokhorov, T. Hofbeck, R. Czerwieniec, A. F. Suleymanova, D. N. Kozhevnikov and H. Yersin, J. Am. Chem. Soc., 2014, 136, 9637–9642 CrossRef CAS PubMed;
(d) T. Ogawa, W. M. C. Sameera, D. Saito, M. Yoshida, A. Kobayashi and M. Kato, Inorg. Chem., 2018, 57, 14086–14096 CrossRef CAS PubMed;
(e) C. Wakasugi, M. Yoshida, W. M. C. Sameera, Y. Shigeta, A. Kobayashi and M. Kato, Chem. – Eur. J., 2020, 26, 5449–5458 CrossRef CAS PubMed.
-
(a) C.-W. Hsu, K. T. Ly, W.-K. Lee, C.-C. Wu, L.-C. Wu, J.-J. Lee, T.-C. Lin, S.-H. Liu, P.-T. Chou, G.-H. Lee and Y. Chi, ACS Appl. Mater. Interfaces, 2016, 8, 33888–33898 CrossRef CAS PubMed;
(b) K. T. Ly, R.-W. Chen-Cheng, H.-W. Lin, Y.-J. Shiau, S.-H. Liu, P.-T. Chou, C.-S. Tsao, Y.-C. Huang and Y. Chi, Nat. Photonics, 2017, 11, 63–68 CrossRef;
(c) D. Saito, T. Ogawa, M. Yoshida, J. Takayama, S. Hiura, A. Murayama, A. Kobayashi and M. Kato, Angew. Chem., Int. Ed., 2020, 59, 18723–18730 CrossRef CAS PubMed;
(d) K. Sasaki, D. Saito, M. Yoshida, F. Tanaka, A. Kobayashi, K. Sada and M. Kato, Chem. Commun., 2023, 59, 6745–6748 RSC.
-
(a) W. B. Connick, L. M. Henling, R. E. Marsh and H. B. Gray, Inorg. Chem., 1996, 35, 6261–6265 CrossRef CAS;
(b) G. Y. Zheng and D. P. Rillema, Inorg. Chem., 1998, 37, 1392–1397 CrossRef CAS PubMed;
(c) M. Kato, C. Kosuge, K. Morii, J. S. Ahn, H. Kitagawa, T. Mitani, M. Matsushita, T. Kato, S. Yano and M. Kimura, Inorg. Chem., 1999, 38, 1638–1641 CrossRef CAS;
(d) M. Nakagaki, S. Aono, M. Kato and S. Sakaki, J. Phys. Chem. C, 2020, 124, 10453–10461 CrossRef CAS.
-
(a) M. Kato, H. Ito, M. Hasegawa and K. Ishii, Chem. – Eur. J., 2019, 25, 5105–5112 CrossRef CAS PubMed;
(b) M. Kato, M. Yoshida, Y. Sun and A. Kobayashi, J. Photochem. Photobiol., C, 2022, 51, 100477 CrossRef CAS.
Footnote |
† Electronic supplementary information (ESI) available: Supplementary crystallographic, spectroscopic, and theoretical data. CCDC 2333937–2333939. For ESI and crystallographic data in CIF or other electronic format see DOI: https://doi.org/10.1039/d4dt00713a |
|
This journal is © The Royal Society of Chemistry 2024 |