DOI:
10.1039/D4DT00626G
(Paper)
Dalton Trans., 2024,
53, 8969-8979
Magnetic properties and magnetocaloric effect of Ln = Dy, Tb carborane-based metal–organic frameworks†
Received
1st March 2024
, Accepted 12th April 2024
First published on 16th April 2024
Abstract
We present the synthesis and magneto-thermal properties of carborane-based lanthanide metal–organic frameworks (MOFs) with the formula {[(Ln)3(mCB-L)4(NO3)(DMF)n]·Solv}, where Ln = Dy or Tb, characterized by dc and ac susceptibility, X-ray absorption spectroscopy (XAS), X-ray magnetic circular dichroism (XMCD) and heat capacity measurements. The MOF structure is formed by polymeric 1D chains of Ln ions with three different coordination environments (Ln1, Ln2, Ln3) running along the b-axis, linked by carborane-based linkers thus to provide a 3D structure. Static magnetic measurements reveal that these MOFs behave at low temperature as a system of S* = 1/2 Ising spins, weakly interacting ferromagnetically along the 1D polymeric chain (J*/kB = +0.45 K (+0.5 K) interaction constant estimated for Dy-MOF (Tb-MOF)) and coupled to Ln ions in adjacent chains through dipolar antiferromagnetic interactions. The Dy MOF exhibits slow relaxation of magnetization through a thermally activated process, transitioning to quantum tunneling of the magnetization at low temperatures, while both compounds exhibit field-induced relaxation through a very slow, direct process. The maximum magnetic entropy changes (−ΔSmaxm) for an applied magnetic field change of 2-0 T are 5.71 J kg−1 K−1 and 4.78 J kg−1 K−1, for Dy and Tb MOFs, respectively, while the magnetocaloric effect (MCE) peak for both occurs at T ∼ 1.6 K, approximately double that for the Gd counterpart.
1. Introduction
Metal–organic frameworks (MOFs) have emerged as a transformative class of crystalline materials, owing to their intriguing porous architecture1–3 and application potential in catalysis,4 gas storage5 and separation, magnetism,6–8 sensors,9etc. Chemical design enables the creation of diverse MOF architectures, incorporating various functionalities by carefully selecting the functional nodes, organic linkages, or species inside the pores.
In particular, MOFs coordinating lanthanide ions (Ln-MOFs) exhibit unique optical, electronic, and magnetic properties derived from 4f electrons, making them attractive for applications in luminescence,10,11 anticounterfeiting,12 sensors,13–15 switching,16 thermometry,17 magnetic refrigeration,18–22 conductive MOFs23 and information storage and processing,24,25 among others.
Indeed, trivalent Ln(III) ions favor outstanding luminescence features like a large Stokes shift, high purity of colours and considerable quantum yields, especially in Tb(III) and Eu(III) ions.12,26–28 In addition, the 4f orbitals of Ln(III) ions provide a significant magnetic moment and intrinsic anisotropy. Thus, they are good candidates for the construction of single-molecule magnets (SMMs), showcasing magnetic stability and quantum-tunneling effects.29–31 Recent advances have shown the possibility to produce single-ion magnets (SIMs) with huge thermal activation energies close to 2000 K32 and open hysteresis loops above the liquid nitrogen temperature,33 using Dy(III) in an adequate coordination environment. In parallel, Ln(III) ions are being considered to encode molecular qubits,34 offering attractive advantages for quantum computing, such as the possibility to encode multiple qubits in one sole ion,35,36 and enlarge the quantum basis states by coupling the electronic spin with the nuclear spin.37 The latter approach was for instance used to demonstrate the Grover algorithm in a TbPc2 molecule.38
Therefore, Ln-MOFs represent an attractive possibility both for the preparation of ultra-high-density information storage devices39 and the construction of spatially ordered qubit arrays, or “quMOFs”,40,41 for quantum computing technologies. Lanthanide SIM-MOFs constitute also an ideal benchmark to study dynamic spin magnetic relaxation, depending on the relative importance of the ions’ anisotropy compared to interactions, regulated by the structure topology and linkers.42 Achieving high-energy barriers in MOF systems remains challenging, though, due to the difficulty in preserving strong uniaxial magnetic anisotropy and controlling the easy-axis alignment of the spin carriers throughout the framework. Consequently, compared to the widespread reporting of isolated Ln-SIMs, there exist relatively few Ln-MOFs, predominantly based on Dy(III), exhibiting slow magnetic relaxation properties,14,43–49 and the energy barriers in these systems remain modest (at most 417 K (ref. 16)).
In addition, Ln-MOFs are well suited for crafting materials with the magnetocaloric effect (MCE), for use in ultra-low temperature cooling.18–21 Although most complexes investigated for magnetocalorics contain Gd3+, because its large S = 7/2 and negligible magnetic anisotropy allow achieving the highest maximum entropy changes (typically for temperatures <2 K and applied magnetic fields >5 T),22 some proposals advocate the use of anisotropic lanthanide ions to optimize the MCE performance for lower field changes, achievable by permanent magnets,50 and high temperatures above 4 K.51–53 Additionally, some interesting MOFs for MCE have been reported using [Ln6] clusters (Ln = Gd, Dy),54 or 4f clusters separated by 3d centres.55
All in all, Ln-MOFs offer immense potential for designing multifunctional materials incorporating several properties, such as magnetism combined with MCE, luminescence,26,27,56 proton conductivity,57,58etc.
Despite the enormous interest, Ln-MOFs are relatively scarcer than their transition metal counterparts. This is because typically Ln(III) ions exhibit high coordination numbers with distorted symmetries which bring flexibility to the coordination network, making the prediction of structures more challenging. In order to build stable and efficiently functionalized MOFs, the choice of appropriate organic linkers is a key part of the framework design. Considering the diversity of the coordination modes and the high affinity between the O atom and Ln(III) ions, carboxylates have been the most popular type of spacer employed for the assembly of Ln-MOFs.59–61 Still, the design and synthesis of new Ln-MOFs with alternative linkers is a promising endeavor, as it unlocks heightened tunability, substantially expanding their range of applications.62
In the last few years, we have demonstrated the efficacy of carborane-based linkers to synthesize a variety of functional MOFs.12,63–65,66–73 Icosahedral carboranes are a class of commercially available and exceptionally stable boron-rich clusters,74–76 possessing an unusual electronic structure, which is often highlighted by considering carboranes as inorganic 3D “aromatic” analogs of arenes.74–76 They have high hydrophobicity,77–83 allowing the preparation of water-stable MOFs.12,64,65,67,71
Recently, we reported the successful synthesis and optical properties of {[(Ln)3(mCB-L)4(NO3)(DMF)n]·Solv} (Ln = Eu, Tb, Eu/Tb),12 using the carborane linker mCB-L = 1,7-di(4-carboxyphenyl)-1,7-dicarba-closo-dodecaborane. Moreover, we demonstrated the possibility of synthesizing multi-metallic MOFs incorporating up to eight different sized rare-earth cations,66 leveraging the steric bulkiness and acidity of the mCB-L ligand. This milestone opens the way for the development of multifunctional multi-Ln MOF materials with tailored properties.
Herein, we present the magneto-thermal properties of two homonuclear MOFs of this family, {[(Ln)3(mCB-L)4(NO3)(DMF)n]·Solv}, incorporating Ln = Dy or Tb. These two lanthanides have been selected to compare the magnetic properties of two isostructural MOFs based on a Kramers ion (Dy, 6H15/2, S = 5/2, L = 5, J = 15/2) and a non-Kramers ion (Tb, 7F6, S = 3, L = 3, J = 6). The magnetic and thermal properties have been characterized by combining dc and ac magnetometry, X-ray absorption spectroscopy (XAS), X-ray magnetic circular dichroism (XMCD), and heat capacity techniques.
2. Experimental
Synthesis
All chemicals were of reagent-grade quality. They were purchased from commercial sources and used as received. The 1,7-Di(4-carboxyphenyl)-1,7-dicarba-closo-dodecaborane ligand (mCB-H2L) was synthesized according to the literature procedure.84 Synthesis of {[(Ln)3(mCB-L)4(NO3)(DMF)n]·Solv} (mCB-Ln, where Ln = Dy or Tb) was performed as previously described.12,66 In a typical preparation, mCB-L (0.03 mmol) and Ln(NO3)3 (0.02 mmol; Ln = Dy or Tb) were added to a mixture of DMF (0.5 mL)/methanol (1.5 mL)/H2O (0.3 mL) and sonicated until complete dissolution of all reagents. The above mixture was transferred to an 8-dram vial and heated at 95 °C in an oven for 48 h. Needle-like white crystals were collected and washed with DMF.
Basic characterization
Powder X-ray diffraction (PXRD) was recorded at room temperature on a Siemens D-5000 diffractometer with Cu Kα radiation (λ = 1.5418 Å). Thermogravimetric analysis (TGA) was performed in N2, on an nSTA 449 F1 Jupiter instrument (heating rate: 10 °C min−1; temperature range: 25 to 800 °C).
Magnetometry
The magnetization and the dc and ac susceptibility of powdered samples were measured, above 1.8 K, using a Quantum Design superconducting quantum interference device (SQUID) magnetometer. Ac measurements were performed at an excitation field of 4 Oe, at temperatures between 1.8 K and 8.0 K, under dc fields between 0 and 3 T, while sweeping the frequency between 0.1 and 1000 Hz. Additional ac susceptibility measurements in the 10–10
000 Hz range were carried out in a Quantum Design PPMS. Measurements on powdered samples were performed with the addition of Daphne oil, introduced to fix the grains at low temperatures.
XAS and XMCD
X-ray absorption spectroscopy (XAS) and X-ray magnetic circular dichroism (XMCD) experiments across the M4,5 edges of Dy and Tb were performed at BOREAS beamline in ALBA synchrotron. The powdered samples were crushed on an indium foil and placed at the tip of a cold finger. Measurements were performed at 3.4 K ± 0.5 K, in total electron yield (TEY) detection mode, with a 90% circularly polarized light. The XMCD (μ− − μ+) and XAS (μ+ + μ−/2) spectra at 6 T were determined from eight X-ray absorption spectra measured under right-handed (μ+) and left-handed (μ−) circular polarizations. XMCD(H) cycles were performed by following the resonant M5 peak while sweeping the magnetic field between 6 T and −6 T at a rate of 2 T min−1.
Heat capacity
Heat capacity as a function of temperature was measured between 0.3 and 100 K at different applied fields between 0 and 3 T on a pressed powder pellet fixed with Apiezon N grease, using a Quantum Design PPMS equipped with a 3He refrigerator.
3. Results
Structural characterization
The single-crystal structure of {[(Tb)3(mCB-L)4(NO3)(DMF)n]·Solv} (mCB-Tb) was previously determined by single-crystal X-ray diffraction (SCXRD).12 The secondary building unit (SBU) of this MOF is composed of three non-equivalent crystallographic terbium atoms, Tb(1) and Tb(3) being eight-coordinated, while Tb(2) is seven-coordinated (Fig. 1a). The varied coordination around the three crystallographic independent Ln atoms results in three different Tb–Tb metal distances (Tb1–Tb2 4.5830(8), Tb2–Tb3 5.2550(7) and Tb1–Tb3 4.6398(7) Å). The Tb–O bond distances are in the range 2.272(10)–2.906(10) Å. These Tb cations are capped by bridging, chelate bridging or by chelating mCB-L, and chelating NO3− and DMF molecules forming 1D chains running along the b-axis, that are further connected by the ditopic mCB-L ligand and thus provide the observed 3D structure (Fig. 1b and c). Two Tb inter-chain distances can be found in the structure (11.0 and 17.4 Å; see Fig. 1b and c). Powder XRD measurements reveal that mCB-Dy is isostructural to mCB-Tb (Fig. S1†) and confirm that these single-metal mCB-Ln materials display the same crystal structure.
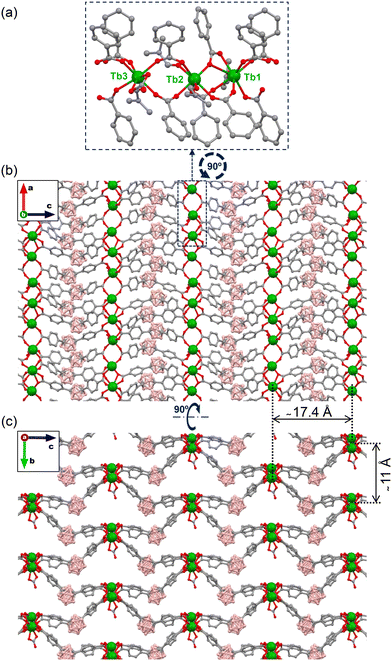 |
| Fig. 1 Structure of {[(Tb)3(mCB-L)4(NO3)(DMF)n]·Solv} (mCB-Tb). (a) View of the coordination of mCB-L to the three independent Tb atoms that are repeated along the structure. (b and c) Two perpendicular views of the extended 3D framework showing the 1D Tb chains. Distances between the polymeric 1D Tb chains are indicated. Colour code: Tb (green), O (red), B (pink), C (grey), and N (blue); H atoms (a–c) and DMF molecules (b–c) are omitted for clarity. The figure was prepared using Mercury.97 | |
1D channels can be observed along the same direction as the 1D-Tb chains that can accommodate guest molecules (Fig. 1c). Thermogravimetric analysis indicates that both materials undergo loss of the solvent (coordinated to metal sites and/or distributed in the pores) in the range 200–350 °C and decomposition of the framework above 450 °C (Fig. S2†). Following thermal activation (elimination of solvent in the voids through high vacuum thermal treatment), sorption measurements indicated that mCB-Dy and mCB-Tb compounds are non-porous to N2 but porous to CO2, with Dubini–Radushkevich surface areas of 196 and 331 m2 g−1, respectively (Fig. S3 and S4†). The smaller surface area of mCB-Dy than that of mCB-Tb can be explained by the lanthanide contraction effect, which predicts a shrinkage of pore size as the atomic number of the lanthanide increases for an isostructural family of MOFs.85
Static magnetic results
To characterize the static magnetic behavior of the Dy and Tb carborane-based MOF, we measured the temperature dependence of the susceptibility times the temperature, χT(T), at 0.1 T (Fig. 2a and c) and the field dependence of the magnetization M(H) at different temperatures between T = 1.8 K and T = 21 K (Fig. 2b and d). For each compound, the 1/χ(T) data in the high-temperature region between 150 and 300 K were fit to a Curie–Weiss law, 1/χ = C/(T − θ), to obtain the Curie constant (C) and the Curie–Weiss temperature (θ); see Fig. 2a–c, insets.
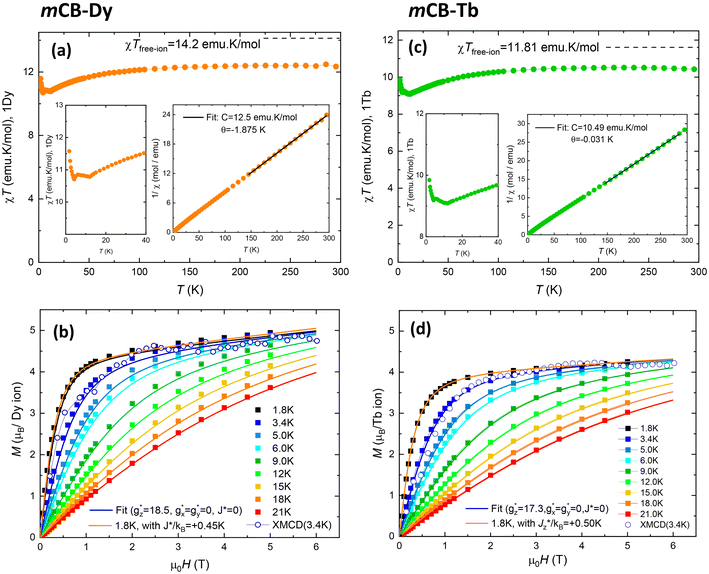 |
| Fig. 2 Static magnetic properties for mCB-Dy (left) mCB-Tb (right). (a and c) Temperature dependence of the susceptibility times the temperature, χT(T), at 0.1 T. Inset: Zoom of the low-T region. Additionally, inverse of the susceptibility, 1/χ and fit to a Curie–Weiss law in the T > 150 K region; (b and d) bold symbols: field dependence of the magnetization per Ln ion, M(H), measured at different temperatures between T = 1.8 K and 21 K; open circles: mtot(H)/ion curve obtained at 3.4 K from XMCD(H). Lines: theoretical M(H) curves calculated with Magpack within an Ising model of ions with an effective spin S* = 1/2 and gyromagnetic values and , and negligible interactions (J = 0). The fit of the M(H) at 1.8 K within a dimer model with a small, ferromagnetic constant J*/kB = +0.45 K (+0.50 K) for mCB-Dy (mCB-Tb) is also shown. | |
For mCB-Dy, the χT(T) product curve per Dy ion is shown in Fig. 2a. The room temperature value of 12.47 emu K mol−1 is somewhat below the χTfree ion = gJ2J(J + 1)/8 = 14.2 emu K mol−1 expected for Dy3+(6H15/2, gJ = 4/3). Upon decreasing the temperature, χT decreases smoothly until 10.7 emu K mol−1 at 4.2 K, and then increases, reaching 11.4 emu K mol−1 at 1.8 K. (It is noted that the small bulge appearing around 7 K is an instrumental artifact produced by the change of measurement regime mode.) This increase points to the existence of ferromagnetic interactions between the intra-chain Dy–Dy ions. On the other hand, the Curie–Weiss fit of the 1/χ data (Fig. 2a, inset) yields a negative θ = −1.87 K, evidencing the existence of overall antiferromagnetic (AF) interactions. Given that intra-chain short-range interactions are FM, this result suggests the existence of additional antiferromagnetic inter-chain Dy–Dy interactions, possibly of dipolar origin.
The isothermal magnetization curves measured for the mCB-Dy compound down to 1.8 K are shown in Fig. 2b. The M(H) can be reasonably well fit under a model of non-interacting Dy ions with effective spin S* = 1/2 and the anisotropic ground state with gyromagnetic factors
and
, with a small van Vleck contribution (MVV = H × 0.12μB/T).86 Since XRD measurements evidence that there are three different Dy sites (Dy1, Dy2, Dy3), in this model
can be considered as an average of the anisotropic gyromagnetic factors of the three. At low T, the data fall slightly above the theoretical curves calculated for non-interacting ions (J* = 0). A reasonable fit of M(H) curve at 1.8 K is achieved assuming a simple model of dimer Dy–Dy ions interacting through a coupling constant of J*/kB = +0.45 K, thereby confirming the existence of weak, ferromagnetic (FM) intrachain as anticipated from χT(T) results.
Qualitatively, the static magnetic properties of mCB-Tb are similar to those of the Dy counterpart. The χT(T) curve per Tb (Fig. 2c) shows a room-temperature value of 10.47 emu K mol−1, somewhat below the χTfree ion = 11.8 emu K mol−1 expectation for Tb3+(Tb3+, 7F6, gJ = 3/2). By lowering the temperature, the χT decreases until reaching 9.1 emu K mol−1 (10 K), and then increases to 9.8 emu K mol−1 at 1.8 K, again pointing to the presence of FM intra-chain Tb–Tb couplings. The Curie–Weiss fitting of 1/χ data (Fig. 2c, inset) yields a negative, close to zero value of θ = −0.03 K (smaller than for mCB-Dy), suggesting near zero AF inter-chain interactions in the case of mCB-Tb. On the other hand, the isothermal M(H) curves measured between 3.4 and 21 K (Fig. 2d) are well reproduced under a model of S* = 1/2 spins with average, anisotropic gyromagnetic factors
,
, a van Vleck contribution of MVV = H × 0.058μB/T and negligible interactions (J* = 0), while the 1.8 K is better fit including a small, ferromagnetic interaction of J*/kB = +0.50 K (see details in S2†).
XAS and XMCD characterization
X-ray absorption spectroscopy (XAS) and X-ray magnetic circular dichroism (XMCD) measurements were performed to further characterize the spectral characteristics across the M4,5 edges of Dy in mCB-Dy and Tb in mCB-Tb, and obtain the orbital moment (mL), spin moment (ms) and total magnetic moment (mtot = mL + ms) of each ion through application of the sum rules87,88 adapted for lanthanides, following the procedure described by S. Tripathi.89 The correction is needed to account for the large jj mixing between the 3d5/2 and 3d3/2 core levels, on the one hand, and the contribution of the 〈Tz〉 magnetic dipole term, on the other hand (see details in S3†).
Fig. 3 (left) shows the XAS and XMCD spectra measured across the M4,5 edge of Dy in mCB-Dy at 3.4 K and 6 T, which show the typical spectral features for trivalent dysprosium. The XAS spectrum displays two pre-peaks (1290.81, 1292.79 eV), a main peak (1294.6) at M5 and a shoulder (1297.3 eV), while M4 includes two unresolved peaks (1328.1 and 1328.8 eV). The XMCD spectrum consists of negative peaks, across M5 a main peak at 1294.6 eV, a minor peak at 1296.9 eV, and a small peak at M4 (1327.8 eV). Application of the sum rules adapted for Dy(III) with a number of holes nh = 5 yields mL = 2.53μB/ion, ms = 2.34μB/ion, and mtot = 4.87μB/ion at 3.4 K and 6 T.
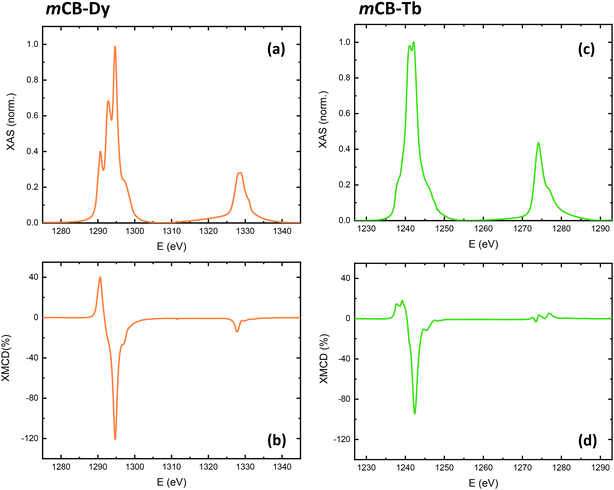 |
| Fig. 3 XAS and XMCD characterization of mCB-Dy (left) and mCB-Tb (right). Normalized, background-subtracted XAS (top) and XMCD (bottom) spectra measured across the M4,5 edge of Dy (a and b) and Dy (c and d) at 6 T at T = 3.4 K. | |
The XAS and XMCD spectra across the M4,5 edge of Tb in mCB-Tb at 3.4 K and 6 T (Fig. 3, right) show the expected features for terbium(III). The XAS spectrum exhibits a pre-peak (1235.19 eV) and two main peaks (1241, 1242.1 eV) and shoulder in the M5 region, while M4 consists of a peak at 1270.53 eV and shoulder (1273.2 eV). The XMCD spectrum contains a primary negative peak at 1242.31 eV in the M5 region and a minor peak at 1245.3 eV, and three features in the M4 region (1273.4–1276.7 eV). The sum rules applied to Tb(III), with nh = 6, yield in this case mL = 1.47μB/ion, ms = 2.89μB/ion and mtot = 4.36μB/ion, at 3.4 K and 6 T. Thus, the determined orbital magnetic moment for Dy is larger than for Tb in these mCB-Ln MOFs.
In addition, the field dependence of the total magnetic moment, mtot(H)/ion, of Dy in mCB-Dy and Tb in mCB-Tb at 3.4 K was determined by following the intensity of the XMCD(H) peak at the M5 edge between −6 T and 6 T, and scaling the curve with the mtot(6 T) value obtained from the sum rules. As shown in Fig. 2b and d (open circles), the mtot(H)/ion curves determined by XMCD are in good agreement with the M(H) curves measured by SQUID at 3.4 K, within the experimental noise of the technique.
Heat capacity
The temperature dependence of the specific heat, cp(T), measured down to 0.3 K at different applied magnetic fields ranging between 0 and 3 T for the two studied compounds is shown in Fig. 4 (top). In both cases, the magnetic heat capacity contribution peaks, cm(T, H), are observed over the lattice contribution cL(T).
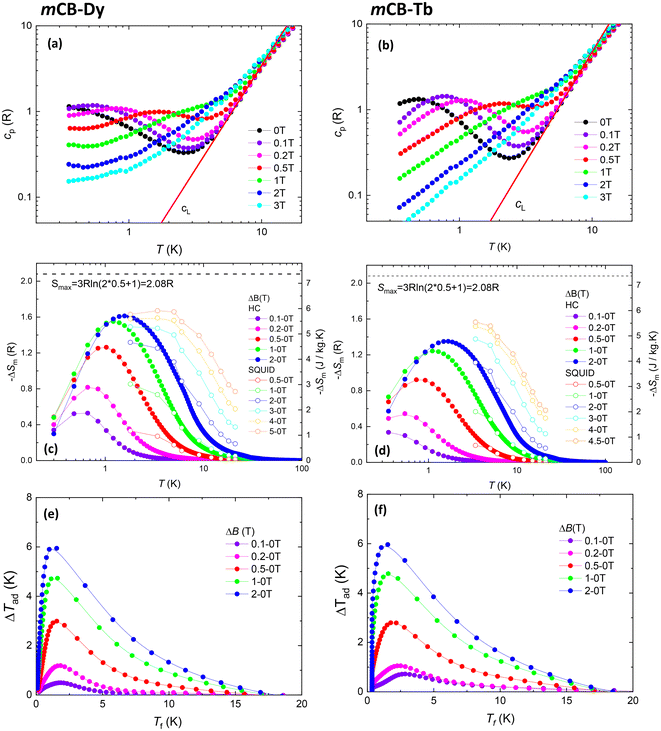 |
| Fig. 4 Heat capacity and MCE characterization for mCB-Dy (left) mCB-Tb (right). (a, b) Specific heat as a function of the temperature, cp(T), at different applied magnetic fields. The line corresponds to the lattice contribution, cL(T) = ATn, (A/R = 0.01265 K−2.57, n = 2.57 for mCB-Dy; A/R = 0.0128 K−2.43, n = 2.43 for mCB-Tb); (c, d) temperature dependence of the magnetic entropy change, −ΔSm, obtained from the cm(T) = cp(T) − cL(T) data for the indicated magnetic field changes ΔB. The dashed line represents the maximum full entropy content of the Dy and Tb MOFs, Smax = 3R ln(2S + 1), containing three Ln3+ ions with S* = 1/2 at low T; (e, f) temperature dependence of the adiabatic temperature change ΔTad(T) for the indicated field changes ΔB. | |
For mCB-Dy, the cm(T) curve at H = 0 shows a Schottky-type broad anomaly with a center lower than 0.3 K. Upon increasing the applied field, the cm(T) peak shifts to higher temperatures, and an increase of the cm signal at the lowest temperature is hinted (Fig. 5a).
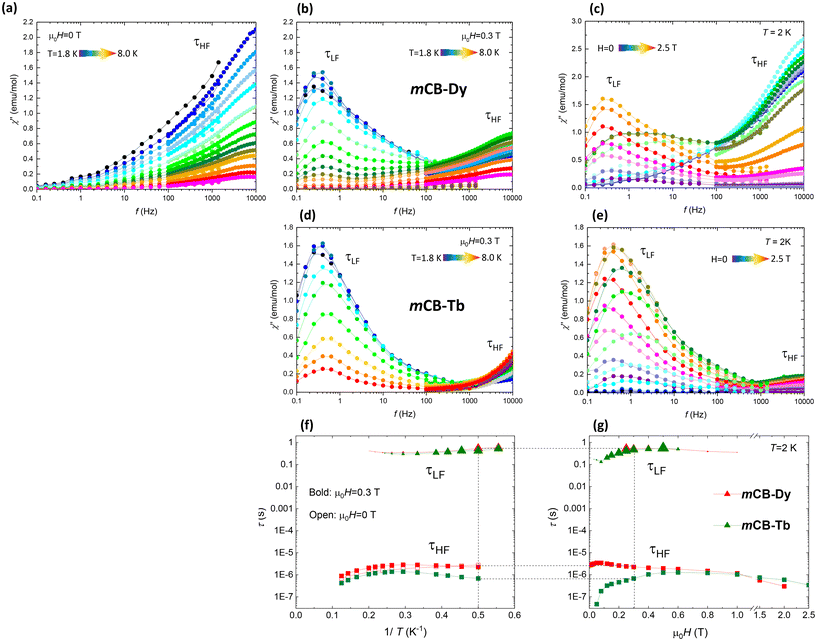 |
| Fig. 5 Ac susceptibility results for the mCB-Dy and mCB-Tb MOFs: (a) χ′′(f, T) at H = 0; (b and d) χ′′(f, T) at μ0H = 3 T; (c), (e) χ′′(f, H) at constant T = 2 K; (f) relaxation time vs. inverse of the temperature, and (g) relaxation time as a function of the applied field, at T = 2.0 K. The relaxation times for the two observed processes are denoted as τLF and τHF. | |
On the other hand, the cm(T, 0) curve of mCB-Tb also shows a Schottky-type anomaly, which is centered at a higher temperature (Tsc = 0.5 K). With increasing field, this peak also shifts to a higher T, but unlike in the former compound, no further contribution at low temperatures is observed (Fig. 5b). No magnetic ordering is observed in either of the two MOFs down to the lowest measured temperature of 0.4 K.
The heat capacity data allowed us to characterize the magnetocaloric effect (MCE). The magnetic entropy change −ΔSm(T, ΔB) for selected magnetic field changes ΔB = μ0(Hf − Hi) is shown in Fig. 4c and d for mCB-Dy and mCB-Tb, respectively. The maximum final field μ0Hf = 2 T is chosen to compare with other values in the literature.50,51,90 This field is taken as the standard, since it is reachable with permanent magnets in the potential application as a magnetic refrigerator. For ΔB = 2-0 T, we find a maximum value of −ΔSmaxm = 5.71 J kg−1 K−1 at Tmax = 1.61 K for mCB-Dy and −ΔSmaxm = 4.78 J kg−1 K−1 at Tmax = 1.67 K for mCB-Tb. Considering the full width at half maximum of the −ΔSm curve, δTFWHM, the obtained relative cooling power is RCP = −ΔSmaxm × δTFWHM = 32.6 J kg−1 K−1 (mCB-Dy) and 27.9 J kg−1 K−1 (mCB-Tb). Besides, the adiabatic temperature change ΔTad(T) = Tf − Ti has been calculated and is shown in Fig. 4e and f. For ΔB = 2–0 T the temperature change reaches a maximum ΔTmaxad = 5.94 K at T = 1.22 K (mCB-Dy) and ΔTmaxad = 5.87 K at T = 1.36 K (mCB-Tb).
Alternatively, the magnetic entropy change was extracted from the M(H) isotherms recorded at different temperatures, using Maxwell's equations.91 This method allowed us to characterize the MCE for a higher ΔB of 5–0 T. The maximum magnetic entropy change obtained was −ΔSm(5 T) = 5.92 J K−1 kg−1 (mCB-Dy) and 5.55 J K−1 kg−1 (mCB-Tb). The magnetic entropy change values for these compounds are smaller than that previously obtained for the gadolinium (S = 7/2) analogue of the same family, mCB-Gd, which exhibited a value of −ΔSmaxm = 16.23 J kg−1 K−1 at T = 0.8 K for ΔB = 2 T.92 However, interestingly, the MCE peak for the Dy and Tb MOFs occurs at a higher temperature than for Gd, consistent with previous findings in the literature,51–53 thus extending the operating temperature for magnetic cooling. Moreover, we emphasize that understanding the magnetocaloric performance of these homonuclear mCB-Ln (Ln = Dy, Tb) compounds is crucial, in view of the subsequent design of multifunctional mixed {GdLn} MOFs.92
Dynamic results
Alternating current (ac) magnetic susceptibility measurements as a function of frequency (f = 0.1–104 Hz) at different temperatures between 1.8 and 8 K and applied magnetic fields from 0 to 2.5 T were performed to characterize spin dynamic relaxation of the studied carborane-based MOFs.
For mCB-Dy, a frequency-dependent out-of-phase susceptibility χ′′(f, T) between 1.8 and 18 K was observed even at zero applied field (Fig. 5a). The χ′′(f, H) measurements performed at constant T = 2 K show the evolution of the processes under magnetic fields ranging from 0 to 2.5 T (Fig. 5c). Under the application of a constant optimum field of 0.3 T (Fig. 5b), the χ′′ curves show a clear peak at low frequencies (LF) at ca. 0.3 Hz, a shoulder at 0.5 Hz, and the tail of a high frequency (HF) peak. In the case of the mCB-Tb compound, no out-of-phase signal was detected at H = 0. Under the application of a magnetic field, the χ′′(f, T, H) curves deployed a large peak at a low frequency, and the emergence of a second relaxation process for f > 10 kHz (Fig. 5d and e).
The relaxation time of the low-frequency processes as a function of the inverse of the temperature and the magnetic field τLF(1/T, H) were determined from the maximum of the χ′′(f) peaks, at which ωτ = 1 (ω = 2πf). For the high-frequency process, for which only the tails of the χ′′ peak were visible within the measurement frequency window, the relaxation time was estimated as τHF = (1/2πf) (χ′′/χ′), at a constant f fulfilling the condition ωτ ≪ 1. The relaxation times of the different processes as a function of the inverse of the temperature and the field, τLF(1/T, H) and τHF(1/T, H), determined for the two compounds are depicted in Fig. 5f and g.
The HF process observed in the mCB-Dy compound with a τHF ≈ 5 × 10−5 s at low temperatures is associated with a fast mechanism of relaxation through quantum tunneling of the magnetization (QTM). At increasing temperatures, the τHF(1/T) exhibits a decreasing trend, indicating that this mechanism is being replaced by a thermally activated mechanism, τ = τ0
exp(Ueff/kBT); however, the data available do not afford obtaining the activation energy (Ueff) reliably, neither at H = 0 nor at H = 0.3 T.
The fact that slow relaxation of magnetization under zero applied field only appears in mCB-Dy and not in mCB-Tb may be attributed to the heightened anisotropy of Ln ions in the former, as suggested by the larger
value obtained from M(H) fits, and supported by the larger orbital magnetic moment mL, as determined by XMCD.
On the other hand, the temperature and field dependencies of the low-frequency processes observed in the two compounds are characteristic of a direct, bottlenecked slow relaxation mechanism. This type of relaxation process has been frequently documented in previous studies of coordination polymers.93–96
4. Conclusions
The magnetic and MCE properties of two isostructural MOFs, {[(Ln)3(mCB-L)4(NO3)(DMF)n]·Solv} (Ln = Dy, Tb) were characterized by combining dc magnetometry, ac magnetometry, XAS–XMCD and heat capacity measurements. The porous 3D structure of these MOFs is constructed by polymeric 1D chains of Ln ions in three different coordination environments (Ln1, Ln2, Ln3) within the SBU, interconnected to adjacent chains through bulky carborane linkers. Static magnetic results revealed that these MOFs can be modeled at low temperature as a system of S* = 1/2 Ising spins weakly coupled ferromagnetically along the chain and simultaneously coupled to Ln ions in neighbouring chains through weak antiferromagnetic dipolar interactions. XAS and XMCD measurements were used to spectroscopically characterize the Ln(III) ions in the two MOFs and determine the average spin, orbital and total magnetic moments. The obtained mL values, together with the gyromagnetic values obtained from the theoretical fitting of the low-temperature M(H) curves, evidence the larger anisotropic character of the Dy ion compared to Tb in these mCB-based MOFs. As expected in this scenario, thermally activated SMM behavior under zero dc field is only observed for the Dy complex, while relaxation through very slow, direct processes under application of a 0.3 T field is observed in both MOFs. Direct MCE characterization through heat capacity measurements revealed a maximum −ΔSmaxm of 5.71 J kg−1 K−1 at 1.61 K for mCB-Dy and 4.78 J kg−1 K−1 at 1.67 K for mCB-Tb, for a field change of 2–0 T. While these magnetic entropy changes are modest compared to the value achieved by the Gd analogue, the MCE peak occurs at a higher temperature, underscoring the potential of using anisotropic ions, alone or mixed with Gd, to design magnetocaloric materials for different cryogenic temperature regions. The two reported carborane-based MOFs emerge as interesting multifunctional materials, combining MCE and magnetic relaxation and high quantum yield luminescent emission in the visible light region for the Tb analogue.
Conflicts of interest
There are no conflicts of interest to declare.
Acknowledgements
This work was financially supported by the Spanish Ministerio de Ciencia e Innovación projects PID2022-138492NB-I00 and PID2022-136892NB-I00, the Generalitat de Catalunya (2021/SGR/00442), and the Gobierno de Aragón (project RASMIA E12-23R). Zhen Li acknowledges the China Scholarship Council (CSC) for his PhD grant (201808310071). The authors would like to acknowledge the use of Servicio General de Apoyo a la Investigación-SAI, Universidad de Zaragoza. XAS and XMCD experiments were performed at the BOREAS beamline of the ALBA synchrotron with the support of ALBA staff (experiment number 2022085925).
References
- H. Li, M. Eddaoudi, M. O'Keeffe and O. M. Yaghi, Nature, 1999, 402, 276–279 CrossRef CAS.
- O. M. Yaghi, M. O'Keeffe, N. W. Ockwig, H. K. Chae, M. Eddaoudi and J. Kim, Nature, 2003, 423, 705–714 CrossRef CAS PubMed.
- O. K. Farha, A. O. Yazaydın, I. Eryazici, C. D. Malliakas, B. D. Hauser, M. G. Kanatzidis, S. T. Nguyen, R. Q. Snurr and J. T. Hupp, Nat. Chem., 2010, 2, 944–948 CrossRef CAS PubMed.
- X. J. Wang, X. Zhang, R. Pandharkar, J. F. Lyu, D. Ray, Y. Yang, S. Kato, J. Liu, M. C. Wasson, T. Islamoglu, Z. Li, J. T. Hupp, L. Gagliardi and O. K. Farha, ACS Catal., 2020, 10, 8995–9005 CrossRef CAS.
- D. Q. Yuan, D. Zhao, D. F. Sun and H. C. Zhou, Angew. Chem., Int. Ed., 2010, 49, 5357–5361 CrossRef CAS PubMed.
- X. Zhang, V. Vieru, X. Feng, J.-L. Liu, Z. Zhang, B. Na, W. Shi, B.-W. Wang, A. K. Powell, L. F. Chibotaru, S. Gao, P. Cheng and J. R. Long, Angew. Chem., Int. Ed., 2015, 54, 9861–9865 CrossRef CAS PubMed.
- K. Liu, X. Zhang, X. Meng, W. Shi, P. Cheng and A. K. Powell, Chem. Soc. Rev., 2016, 45, 2423–2439 RSC.
- G. Mínguez Espallargas and E. Coronado, Chem. Soc. Rev., 2018, 47, 533–557 RSC.
- Y. Zhang, S. Yuan, G. Day, X. Wang, X. Yang and H.-C. Zhou, Coord. Chem. Rev., 2018, 354, 28–45 CrossRef CAS.
- Y. Cui, B. Chen and G. Qian, Coord. Chem. Rev., 2014, 273–274, 76–86 CrossRef CAS.
- Z. Qu, D. Wu, J. Jin, G. Yang and Y.-Y. Wang, J. Solid State Chem., 2022, 309, 123003 CrossRef CAS.
- Z. Li, R. Núñez, M. E. Light, E. Ruiz, F. Teixidor, C. Viñas, D. Ruiz-Molina, C. Roscini and J. G. Planas, Chem. Mater., 2022, 34, 4795–4808 CrossRef CAS PubMed.
- Y. Guo, Z. Han, H. Min, Z. Chen, T. Sun, L. Wang, W. Shi and P. Cheng, Inorg. Chem., 2021, 60, 9192–9198 CrossRef CAS PubMed.
- J.-M. Li, R. Huo, X. Li and H.-L. Sun, Inorg. Chem., 2019, 58, 9855–9865 CrossRef CAS PubMed.
- Q. Lin, W. Xie, Z. Zong, Z. Liu, Y. Sun and L. Liang, New J. Chem., 2021, 45, 7382–7389 RSC.
- S. Mohapatra, B. Rajeswaran, A. Chakraborty, A. Sundaresan and T. K. Maji, Chem. Mater., 2013, 25, 1673–1679 CrossRef CAS.
- D. B. Kanzariya, M. Y. Chaudharya and T. K. Pal, Dalton Trans., 2023, 52, 7383–7404 RSC.
- G. Lorusso, J. W. Sharples, E. Palacios, O. Roubeau, E. K. Brechin, R. Sessoli, A. Rossin, F. Tuna, E. J. L. McInnes, D. Collison and M. Evangelisti, Adv. Mater., 2013, 25, 4653–4656 CrossRef CAS PubMed.
- G. Lorusso, E. Natividad, M. Evangelisti and O. Roubeau, Mater. Horiz., 2019, 6, 144–156 RSC.
- M. Falsaperna and P. Saines, Dalton Trans., 2022, 51, 3394–3410 RSC.
- P. Konieczny, W. Sas, D. Czernia, A. Pacanowska, M. Fitta and R. Pełka, Dalton Trans., 2022, 51, 12762–12780 RSC.
- S. Kumar, G. Gabarró-Riera, A. Arauzo, J. Hrubý, S. Hill, L. Bogani, J. Rubio-Zuazo, J. Jover, E. Bartolomé and E. C. Sañudo, J. Mater. Chem. A, 2024, 12, 6269–6279 RSC.
- C.-L. Chen, C. Wang, X.-Y. Zheng, R. Zhang, Y. Xu, G.-L. Zhuang, L.-S. Long, L.-S. Zheng, X.-J. Kong and Y. Cao, J. Am. Chem. Soc., 2023, 145, 16983–16987 CrossRef CAS PubMed.
- E. Coronado, Nat. Rev. Mater., 2020, 5, 87–104 CrossRef.
- M. J. Graham, J. M. Zadrozny, M. S. Fataftah and D. E. Freedman, Chem. Mater., 2017, 29, 1885–1897 CrossRef CAS.
- E. Bartolomé, A. Arauzo, S. Fuertes, L. Navarro-Spreafica, P. Sevilla, H. F. Cortés, N. Settineri, S. J. Teat and E. C. Sañudo, Dalton Trans., 2023, 52, 7258–7270 RSC.
- E. Bartolomé, A. Arauzo, S. Herce, A. Palau, N. Mestres, S. Fuertes, P. Sevilla, N. S. Settineri, L. Navarro-Spreafico, J. González and E. C. Sañudo, Molecules, 2021, 26, 5503 CrossRef PubMed.
- A. Arauzo, L. Gasque, S. Fuertes, C. Tenorio, S. Bernès and E. Bartolomé, Dalton Trans., 2020, 49, 13671–13684 RSC.
- N. Ishikawa, M. Sugita, T. Ishikawa, S. Y. Koshihara and Y. Kaizu, J. Am. Chem. Soc., 2003, 125, 8694–8695 CrossRef CAS PubMed.
-
E. Bartolomé, A. Arauzo, J. Luzón, J. Bartolomé and F. Bartolomé, in Handbook of Magnetic Materials, ed. E. Brück, Elsevier, 2017, pp. 1–289 Search PubMed.
-
L. Sorace and D. Gatteschi, in Lanthanides and Actinides in Molecular Magnetism, ed. R. Layfield and M. Murugesu, Wiley-VCH, 2015 Search PubMed.
- Z. Zhu, C. Zhao, T. Feng, X. Liu, X. Ying, X.-L. Li, Y.-Q. Zhang and J. Tang, J. Am. Chem. Soc., 2021, 143, 10077–10082 CrossRef CAS PubMed.
- F. S. Guo, B. M. Day, Y. C. Chen, M. L. Tong, A. Mansikkamäki and R. A. Layfield, Science, 2018, 362, 1400–1403 CrossRef CAS PubMed.
- A. Gaita-Ariño, F. Luis, S. Hill and E. Coronado, Nat. Chem., 2019, 11, 301–309 CrossRef PubMed.
- M. D. Jenkins, Y. Duan, B. Diosdado, J. J. García-Ripoll, A. Gaita-Ariño, C. Giménez-Saiz, P. J. Alonso, E. Coronado and F. Luis, Phys. Rev.
B, 2017, 95, 064423 CrossRef.
- F. Luis, P. J. Alonso, O. Roubeau, V. Velasco, D. Zueco, D. Aguilà, J. I. Martínez, L. A. Barrios and G. Aromí, Commun. Chem., 2020, 3, 176 CrossRef CAS PubMed.
- M. Urdampilleta, S. Klyatskaya, M. Ruben and W. Wernsdorfer, Phys. Rev. B: Condens. Matter Mater. Phys., 2013, 87, 195412 CrossRef.
- C. Godfrin, A. Ferhat, R. Ballou, S. Klyatskaya, M. Ruben, W. Wernsdorfer and F. Balestro, Phys. Rev. Lett., 2017, 119, 187702 CrossRef CAS PubMed.
- J. J. Baldovi, E. Coronado and A. Gaita-Arino, Chem. – Eur. J., 2014, 20, 10695–10702 CrossRef CAS PubMed.
- J. López-Cabrelles, L. Escalera-Moreno, Z. Hu, H. Prima-García, G. Mínguez-Espallargas, A. Gaita-Ariño and E. Coronado, Inorg. Chem., 2021, 60, 8575–8580 CrossRef PubMed.
- A. Urtizberea, E. Natividad, P. J. Alonso, M. A. Andrés, I. Gascón, M. Goldmann and O. Roubeau, Adv. Funct. Mater., 2018, 28, 1801695 CrossRef.
- F. Ma, R. Sun, A.-H. Sun, J. Xiong, H.-L. Sun and S. Gao, Inorg. Chem. Front., 2020, 7, 930–938 RSC.
- C. Zhang, X. Ma, P. Cen, X. Jin, J. Yang, A. Y.-Q. Zhang, J. Ferrando-Soria, E. Pardo and X. Liu, Dalton Trans., 2020, 49, 14123–14132 RSC.
- L. Li, Y. Fang, S. Liu, M. Hu and W. Wang, J. Rare Earths, 2023, 41, 100–107 CrossRef CAS.
- C. Bai, C.-T. Li, H.-M. Hu, B. Liu, J.-D. Li and G. Xue, Dalton Trans., 2019, 48, 814–817 RSC.
- Z. Chen, B. Zhao, P. Cheng, X. Q. Zhao, W. Shi and Y. Song, Inorg. Chem., 2009, 48, 3493–3495 CrossRef CAS PubMed.
- T. Yang, S. Wang, C. Lin, X. Wang, B. Zhu and D. Wu, Dalton Trans., 2021, 50, 1293–1299 RSC.
- A. Calahorro, I. Oyarzabal, B. Fernandez, J. Seco, T. Tian and D. Fairen-Jimenez, Dalton Trans., 2016, 45, 591–598 RSC.
- R. Gao, F. Guo, N. Bai, Y. Wu, F. Yang and J. Liang, Inorg. Chem., 2016, 55, 11323–11330 CrossRef CAS PubMed.
- R. J. C. Dixey and P. J. Saines, Inorg. Chem., 2018, 57, 12543–12551 CrossRef CAS PubMed.
- P. J. Saines, J. A. M. Paddison, P. M. M. Thygesen and M. G. Tucker, Mater. Horiz., 2015, 2, 528–535 RSC.
- P. W. Doheny, S. J. Cassidy and P. J. Saines, Inorg. Chem., 2022, 61, 4957–4964 CrossRef CAS PubMed.
- S. Kim, R. Muhammad, K. Son and H. Oh, Inorg. Chem., 2023, 62, 2994–2999 CrossRef CAS PubMed.
- Y.-L. Hou, G. Xiong, P.-F. Shi, R.-R. Cheng, J.-Z. Cui and B. Zhao, Chem. Commun., 2013, 49, 6066–6068 RSC.
- H.-C. Hu, X.-M. Kang, C.-S. Cao, P. Cheng and B. Zhao, Chem. Commun., 2015, 51, 10850–10853 RSC.
- J. González, P. Sevilla, G. Gabarró-Riera, J. Jover, J. Echeverría, S. Fuertes, A. Arauzo, E. Bartolomé and E. C. Sañudo, Angew. Chem., Int. Ed., 2021, 60, 12001–12006 CrossRef PubMed.
- F.-G. Chen, W. Xu, J. Chen, H.-P. Xiao, H.-Y. Wang, Z. Chen and J.-Y. Ge, Inorg. Chem., 2022, 61, 5388–5396 CrossRef CAS PubMed.
- X.-Q. Ji, R. Sun, J. Xiong, H.-L. Sun and S. Gao, J. Mater. Chem. C, 2021, 9, 15858–15867 RSC.
- I. Oyarzabal, B. Fernández, J. Cepeda, S. Gómez-Ruiz, A. J. Calahorro, J. M. Seco and A. Rodríguez-Diéguez, CrystEngComm, 2016, 18, 3055–3063 RSC.
- R. Li, R. Li, X. Liu, X. Chang and X. Feng, RSC Adv., 2020, 10, 6192–6199 RSC.
- Q.-Y. Liu, Y.-L. Li, Y.-L. Wang, C.-M. Liu, L.-W. Ding and Y. Liu, CrystEngComm, 2014, 16, 486–491 RSC.
- S. Wang, T. Cao, H. Yan, Y. Li, J. Lu, R. Ma, D. Li, J. Dou and J. Bai, Inorg. Chem., 2016, 55, 5139–5151 CrossRef CAS PubMed.
- L. Gan, E. Andres-Garcia, G. Mínguez Espallargas and J. G. Planas, ACS Appl. Mater. Interfaces, 2023, 15, 5309–5316 CrossRef CAS PubMed.
- M. Y. Tsang, S. Rodríguez-Hermida, K. C. Stylianou, F. Tan, D. Negi, F. Teixidor, C. Viñas, D. Choquesillo-Lazarte, C. Verdugo-Escamilla, M. Guerrero, J. Sort, J. Juanhuix, D. Maspoch and J. Giner Planas, Cryst. Growth Des., 2017, 17, 846–857 CrossRef CAS.
- S. Rodríguez-Hermida, M. Y. Tsang, C. Vignatti, K. C. Stylianou, V. Guillerm, J. Pérez-Carvajal, F. Teixidor, C. Viñas, D. Choquesillo-Lazarte, C. Verdugo-Escamilla, I. Peral, J. Juanhuix, A. Verdaguer, I. Imaz, D. Maspoch and J. Giner Planas, Angew. Chem., Int. Ed., 2016, 55, 16049–16053 CrossRef PubMed.
- Z. Li, X.-B. Li, M. E. Light, A. E. Carrillo, A. Arauzo, M. Valvidares, C. Roscini, F. Teixidor, C. Viñas, F. Gándara, E. Bartolomé and J. G. Planas, Adv. Funct. Mater., 2023, 33, 2307369 CrossRef CAS.
- L. Gan, M. T. Nord, J. M. Lessard, N. Q. Tufts, A. Chidambaram, M. E. Light, H. Huang, E. Solano, J. Fraile, F. Suárez-García, C. Viñas, F. Teixidor, K. C. Stylianou and J. G. Planas, J. Am. Chem. Soc., 2023, 145, 13730–13741 CrossRef CAS PubMed.
- Z. Li, D. Choquesillo-Lazarte, J. Fraile, C. Viñas, F. Teixidor and J. G. Planas, Dalton Trans., 2022, 51, 1137–1143 RSC.
- J. Soldevila-Sanmartín, E. Ruiz, D. Choquesillo-Lazarte, M. E. Light, C. Viñas, F. Teixidor, R. Núñez, J. Pons and J. G. Planas, J. Mater. Chem. C, 2021, 9, 7643–7657 RSC.
- Z. Li, J. Fraile, C. Viñas, F. Teixidor and J. G. Planas, Chem. Commun., 2021, 57, 2523–2526 RSC.
- L. Gan, A. Chidambaram, P. G. Fonquernie, M. E. Light, D. Choquesillo-Lazarte, H. Huang, E. Solano, J. Fraile, C. Viñas, F. Teixidor, J. A. R. Navarro, K. C. Stylianou and J. G. Planas, J. Am. Chem. Soc., 2020, 142, 8299–8311 CrossRef CAS PubMed.
- L. Gan, P. G. Fonquernie, M. E. Light, G. Norjmaa, G. Ujaque, D. Choquesillo-Lazarte, J. Fraile, F. Teixidor, C. Viñas and J. G. Planas, Molecules, 2019, 24, 3204 CrossRef CAS PubMed.
- F. Tan, A. López-Periago, M. E. Light, J. Cirera, E. Ruiz, A. Borrás, F. Teixidor, C. Viñas, C. Domingo and J. G. Planas, Adv. Mater., 2018, 30, 1800726 CrossRef PubMed.
- J. Poater, C. Viñas, I. Bennour, S. Escayola, M. Solà and F. Teixidor, J. Am. Chem. Soc., 2020, 142, 9396–9407 CrossRef CAS PubMed.
- J. Poater, M. Solà, C. Viñas and F. Teixidor, Angew. Chem., Int. Ed., 2014, 53, 12191–12195 CrossRef CAS PubMed.
- J. Poater, C. Viñas, M. Solà and F. Teixidor, Nat. Commun., 2022, 13, 3844 CrossRef CAS PubMed.
- J. Plesek, Chem. Rev., 1992, 92, 269–278 CrossRef CAS.
- M. Scholz and E. Hey-Hawkins, Chem. Rev., 2011, 111, 7035–7062 CrossRef CAS PubMed.
-
R. N. Grimes, Carboranes, Academic Press, 2016 Search PubMed.
-
F. Teixidor and D. E. Kaufmann, Science of Synthesis: Houben-Weyl Methods of Molecular Transformations, Georg Thieme Verlag, Stuttgart, 5th edn, 2015, vol. 6 Search PubMed.
- S. Fujii, MedChemComm, 2016, 7, 1082–1092 RSC.
- F. Issa, M. Kassiou and L. M. Rendina, Chem. Rev., 2011, 111, 5701–5722 CrossRef CAS PubMed.
- J. F. Valliant, K. J. Guenther, A. S. King, P. Morel, P. Schaffer, O. O. Sogbein and K. A. Stephenson, Coord. Chem. Rev., 2002, 232, 173–230 CrossRef CAS.
-
M. A. Fox, Icosahedral carborane derivatives, Doctoral thesis, Durham University, 1991 Search PubMed.
- L. Han, T. Pham, M. Zhuo, K. A. Forrest, S. Suepaul, B. Space, M. J. Zaworotko, W. Shi, Y. Chen, P. Cheng and Z. Zhang, ACS Appl. Mater. Interfaces, 2019, 11, 23192–23197 CrossRef CAS PubMed.
- J. H. van Vleck, Phys. Rev., 1928, 31, 587 CrossRef CAS.
- P. Carra, B. T. Thole, M. Altarelli and X. Wang, Phys. Rev. Lett., 1993, 70, 694–697 CrossRef CAS PubMed.
- B. Thole, P. Carra, F. Sette and G. van der Laan, Phys. Rev. Lett., 1992, 68, 1943–1946 CrossRef CAS PubMed.
-
S. Tripathi, Max-Planck-Institut für Intelligente Systeme, PhD Thesis, Stuttgart, 2018 Search PubMed.
- A. Arauzo, A. Lazarescu, S. Shova, E. Bartolomé, R. Cases, J. Luzón, J. Bartolomé and C. Turta, Dalton Trans., 2014, 43, 12342–12356 RSC.
- M. Evangelisti and E. K. Brechin, Dalton Trans., 2010, 39, 4672–4676 RSC.
- Manuscript in preparation, 2024.
- E. Bartolomé, J. Bartolomé, S. Melnic, D. Prodius, S. Shova, A. Arauzo, J. Luzón, F. Luis and C. Turta, Dalton Trans., 2013, 42, 10153–10171 RSC.
- E. Bartolomé, A. Arauzo, J. Luzón, S. Melnic, S. Shova, D. Prodius, J. Bartolomé, A. Amann, M. Nallaiyan and S. Spagna, Dalton Trans., 2019, 48, 5022–5034 RSC.
- E. Bartolomé, J. Bartolomé, S. Melnic, D. Prodius, S. Shova, A. Arauzo, J. Luzón, L. Badía-Romano, F. Luis and C. Turta, Dalton Trans., 2014, 43, 10999–11013 RSC.
- E. Bartolomé, J. Bartolomé, A. Arauzo, J. Luzón, L. Badía, R. Cases, F. Luis, S. Melnic, D. Prodius, S. Shova and C. Turta, J. Mater. Chem. C, 2016, 4, 5038–5050 RSC.
- C. F. Macrae, I. Sovago, S. J. Cottrell, P. T. A. Galek, P. McCabe, E. Pidcock, M. Platings, G. P. Shields, J. S. Stevens, M. Towler and P. A. Wood, J. Appl. Crystallogr., 2020, 53, 226–235 CrossRef CAS PubMed.
Footnote |
† Electronic supplementary information (ESI) available: S1: Structural characterization: supplementary figures; S2: magnetization field-dependence modeling; S3: corrected sum rules for lanthanide Ln3+ ions. See DOI: https://doi.org/10.1039/d4dt00626g |
|
This journal is © The Royal Society of Chemistry 2024 |