DOI:
10.1039/D4DT00021H
(Paper)
Dalton Trans., 2024,
53, 6275-6281
Heterometallic MIL-125(Ti–Al) frameworks for electrochemical determination of ascorbic acid, dopamine and uric acid†
Received
4th January 2024
, Accepted 7th March 2024
First published on 14th March 2024
Abstract
The detection of ascorbic acid (AA), dopamine (DA), and uric acid (UA) is not only of great significance in the areas of biomedicine and neurochemistry but also helpful in disease diagnosis and pathology research. Due to their diverse structures, designability, and large specific surface areas, metal–organic frameworks (MOFs) have recently caught considerable attention in the electrochemical field. Herein, a family of heterometallic MOFs with amino modification, MIL-125(Ti–Al)-xNH2 (x = 0%, 25%, 50%, 75%, and 100%), were synthesized and employed as electrochemical sensors for the detection of AA, DA, and UA. Among them, MIL-125(Ti–Al)-75%NH2 exhibited the most promising electrochemical behavior with 40% doping of carbon black in 0.1 M PBS (pH = 7.10), which displayed individual detection performance with wide linear detection ranges (1.0–6.5 mM for AA, 5–100 μM for DA and 5–120 μM for UA) and low limits of detection (0.215 mM for AA, 0.086 μM for DA, and 0.876 μM for UA, S/N = 3). Furthermore, the as-prepared MIL-125(Ti–Al)-75%NH2/GCE provided a promising platform for future application in real sample analysis, owing to its excellent anti-interference performance and good stability.
Introduction
As a new emerging class of highly ordered porous materials, metal–organic frameworks (MOFs), also known as porous coordination polymers (PCPs), are constructed from metal-containing nodes and organic linkers.1–5 The structures and structure-related properties of MOFs can be tuned by judicious design of both constituents at the molecular level.6 Owing to their unlimited structural diversity and functional tunability,7,8 MOFs have become one of the most fascinating classes of materials in numerous areas including gas storage and separation,9–11 ion exchange,12 chemical sensing,13–15 catalysis,16–18 energy harvesting,19,20 and biomedicine.21,22 Since multivariate MOFs were reported by the Yaghi group, and more and more multi-component MOFs with complementary or contradictory properties were created to obtain better performance,23–25 tremendous efforts have been devoted to introducing multiple metal centers or ligands to improve the properties of the targeted MOF materials.26,27
Ascorbic acid (AA), dopamine (DA), and uric acid (UA) are essential biomolecules existing in bodily fluids. AA, also known as vitamin C, is an essential nutrient in many physiological and biochemical processes, including the synthesis of collagen and wound healing as well as redox functions.28–31 The abnormality of DA concentration, whether an increase or decrease, has been proven to be closely related to various neurological diseases, for instance, depression, Alzheimer's disease, Parkinson's disease, and even HIV infection.32,33 UA is the final product of purine metabolism, and can be found in different concentrations in urine and human blood plasma. Gout and hyperuricemia are closely related to the concentration of UA in the body.34 In this sense, the rapid and accurate detection of AA, DA, and UA is of great significance not only in the fields of biomedicine and neurochemistry, but also in diagnostic and pathological research.35–37 Due to the fact that these electro-active molecules could be reduced or oxidized at different potential values, electrochemical determination received widespread attention due to its fast response, high sensitivity, good stability, and good selectivity.38,39 Recently, more and more MOFs have been employed in electrochemical studies.40,41 However, MOF materials often suffer from poor conductivity and the inability to simultaneously detect multiple substances. So far, it has been a great challenge to prepare stable MOF materials doped with highly conductive materials to improve the conductivity of the parent MOFs and thus their electrochemical behaviour.
Recently, the [TiIV7CrIII] cluster was established on the basis of the same charge and similar size of the TiO2+ and CrF2+ fragments, and it was subsequently expanded in terms of the MIII centres to include Ga(III), Al(III), and In(III) ions.42,43 In this sense, a stable porous architecture, MIL-125(Ti), was selected as a parent prototype to obtain MIL-125(Ti–M) by transition metal(III) centre replacement. Herein, a heterometallic–organic framework, MIL-125(Ti–Al), was achieved with the introduction of aluminium nitrate as the second metal source. In addition, a series of amino group modified MOF materials, MIL-125(Ti–Al)-xNH2 (x = 25%, 50%, 75%, and 100%), were prepared through the partial replacement of 1,4-benzenedicarboxylic acid (H2BDC) with 2-aminoterephthalic acid (NH2-H2BDC). The MIL-125(Ti–Al)-xNH2 family were utilized as electrochemical sensors for the detection of AA, DA, and UA, and MIL-125(Ti–Al)-75%NH2 doped with 40% carbon black was proved to be the best electrocatalyst with high sensitivity, stability, good repeatability, and reproducibility in 0.1 M PBS (pH = 7.10). Furthermore, the as-prepared MIL-125(Ti–Al)-75%NH2/GCE exhibited excellent selectivity in the presence of a series of interfering substances including inorganic compounds and proteins. In addition, it was also employed for real sample analysis owing to its anti-interference performance and good stability.
Results and discussion
Preparation and characterization
MIL-125(Ti–Al) was achieved by the introduction of Al(III) as a secondary metal source according to the previous work (Fig. S1, ESI†).44,45 A series of MIL-125(Ti–Al)-xNH2 materials were synthesized through the addition of NH2-H2BDC instead of some of H2BDC as the secondary organic linker based on an improved solvothermal process. Correspondingly, the as-synthesized MOF materials were named MIL-125(Ti–Al)-xNH2 (x = 0%, 25%, 50%, 75%, and 100%), according to the feeding ratio of NH2-H2BDC.
As shown in the powder X-ray diffraction (PXRD) patterns (Fig. 1a), the peak positions of the as-prepared products were in good agreement with the simulated pattern of MIL-125(Ti),46,47 which confirmed the successful synthesis of the MIL-125 framework. Moreover, the high purities of the as-prepared samples were confirmed by the sharp and strong diffraction peaks.48 A significant colour difference was found for MIL-125(Ti–Al)-xNH2 (x = 25%, 50%, 75%, and 100%, Fig. S2, ESI†), which can be attributed to the increased ratio of amino groups. Besides, a remarkable difference in the light responses was further confirmed by ultraviolet-visible (UV-vis) spectroscopy. The UV-vis spectra of the MIL-125(Ti–Al)-xNH2 family showed an absorption band from 200 to 320 nm (Fig. 1b), which was induced by the charge transfer from O to Ti centres in the TiO5(OH) inorganic clusters.49 Meanwhile, a peak at ∼380 nm was observed, resulting from the introduction of the amine-functionalized organic fragments, which greatly influenced the visible light response.49–51
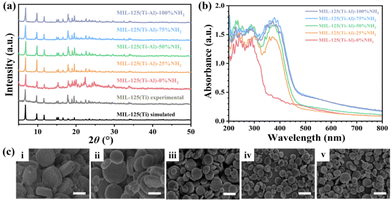 |
| Fig. 1 (a) The PXRD patterns, (b) UV–vis spectra and (c) SEM images (scale bar: 1 μm) of MIL-125(Ti–Al)-xNH2 (x = 0%, 25%, 50%, 75%, and 100%). | |
The morphologies of MIL-125(Ti–Al)-xNH2 (x = 0%, 25%, 50%, 75%, and 100%) were recorded by scanning electron microscopy (SEM). The SEM images of the as-synthesized MIL-125(Ti–Al)-xNH2 materials show a thick round biscuit shape with smooth surfaces (Fig. 1c). As the proportion of amino groups in the organic fragments increased, the diameter of the corresponding products decreased from 1.57 to 0.43 μm, which could be seen more directly in the size distribution histograms shown in Fig. S3 (ESI†). These results are perhaps caused by the presence of the amino groups in the organic fragments, which promotes a rapid nucleation that limits the particle growth. Meanwhile, the successful construction of MIL-125(Ti–Al)-xNH2 could be further supported by energy-dispersive X-ray spectroscopy (EDS, Fig. S4 and S5, ESI†). In addition, the ratios of metal elements before and after the reaction process are displayed in Table S1 (ESI†). The feeding ratio of Ti
:
Al was 9
:
1, however, the Ti
:
Al ratio was approximately 5.5
:
2.5 in the as-synthesized MIL-125(Ti–Al)-xNH2 series as determined through inductively coupled plasma atomic emission spectroscopy analysis.
Electrochemical characterization
The electrochemical method was used to characterize the charge transfer characteristics of MIL-125(Ti–Al)-xNH2/GCE. The cyclic voltammogram (CV) curves of MIL-125(Ti–Al)-xNH2 (x = 25%, 50%, 75%, and 100%) were obtained in 0.1 M KCl solution containing 5 mM [Fe(CN)6]3−/4− (1
:
1) (Fig. S6a, ESI†). In addition, a bare glassy carbon electrode (GCE) was tested for comparison. Obviously, a pair of well-defined quasi-reversible redox peaks appeared for the modified electrodes, and the peak current signal of MIL-125(Ti–Al)-75%NH2/GCE was significantly larger than those of the other materials. Furthermore, the relationship between the peak currents of the modified electrodes and the scanning rates was also studied (Fig. S7a–e, ESI†). The peak currents were proportional to the square root of the scan rates (Fig. S7f, ESI†), indicating that it is a diffusion-controlled process. In addition, electrochemical impedance spectroscopy (EIS) was performed to evaluate the conductivity of MIL-125(Ti–Al)-xNH2 (x = 0%, 25%, 50%, 75%, 100%)/GCE (Fig. S6b, ESI†). The Nyquist plots of the MIL-125(Ti–Al)-xNH2 series were fitted to an equivalent circuit (Fig. S6b, inset, ESI†). The charge-transfer resistance (Rct) values at the surface of the catalysts can be assessed from the diameters of the semicircles at high frequencies in the Nyquist plots, which vary inversely with the electrocatalytic activity. The diameter of MIL-125(Ti–Al)-75%NH2 was much lower than those of the other materials, suggesting the smallest Rct value of MIL-125(Ti–Al)-75%NH2.
Optimized conditions
It is well-known that the pH value of an analytical system is of great significance for electrochemical exploration,38 as protons participate in electrode reactions. Herein, MIL-125(Ti–Al)-50%NH2 was taken as an example to prepare a modified electrode after doping with 50% mass fraction carbon black (Vulcan XC-72R). The electrochemical studies were carried out in 0.1 M PBS containing 1.0 mM AA, 30 μM DA, and 50 μM UA with the pH varied from 3.00 to 8.10. The oxidation peak potentials were all affected by the acidity and alkalinity of the solution as shown in Fig. 2a. As the pH increased, the oxidation potentials of AA, DA, and UA shifted to the negative potential direction (Fig. 2b). In addition, the oxidation peak potentials of AA, DA, and UA exhibited linear relationships with pH, with slopes of 0.0507, 0.0614 and 0.0649 V per pH. These values were close to the theoretical value of 0.0590 V per pH calculated by the Nernst equation, illustrating that the numbers of electrons and protons were equal and all reactions were isoelectronic–isoprotic processes.52–54 Moreover, the relationship between anodic peak currents and pH was also explored (Fig. 2c). With the increase of pH values, the peak currents of DA and UA did not change significantly in the range of 3.00–7.10. The peak current of AA showed a decreasing trend overall. To determine these three substances sensitively at the same time considering the physiological pH value, a pH value of 7.10 was selected for further study.
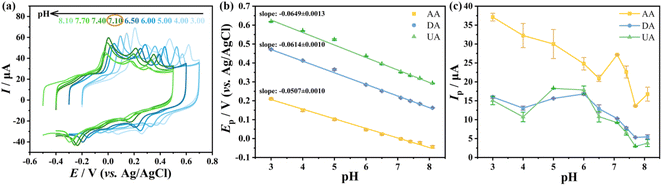 |
| Fig. 2 (a) CV curves of MIL-125(Ti–Al)-50%NH2/GCE with 50% Vulcan XC-72R doping at different pH values in 0.1 M PBS containing [AA]: 1 mM, [DA]: 30 μM, and [UA]: 50 μM. Scan rate: 100 mV s−1. The relationship between pH values and peak potentials (b) and currents (c). | |
Furthermore, the optimum conditions were applied with different MOF materials and doping ratios of carbon materials as follows. The different modified electrode materials were studied in the presence of 1.0 mM AA, 30 μM DA, and 50 μM UA in 0.1 M PBS at pH 7.10 (Fig. S8a, ESI†). As for the bare GCE, close oxidation potentials and broad oxidation peaks were observed, demonstrating that the bare GCE couldn't be used for the simultaneous detection of AA, DA, and UA. The anodic peak currents on MIL-125(Ti–Al)-xNH2/GCE were 5 times those of the bare GCE, respectively. The separation of the CV responses between AA and DA (ΔEp1 = EPDA − EPAA) was around 210 mV, while the separation between DA and UA (ΔEp2 = EPUA − EPDA) was around 150 mV, which was broad enough for application in simultaneous electrochemical determination. The GCE modified with the MIL-125(Ti–Al)-xNH2 series had a good catalytic activity for the oxidation of AA, DA, and UA, suggesting that as-prepared sensors can be utilized for the detection of these three biomolecules simultaneously. Among them, the best-modified electrode was MIL-125(Ti–Al)-75%NH2/GCE, owing to its best resolution and high sensitivity. The doping with conductive materials like carbon black increased the conductivity and surface area of the electroactive species when compared to the parent materials.55 A series of doping amounts of Vulcan XC-72R was tested in 0.1 M PBS (pH = 7.10) containing 1 mM AA, 30 μM DA, and 50 μM UA, and a 40% doping ratio was selected as the optimal ratio with the largest peak current and peak separation (Fig. S8b, ESI†).
In addition, the reaction kinetics of MIL-125(Ti–Al)-75%NH2/GCE was studied under the optimum conditions. The CV curves were obtained at different scanning rates ranging from 25 to 200 mV s−1. As shown in Fig. S9 (ESI†), linear relationships between anodic peak currents and square roots of the scan rate were found as Ipa(AA)/μA = 4.76 + 44.35v1/2/(V s−1) (R2 = 0.9844), Ipa(DA)/μA = −3.06 + 21.81v1/2/(V s−1) (R2 = 0.9839), Ipa(UA)/μA = −4.99 + 85.33v1/2/(V s−1) (R2 = 0.9970). These results indicated that the electrode reactions of AA, DA, and UA were diffusion-controlled processes.
Individual and simultaneous determination
The differential pulse voltammetry (DPV) technique is usually utilized to separate the oxidative signals from DA, UA, and AA.56,57 The determination of AA, DA, and UA concentrations was performed using the MIL-125(Ti–Al)-75%NH2/GCE electrode with the DPV method. Under the optimized conditions, the potential range of −0.15 V to 0.5 V was chosen for the following measurements. A linear response was obtained for the individual determination of AA, DA, and UA in the concentration ranges of 1.0 mM to 6.5 mM, 5 μM to 100 μM and 5 μM to 120 μM (Fig. 3a–c), respectively. The calibration plots were further plotted accordingly with correlation coefficients of 0.9998, 0.9859, and 0.9987 as shown in Fig. 3d–f. The limit of detection (LOD, S/N = 3) of AA, DA, and UA was calculated to be 0.215 mM, 0.086 μM, and 0.876 μM, respectively.
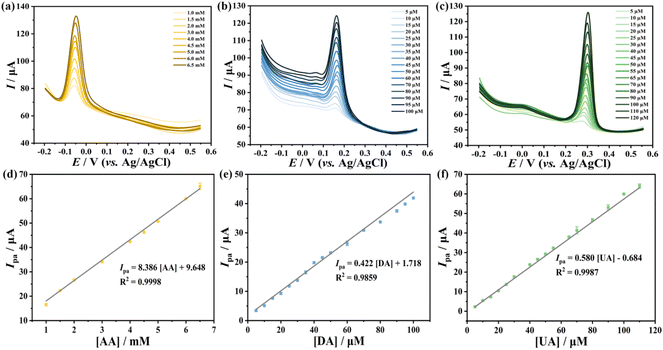 |
| Fig. 3 (a–c) DPV curves and (d–f) calibration plots of individual determination of AA, DA, UA by MIL-125(Ti–Al)-75%NH2/GCE at different concentrations in 0.1 M PBS (pH 7.10). | |
Furthermore, the outstanding performance of MIL-125(Ti–Al)-75%NH2/GCE was also confirmed by the simultaneous determination of the three kinds of biomolecules. As expected, the change in the AA concentration did not significantly affect the peak currents and peak potentials of the other two compounds in Fig. 4a. Similarly, as shown in Fig. 4b and c, while maintaining a constant concentration of the other two compounds, the oxidation peak currents of DA and UA increased with their increasing concentration. It is worth noting that the UA peak slightly decreases with the increasing concentration of DA (Fig. 4b), because the concentration of DA has a certain influence on the UA peak.56 Three clear and well-separated peaks were observed at about −0.025 V for AA, 0.180 V for DA, and 0.350 V for UA, allowing us to detect DA, AA, and UA simultaneously. The current responses for the target molecules (AA, DA, or UA) increased linearly with the concentrations of the corresponding molecules (Fig. 4d–f). The linear range of the concentration for the determination of AA, DA, and UA was 1.0 to 3.5 mM, 5 to 90 μM and 5 to 90 μM with LOD values (S/N = 3) of 0.224 mM, 0.056 μM and 0.558 μM, respectively. The low detection limits and wide linear ranges of MIL-125(Ti–Al)-75%NH2 provided a promising platform for the real application requirements, as reported in the literature (Table S2, ESI†). The good biosensing performance of MIL-125(Ti–Al)-75%NH2 can be attributed to the introduction of Al sites and amino groups.
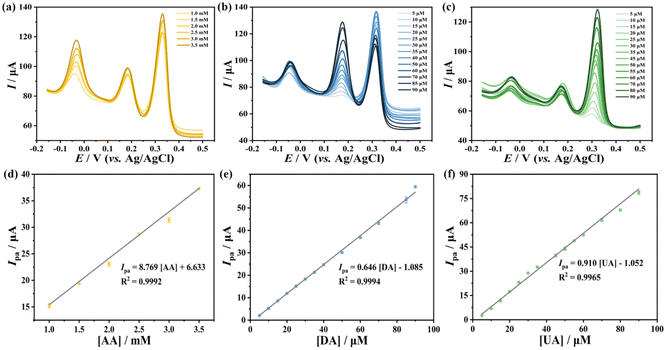 |
| Fig. 4 (a–c) DPV curves and (d–f) calibration plots of simultaneous determination of AA, DA, UA by MIL-125(Ti–Al)-75%NH2/GCE at different concentrations of AA ([DA]: 40 μM, [UA]: 70 μM), DA ([AA]: 1.5 mM, [UA]: 80 μM), UA ([AA]: 1.0 mM, [DA]: 25 μM) in 0.1 M PBS (pH 7.10). | |
Selectivity and stability studies
The selectivity of the proposed sensor was further evaluated in the presence of a series of interfering substances in PBS containing 1.5 mM AA, 35 μM DA, and 50 μM UA at pH = 7.10. The interfering substances not only included common interfering molecules like citric acid (CA), lactic acid (LA), glucose (Glu), L-phenylalanine (L-Phe), glycine (Gly), and L-cysteine (L-Cys), but also included inorganic salts such as NaCl, KCl, MgSO4, CaCl2, and Na2CO3. No obvious interference for the detection of AA, DA, and UA was observed (Fig. 5a), even at high concentrations of these organic and inorganic species. The results suggested that the as-prepared MIL-125(Ti–Al)-75%NH2/GCE electrode showed an excellent sensing performance.
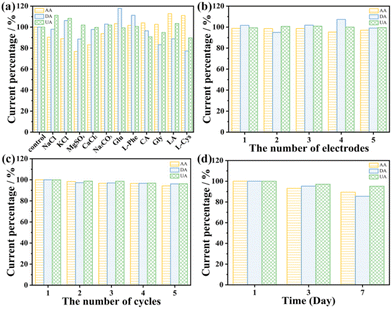 |
| Fig. 5 (a) Selectivity, (b) reproducibility, (c) cycling stability, and (d) long-term stability of MIL-125(Ti–Al)-75%NH2/GCE for the simultaneous detection of AA, DA, and UA. | |
Furthermore, the reproducibility was investigated by using five MIL-125(Ti–Al)-75%NH2/GCE electrodes prepared under the same conditions (Fig. 5b). The relative standard deviation (RSD) calculated for 1.5 mM AA, 35 μM DA, and 50 μM UA was 2.4%, 4.2% and 0.6% (n = 5), respectively. This result revealed that MIL-125(Ti–Al)-75%NH2 possessed good repeatability. Additionally, the stability of the proposed sensor was verified through two methods. Firstly, it was evaluated by a series of repeated measurements of the responses to a mixture of AA (1.5 mM), DA (35 μM), and UA (50 μM) (Fig. 5c). The RSD values for AA, DA, and UA were 3.4%, 1.5%, and 1.5%, respectively. Secondly, the long-term stability of the MIL-125(Ti–Al)-75%NH2/GCE modified electrode was also investigated for AA, DA, and UA (Fig. 5d), and it retained 89.4%, 85.5%, and 95.2% of its initial responses. These results suggested that MIL-125(Ti–Al)-75%NH2 exhibited excellent stability.
Real sample analyses
To exemplify the applicability of the prepared sensor in practical sample analysis, the analytical reliability and applied potentiality were further explored via the standard addition method. Vitamin C, dopamine hydrochloride injection, and human sweat samples were selected for the subsequent experiments. Firstly, 0.028 g of vitamin C tablets (122 mg/0.65 g) were ground and dissolved in 30 mL PBS (0.1 M, pH 7.10). To guarantee correctness, the diluted samples mentioned above were spiked with certain amounts of AA, DA, and UA before being monitored. Secondly, 8 μL of dopamine hydrochloride injection (20 mg/2 mL) was diluted in 20 mL PBS (0.1 M, pH 7.10) for the detection of DA. Thirdly, human sweat samples were directly employed after centrifugation for the detection of UA. The results are listed in Table 1. The recovery of the spiked samples ranged between 96.0 and 102.8%, which suggested the applicability of the MIL-125(Ti–Al)-75%NH2-based sensor in analytical applications.
Table 1 Determination of AA, DA, and UA levels in real samples using MIL-125(Ti–Al)-75%NH2/GCE in 0.1 M PBS (pH 7.10, each result was the average of three determinations)
Samples |
Analyte |
Added (μM) |
Found (μM) |
Recovery (%) |
RSD (%) |
ND: not detected. |
Vitamin C |
AA |
— |
991 |
— |
— |
500 |
1442 |
96.0 |
1.1 |
1500 |
2475 |
99.0 |
2.1 |
2000 |
3003 |
100.1 |
1.6 |
DA |
— |
ND |
— |
— |
10.00 |
10.27 |
102.7 |
0.5 |
25.00 |
25.71 |
102.8 |
0.6 |
55.00 |
54.30 |
98.7 |
1.8 |
UA |
— |
ND |
— |
— |
15.00 |
14.85 |
99.0 |
1.1 |
20.00 |
20.27 |
101.4 |
1.5 |
40.00 |
39.43 |
98.6 |
1.6 |
Dopamine hydrochloride injection |
DA |
— |
19.78 |
— |
— |
20.00 |
41.02 |
102.6 |
0.6 |
40.00 |
58.06 |
96.8 |
0.9 |
60.00 |
78.49 |
98.1 |
1.3 |
Human sweat samples |
UA |
— |
15.12 |
— |
— |
5.00 |
20.22 |
101.1 |
2.2 |
15.00 |
29.91 |
99.7 |
1.8 |
30.00 |
44.76 |
99.5 |
1.9 |
Conclusions
In summary, a family of heterometallic mixed-ligand MOF materials, MIL-125(Ti–Al)-xNH2 (x = 0%, 25%, 50%, 75%, and 100%), were successfully prepared and employed as electrochemical sensors for the detection of AA, DA, and UA. Among them, MIL-125(Ti–Al)-75%NH2 exhibited the most promising behaviour with 40% doping of Vulcan XC-72R in 0.1 M PBS (pH = 7.10). The three biomolecules can be detected selectively or simultaneously by MIL-125(Ti–Al)-75%NH2/GCE with good selectivity, reproducibility, and stability. Moreover, MIL-125(Ti–Al)-75%NH2/GCE exhibited good performance for the detection of AA, DA, and UA in real samples. The present work not only supplies new heterometallic mixed-ligand MOFs for biomolecule detection but also provides new enlightenment for the future application of MOF materials in areas like intelligent monitoring of human health. More work is currently underway in our lab.
Conflicts of interest
There are no conflicts to declare.
Acknowledgements
The authors gratefully acknowledge the financial support from the Natural Science Foundation of Science and Technology Department of Jilin Province (grant no. 20210101131JC) and the Fundamental Research Funds for the Central Universities (grant no. 2412020FZ009 and 2412022ZD048).
For our experiments with human sweat, we obtained permission from the Ethics Committee of our University (No. 202302017).
References
- S. Furukawa, J. Reboul, S. Diring, K. Sumida and S. Kitagawa, Chem. Soc. Rev., 2014, 43, 5700 RSC.
- Y. Gu, Y.-N. Wu, L. Li, W. Chen, F. Li and S. Kitagawa, Angew. Chem., Int. Ed., 2017, 56, 15658–15662 CrossRef CAS PubMed.
- S. Horike and S. Kitagawa, Nat. Mater., 2022, 21, 983–985 CrossRef CAS PubMed.
- T. Kitao, Y. Zhang, S. Kitagawa, B. Wang and T. Uemura, Chem. Soc. Rev., 2017, 46, 3108–3133 RSC.
- B. Dutta, F. Ahmed and M. H. Mir, Dalton Trans., 2023, 52, 17084–17098 RSC.
- V. R. Bakuru, B. Velaga, N. R. Peela and S. B. Kalidindi, Chem. – Eur. J., 2018, 24, 15978–15982 CrossRef CAS PubMed.
- J. Su, P. Jing, K. Jiang and J. Du, Dalton Trans., 2022, 51, 8938–8944 RSC.
- Y. Mu, Z. Zhang, Z. Yang, C. Yue, Z. Liu, D. Dastan, X.-T. Yin and X. Ma, Dalton Trans., 2023, 52, 18257–18267 RSC.
- J. Zheng, X. Cui, Q. Yang, Q. Ren, Y. Yang and H. Xing, Chem. Eng. J., 2018, 354, 1075–1082 CrossRef CAS.
- A. F. Sahayaraj, H. J. Prabu, J. Maniraj, M. Kannan, M. Bharathi, P. Diwahar and J. Salamon, J. Inorg. Organomet. Polym., 2023, 33, 1757–1781 CrossRef.
- G. Li, H. Kobayashi, J. M. Taylor, R. Ikeda, Y. Kubota, K. Kato, M. Takata, T. Yamamoto, S. Toh, S. Matsumura and H. Kitagawa, Nat. Mater., 2014, 13, 802–806 CrossRef CAS PubMed.
- C. K. Brozek and M. Dincă, Chem. Soc. Rev., 2014, 43, 5456–5467 RSC.
- J. F. Olorunyomi, S. T. Geh, R. A. Caruso and C. M. Doherty, Mater. Horiz., 2021, 8, 2387–2419 RSC.
- H.-Z. Li, Y. Pan, Q. Li, Q. Lin, D. Lin, F. Wang, G. Xu and J. Zhang, J. Mater. Chem. A, 2023, 11, 965–971 RSC.
- X. C. Zhou, C. Liu, J. Su, Y. F. Liu, Z. Mu, Y. Sun, Z. M. Yang, S. Yuan, M. Ding and J. L. Zuo, Angew. Chem., Int. Ed., 2023, 62, 202211850 CrossRef PubMed.
- D. Sun, W. Liu, Y. Fu, Z. Fang, F. Sun, X. Fu, Y. Zhang and Z. Li, Chem. – Eur. J., 2014, 20, 4780–4788 CrossRef CAS PubMed.
- D.-F. Lu, S. Li, M.-X. Guan, Y.-P. Han, Y. Sun, X. Wu, F. Wang and J. Zhang, ACS Mater. Lett., 2023, 5, 2836–2842 CrossRef CAS.
- R. Patra, S. Mondal and D. Sarma, Dalton Trans., 2023, 52, 17623–17655 RSC.
- S. Hajra, M. Sahu, A. M. Padhan, I. S. Lee, D. K. Yi, P. Alagarsamy, S. S. Nanda and H. J. Kim, Adv. Funct. Mater., 2021, 31, 2101829 CrossRef CAS.
- A. Radwan, H. Jin, D. He and S. Mu, Nano-Micro Lett., 2021, 13, 132 CrossRef CAS PubMed.
- A. Bieniek, A. P. Terzyk, M. Wiśniewski, K. Roszek, P. Kowalczyk, L. Sarkisov, S. Keskin and K. Kaneko, Prog. Mater. Sci., 2021, 117, 100743 CrossRef CAS.
- F. Yang, J. Dong, Z. Li and Z. Wang, ACS Nano, 2023, 17, 4102–4133 CrossRef CAS PubMed.
- S. Yuan, J.-S. Qin, J. Li, L. Huang, L. Feng, Y. Fang, C. Lollar, J. Pang, L. Zhang, D. Sun, A. Alsalme, T. Cagin and H.-C. Zhou, Nat. Commun., 2018, 9, 808 CrossRef PubMed.
- H. Deng, C. J. Doonan, H. Furukawa, R. B. Ferreira, J. Towne, C. B. Knobler, B. Wang and O. M. Yaghi, Science, 2010, 327, 846–850 CrossRef CAS PubMed.
- S. Zhang, P. Ling, Y. Chen, J. Liu and C. Yang, Diamond Relat. Mater., 2023, 135, 109811 CrossRef CAS.
- J. N. Lu, J. J. Liu, L. Z. Dong, J. M. Lin, F. Yu, J. Liu and Y. Q. Lan, Angew. Chem., Int. Ed., 2023, 62, e202308505 CrossRef CAS PubMed.
- Y. Li, J. Su, Y. Zhao, L. Feng, L. Gao, X. Xu, Y. Yin, Y. Liu, P. Xiao, L. Yuan, J.-S. Qin, Y. Wang, S. Yuan, H. Zheng and J.-L. Zuo, J. Am. Chem. Soc., 2023, 145, 10227–10235 CrossRef CAS PubMed.
- H. Li, Y. Zhou and J. Du, J. Photochem. Photobiol., A, 2022, 429, 113945 CrossRef CAS.
- S. C. Rumsey and M. Levine, J. Nutr. Biochem., 1998, 9, 117–130 CrossRef.
- J. Du, J. J. Cullen and G. R. Buettner, Biochim. Biophys. Acta, Rev. Cancer, 2012, 1826, 443–457 CrossRef CAS PubMed.
- S. Padayatty and M. Levine, Oral Dis., 2016, 22, 463–493 CrossRef CAS PubMed.
- S. Liu, L. Wang, G. Duan, L. Wang, Z. U. H. Khan, Y. Sun, Y. Chen and P. Wan, Electroanalysis, 2018, 30, 2035–2043 CrossRef CAS.
- M. Taleb, R. Ivanov, S. Bereznev, S. H. Kazemi and I. Hussainova, Microchim. Acta, 2017, 184, 4603–4610 CrossRef CAS.
- S. Copur, A. Demiray and M. Kanbay, Eur. J. Intern. Med., 2022, 103, 4–12 CrossRef CAS PubMed.
- J. Pan, M. Liu, D. Li, H. Zheng and D. Zhang, J. Pharm. Anal., 2021, 11, 699–708 CrossRef PubMed.
- L. Jothi, S. Neogi, S. J. Kumar and G. Nageswaran, Biosens. Bioelectron., 2018, 105, 236–242 CrossRef PubMed.
- B. Wu, L. Xiao, M. Zhang, C. Yang, Q. Li, G. Li, Q. He and J. Liu, J. Solid State Chem., 2021, 296, 122023 CrossRef CAS.
- Y. Wu, P. Deng, Y. Tian, J. Feng, J. Xiao, J. Li, J. Liu, G. Li and Q. He, J. Nanobiotechnol., 2020, 18, 112 CrossRef CAS PubMed.
- Y. Wu, P. Deng, Y. Tian, Z. Ding, G. Li, J. Liu, Z. Zuberi and Q. He, Bioelectrochemistry, 2020, 131, 107393 CrossRef CAS PubMed.
- X.-H. Liang, A.-X. Yu, X.-J. Bo, D.-Y. Du and Z.-M. Su, Coord. Chem. Rev., 2023, 497, 215427 CrossRef CAS.
- X. Zha, W. Yang, L. Shi, Q. Zeng, J. Xu and Y. Yang, Dalton Trans., 2023, 52, 2631–2640 RSC.
- G. A. Timco, A. Fernandez, A. K. Kostopoulos, C. A. Muryn, R. G. Pritchard, I. Strashnov, I. J. Vitorica-Yrezebal, G. F. S. Whitehead and R. E. P. Winpenny, Angew. Chem., Int. Ed., 2017, 56, 13629–13632 CrossRef CAS PubMed.
- L. Feng, J. Pang, P. She, J. L. Li, J. S. Qin, D. Y. Du and H. C. Zhou, Adv. Mater., 2020, 32, 2004414 CrossRef PubMed.
- M. Dan-Hardi, C. Serre, T. Frot, L. Rozes, G. Maurin, C. Sanchez and F. Gérard, J. Am. Chem. Soc., 2009, 131, 10857–10859 CrossRef CAS PubMed.
- C.-L. Yang, G.-H. Yu, A.-X. Yu, D.-Y. Du and Z. Su, Cryst. Growth Des., 2022, 22, 6960–6966 CrossRef CAS.
- C. Zlotea, D. Phanon, M. Mazaj, D. Heurtaux, V. Guillerm, C. Serre, P. Horcajada, T. Devic, E. Magnier, F. Cuevas, G. Férey, P. L. Llewellyn and M. Latroche, Dalton Trans., 2011, 40, 4879 RSC.
- Y. Zhang, B. Fu, K. Liu, Y. Zhang, X. Li, S. Wen, Y. Chen and S. Ruan, Sens. Actuators, B, 2014, 201, 281–285 CrossRef CAS.
- N. Belzile and Y.-W. Chen, Appl. Geochem., 2017, 84, 218–243 CrossRef CAS.
- S. Hu, M. Liu, K. Li, Y. Zuo, A. Zhang, C. Song, G. Zhang and X. Guo, CrystEngComm, 2014, 16, 9645–9650 RSC.
- S.-N. Kim, J. Kim, H.-Y. Kim, H.-Y. Cho and W.-S. Ahn, Catal. Today, 2013, 204, 85–93 CrossRef CAS.
- P. George and P. Chowdhury, Microporous Mesoporous Mater., 2019, 288, 109591 CrossRef CAS.
- M. Zhao, Z. Li, X. Zhang, J. Yu, Y. Ding, H. Li and Y. Ma, Sens. Actuators, B, 2020, 309, 127757 CrossRef CAS.
- G. Hu, Y. Ma, Y. Guo and S. Shao, Electrochim. Acta, 2008, 53, 6610–6615 CrossRef CAS.
- G. Wang, J. Meng, H. Liu, S. Jiao, W. Zhang, D. Chen and B. Fang, Electrochim. Acta, 2008, 53, 2837–2843 CrossRef CAS.
- F. C. Vicentini, P. A. Raymundo-Pereira, B. C. Janegitz, S. A. S. Machado and O. Fatibello-Filho, Sens. Actuators, B, 2016, 227, 610–618 CrossRef CAS.
- S. Thiagarajan and S.-M. Chen, Talanta, 2007, 74, 212–222 CrossRef CAS PubMed.
- Z.-H. Sheng, X.-Q. Zheng, J.-Y. Xu, W.-J. Bao, F.-B. Wang and X.-H. Xia, Biosens. Bioelectron., 2012, 34, 125–131 CrossRef CAS PubMed.
Footnotes |
† Electronic supplementary information (ESI) available: Additional synthesis and electrochemical study, CV and DPV curves. See DOI: https://doi.org/10.1039/d4dt00021h |
‡ Present address: School of Chemistry and Chemical Engineering, Huazhong University of Science and Technology, Wuhan 430074, P. R. China. |
|
This journal is © The Royal Society of Chemistry 2024 |