DOI:
10.1039/D4CY00009A
(Review Article)
Catal. Sci. Technol., 2024,
14, 4087-4105
Electrochemical catalysis for the production of green cement: towards decarbonizing the cement industry
Received
3rd January 2024
, Accepted 19th June 2024
First published on 20th June 2024
Abstract
Among the most significant sources of CO2 emissions, cement manufacturing contributes 8% of such emissions worldwide. The CO2 emissions are generated from a few key sources: around 60% comes from the decomposition of limestone into calcium oxide, 30% from the combustion of fossil fuels (mainly coal), and roughly 10% from power consumption. To reduce CO2 emissions during cement manufacturing, alternative fuels, binders, and renewable energy sources could be utilized to achieve carbon neutrality. However, the reduction of CO2 released from the conversion process of limestone into calcium oxide still presents a significant challenge. This review provides a comprehensive analysis of different electrochemical production approaches of green cement. One of these promising approaches is the decarbonization of CaCO3 into Ca(OH)2, while generating pure H2 at the cathode and O2/CO2 gas mixtures at the anode, using several pathways. Ca(OH)2 is a precursor to cement clinker, whose primary use is to manufacture Portland cement. The produced concentrated gas streams of H2 and/or O2 can be used to produce electricity with extremely little pollution through fuel cells or combustion engines. Although hydrogen is the basic fuel for fuel cells, fuel cells require oxygen too. Large portions of hydrogen and oxygen combine to form a harmless by-product, which is water. From an economic point of view, this produced electrical current can be directed to the green cement manufacturing process and can be used to power any electric motor or device.
1. Introduction
The construction industry is a dynamic and vital sector of the global economy. Cement, the primary construction material, plays a crucial role worldwide. Compared to other substances, cement is a highly abundant and affordable material with intriguing properties, including physical, chemical, and mechanical stability. Fig. 1 reveals the increase in cement production from 1390 to 4100 million tons from 1995 to 2023, demonstrating how much the construction industry has expanded lately.1 Also, there is always an increasing demand for cement compared to other materials, as shown in Fig. 2. However, the cement industry contributes significantly to global pollution. Almost 8% of the carbon dioxide produced by human activities is released by the manufacturing of cement.2 Global cement CO2 emissions per year increased from 576 million tons in 1990 to 1400 million tons in 2002, reaching 2900 million tons in 2021. There are primarily three sources of CO2 emissions during cement manufacturing: about 50% result from the calcination step (decomposition of CaCO3 to CaO and CO2), 40% from fuel combustions in rotary kilns, and 10% from quarrying and product transportation.3 Furthermore, Fig. 3 illustrates the quantities of CO2 gas emitted during the three stages of the cement production process. While the energy-related emissions from fuel combustion and electricity use in cement production account for around 40% of the total greenhouse gas emissions from the global cement industry, the remaining 60% are process-related emissions. These process emissions come specifically from the calcination process, where limestone (CaCO3) is converted into calcium oxide (CaO).4 As a result of the rapidly increasing demand for cement production and the challenge of providing this industry without adding CO2 to the atmosphere make us take serious steps towards the production of cement in a green way to mitigate the environmental footprint of the construction industry. By adopting sustainable practices, such as utilizing alternative raw materials and fuels, industry can significantly minimize its carbon footprint and contribute to a greener future.5
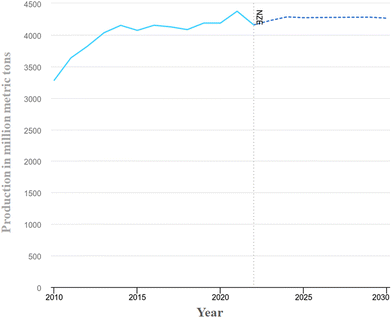 |
| Fig. 1 Production volume of cement worldwide from 2010 to 2022.1 | |
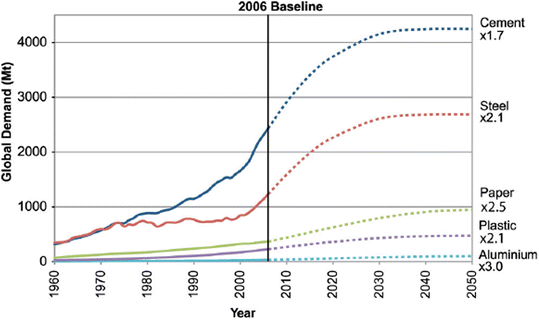 |
| Fig. 2 Worldwide demand for materials.8 | |
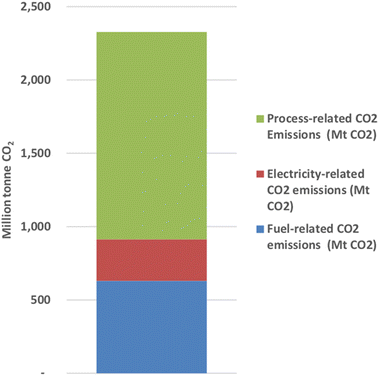 |
| Fig. 3 Global cement industry's CO2 emissions in 2019.4 | |
One promising avenue for producing green cement is the utilization of alternative raw materials, like belite-rich cement, and fuels such as biomass and/or waste-derived fuels. By applying these approaches effectively, the environmental impact of cement production and the reliance on fossil fuels can be greatly reduced.5 The development of modern manufacturing techniques that rely on renewable energy, like electrolysis, has emerged as a compelling and innovative approach. Electrolysis is one of the modern techniques that has attracted much interest lately to produce green cement. Electrochemical processes are expected to have a vital role in developing global power systems. The effective conversion between chemical and electrical energy enables decarbonization of the electric grid and provides novel prospects for different chemical processes. Electrochemical production of cement utilizes water electrolysis to create a pH gradient. While at low pH CaCO3 will be decarbonized and Ca(OH)2 precipitate, at high pH, H2 is formed at the cathode and O2/CO2 mixture is formed at the anode simultaneously. Alite (the primary cementitious phase in Portland cement) is formed via the combination of the precipitated Ca(OH)2 with SiO2.6
The advantages of electrochemical production of green cement are multifaceted. Firstly, Electrolysis does not depend on dangerous agents; instead, it directly depends on electrons obtained from renewable resources. Second, electrochemical production processes can be designed to operate at lower temperatures, reducing energy requirements and associated carbon emissions. In addition, the utilization of electrochemical techniques can facilitate the capture and utilization of carbon dioxide, helping to address the challenge of carbon sequestration. Moreover, electrochemical processes can be independently optimized as they can be split into separate half-reactions, however, thermochemical processes are impractical to be tailored separately.7 However, like any emerging technology, electrochemical production of cement also presents certain disadvantages, such as the need for specialized equipment (certain types of electrodes to achieve high efficiency), stability issues, and the requirement for further research to optimize the process. Fig. 4 reveals how the transition between the thermal pathway to the electrochemical pathway affects CO2 emissions.
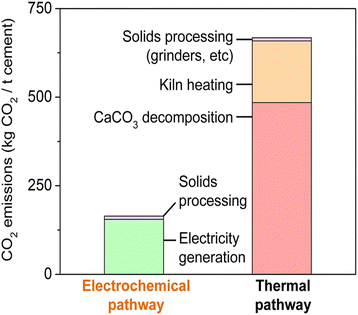 |
| Fig. 4 Comparison between CO2 emissions of electrochemical pathway and thermal pathway.9 | |
Also, the quest for sustainable cement production has led to the exploration of various methods, and electrochemical production holds great promise in achieving this goal. Finally, the selection of appropriate electrode materials directly impacts the performance, durability, and overall efficiency of the electrochemical system. Electrodes with high electrical conductivity, and good chemical stability are crucial for facilitating the desired electrochemical reactions and ensuring long-term operation. Similarly, the electrolyte influences not only the conductivity of the system but also crucial factors such as pH, ion mobility, and mass transport.6,7
This review offers a comprehensive and in-depth analysis of the different possibilities for green cement production. It provides a thorough examination of various scenarios, including a comprehensive overview of alternative fuels and raw materials that can be utilized to reduce CO2 emissions effectively. The review also explores the practical limitations associated with these alternatives. Furthermore, it focuses on the promising field of electrochemical cement production, introducing multiple types of electrodes and electrolytes that play crucial roles in this approach. Overall, this review provides valuable insights into the advancements and challenges in sustainable cement production.
2. Conventional technology of cement production
In the early 19th century, bricklayer Joseph Aspdin of Leeds, England created Portland cement by igniting powdered limestone and clay in his kitchen furnace. He established the industry that yearly processes literally mountains of limestone, clay, and cement rocks into powder that is fine enough to pass through a sieve that can hold water using this basic technique.10 Conventional Portland cement consists mainly of calcium silicate minerals (Table 1). The raw materials are extracted from quarries or mines and transported to the manufacturing facility where they are crushed and ground into a fine powder before entering a massive rotary kiln and being heated to temperatures beyond 1400 °C.11 The product of calcination is known as clinker in the cement industry. Alite (tricalcium silicate) is created during the calcination process, which takes place when calcium carbonate (CaCO3) and silicon dioxide (SiO2) are mixed in a boiler at 1450 °C.12 After cooling the clinker or kiln product and the excess heat is normally returned to the preheater units. Gypsum is added to the clinker to control the setting time before packaging. PC is the finished product, which has very tiny grains (90% ≅ 10 micron). An overview of the overall cement manufacturing process, as well as the inputs and emissions generated during each stage of production, are shown in Fig. 5. The calcination process (the decarbonization of limestone) produces around 50% of the CO2 emissions directly, 40% of the emissions come from the fuel burned in the rotary kiln, and 10% of the emissions come from the quarrying and transportation of the products.13 Due to growing public awareness of global warming, concern over the effects of manmade carbon emissions on the climate has grown in recent years.14 The single most significant industrial source of CO2 emissions is cement production, which accounts for 8% of all the global emissions of CO2.6 In addition to producing CO2, the cement manufacturing process generates millions of tons of waste CKD each year, increasing the risk of respiratory and pollution-related illnesses.11 Thus, the world today is trying to find a solution to reduce these hazard emissions.
Table 1 Raw material composition of clinker, the primary component of PC11
Raw materials |
Sources |
Mass percent |
Lime |
Limestone, shells, chalk |
60–67 |
Silica |
Sand, fly ash |
17–25 |
Alumina |
Clay, shale, fly ash |
2–8 |
Iron oxide |
Iron ore |
0–6 |
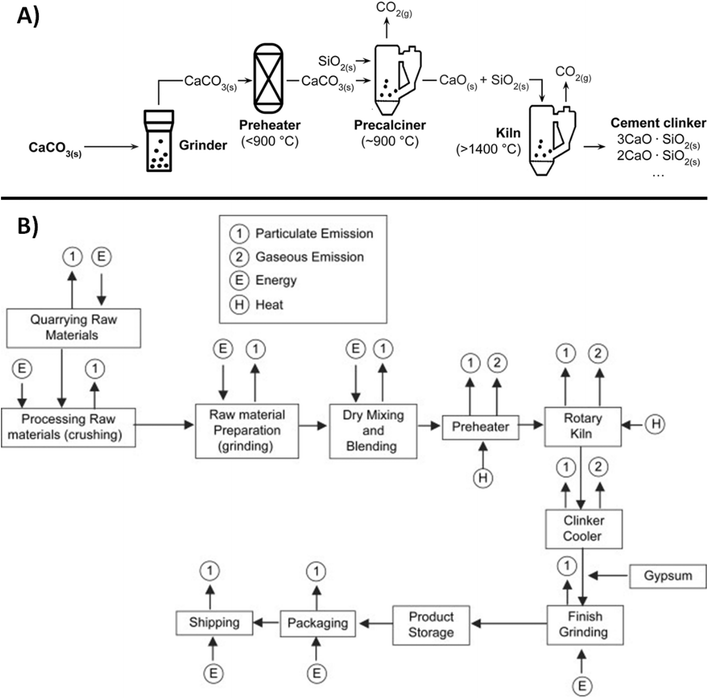 |
| Fig. 5 A) An overview of the incumbent thermal process. B) Process flow diagram for the cement manufacturing process, showing energy and heat consumption or inputs, as well as gaseous and particulate emissions. Note that emissions at the preheating and kiln stages include both fugitive emissions and cement kiln dust or those particulates captured with controlled devices.9,11 | |
3. Green cement production utilizing alternative fuels and raw materials
The recent progress in green cement technology involves the use of various alternative fuels and raw materials to reduce CO2 emissions.
3.1 Alternative fuels
Typically, a clinker's firing process involves using fossil fuels like natural gas, local fuel, or fuel oil. Regrettably, these fuel options play a significant role in the substantial CO2 emissions associated with cement production. The use of alternative fuels (waste tires, sewage sludge, waste oil, and animal residue used to produce clinker) began by the end of the 1980s. It was reported that fuels like biomass, compared to coal, can successfully decrease CO2 emissions by 20–25%,13,15Table 2 illustrates wastes used as alternative fuels and their calorific values content. It shows the calorific values for different wastes that can be used as replacements for fossil fuels, where the husk represents the higher heating values compared to others. Such alternative fuels can reduce the adverse impacts of fossil fuels on the environment.
Table 2 Calorific values of various fuels used in cement16
Wastes |
Energy (MJ Kg−1) |
Used tires |
23.3 |
Husk |
19.93 |
Waste oil |
14.65 |
Plastic |
18.21 |
Scrap paper |
14.23 |
Refused derived fuels |
11.72 |
Sewage sludge |
8.37 |
Due to the chemical and physical differences between these fuels and conventional fuels, there are some real restrictions on their use, many of these fuels may present technical difficulties like poor calorific value as represented in Fig. 6, high moisture content or high concentration of chlorine or any other trace elements. Also, the quantity of the ashes produced by alternative fuels varies significantly from those produced by fossil fuels, introducing some unusual elements into the kiln. Phosphorus is an example, which is primarily found in bones or sewage sludge. The majority of the phosphorous oxide in a solid solution of belite (C2S) is embedded in the crystals. At sintering conditions in the kiln, belite containing a certain quantity of phosphorus does not react with free lime, resulting in clusters of belite and free lime,17 as shown in Fig. 7.
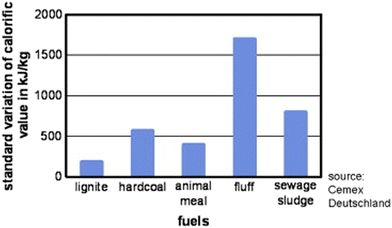 |
| Fig. 6 Standard variations Schneider in calorific values of various fuels.17 | |
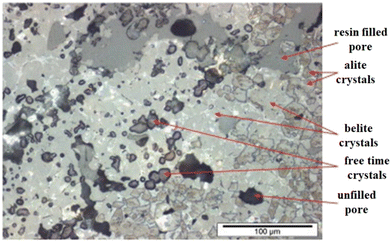 |
| Fig. 7 Belite enriched in P2O5 (light gray), interspersed with fine grained free lime (dark gray).17 | |
Also, more political and legal obstacles stand in the way of higher substitution rates than technical ones:18
- Regulations governing waste management have substantial effect on availability, the higher substitution of alternative fuels occurs when local regulations forbid landfilling and allow the controlled waste collection and treatment of alternative fuels. Therefore, the availability of local waste collection networks is essential.
- With high CO2 costs, alternative fuels prices are expected to rise. It will be more challenging for the cement industry to find sizable amounts of fuels at reasonable costs.
- People frequently worry about harmful emissions from co-processing of waste fuels, even though emissions levels from managed cement plants with alternative fuels are lower than conventional ones.
3.2 Alternative raw materials
The challenge of introducing different raw materials in cement manufacturing is to reduce CO2 emissions and the energy used without sacrificing cement's effectiveness or quality. In this regard, different types of cement were produced upon the use of a plethora of alternative raw materials:
3.2.1 Belite-rich cement.
It is obtained by the same manufacturing process as the standard OPC but utilizes less limestone in a clinker raw blend. Belite is the abundant phase in belite-rich Portland cement, which has a belite content of more than 50%, compared to alite, which is the abundant phase in conventional OPC that makes up roughly 50–65%, while belite makes up only 15–30%. High belite cement exhibits outstanding workability, superior mechanical properties, and durability in addition to low heat evolution.13 In comparison to traditional OPC, it demonstrates higher ultimate strength at later ages,19 as shown in Fig. 8 and reduced drying shrinkage Table 3.
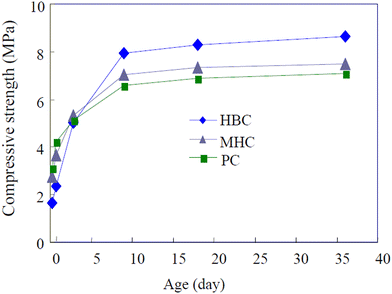 |
| Fig. 8 Strength development of high-belite cement (HBC), moderate heat Portland cement (MHC), and Portland cement (PC).19 | |
Table 3 Drying shrinkage of Portland cement and high-belite cement (%)19
Cement type |
7 days |
14 days |
28 days |
3 months |
6 months |
Portland cement |
0.060 |
0.083 |
0.103 |
0.115 |
0.096 |
High-belite cement |
0.030 |
0.042 |
0.057 |
0.058 |
0.057 |
Production of belite-rich cement requires less energy (the kiln's clinkering temperature is 1350 °C, which is 100 °C lower than what is required for conventional OPC20) and lower CO2 emissions than alite formation. Nevertheless, compared to OPC, the 10% decrease in emissions is not very convincing (Table 4). One significant reason for their limited use is their slower rate of strength development compared to most ordinary Portland cements (OPCs). These cements are particularly well-suited for niche applications, where immediate strength gain is not crucial.21
Table 4 Clinker compounds and associated CO2 emissions21
Clinker compound |
Chemical CO2 emissions (Kg per ton) |
Alite (C3S) |
597 |
Belite (C2S) |
512 |
3.2.2 Magnesium-based cement.
Magnesium oxide is the primary reactive component in Mg cement. Instead of using Portland cement, MgO is used as a building block in Mg-based cements. MgO-based cements will play a key role in the future of environmentally friendly cement production due to: firstly, the lower temperatures needed for the production of MgO compared to the conversion of CaCO3 to CaO and the energy savings connected with this reduced temperature (magnesite is typically calcined at 750 °C to create reactive MgO, which is lower than the processing temperature required to produce PC, which is usually about 1450 °C (ref. 22)), additionally, MgO is a strong candidate for the creation of “carbon-neutral” cements. Such cements have the capability of removing almost as much CO2 from the atmosphere over their service life as was released during their manufacture. In both academic and business circles, the interest in MgO-based cements has significantly increased because of the interaction between these two factors.22
Fig. 9 reveals the differences in thermal decomposition temperatures of magnesite (MgCO3), calcite (CaCO3), and dolomite (CaMg(CO3)2).23 MgO is either produced by wet method or dry method. The dry method involves calcination of mined magnesite (MgCO3), and the wet method from solutions of magnesium-bearing brines or seawater.22 Magnesite must usually be crushed before calcination when using the dry route to produce MgO, also, due to Fe2O3 and SiO2 contaminants, which can negatively impact MgO's usage as a refractory, higher-grade MgO needs precise selection of MgCO3-bearing rocks, or a pretreatment should be done as represented by eqn (1).24
The UK-based firm NOVACEM came up with an interesting method in 2008 to produce this type of cement from magnesium silicate rocks, in order to create magnesium carbonate, magnesium silicates are carbonated at temperatures of 100–225 °C, under a >75% CO
2 atmosphere, and at pressures of 7.1–9.7 MPa as shown in
eqn (2).
22 | Mg2SiO4 + 2CO2(g) → 2MgCO3 + SiO2 | (2) |
Then at 700 °C magnesium oxide is produced by calcination of magnesium carbonate, and the CO
2 can be recycled.
18 However, the “
magnesium oxychloride phase” was found to be unstable after prolonged contact with water. This is due to its leaching out in the form of magnesium chloride and magnesium oxide. Although this type of cement demonstrates high strength, high fire resistance, and absence of wet curing when compared to OPC, the restriction mentioned above prevents the actual application of this type at the construction level.
25 Another reason for unsuitability of MgO-based cement is the lack of available raw materials, and also the significant amount of CO
2 emissions that occur during calcination of magnesite (522 kg of CO
2 per ton of MgCO
3vs. 439.7 kg of CO
2 per ton of CaCO
3).
13 Thus, magnesite is not naturally “green”, CO
2 is produced during production unless MgO is obtained from the calcination of rare naturally occurring brucite deposits or from magnesium silicates.
22
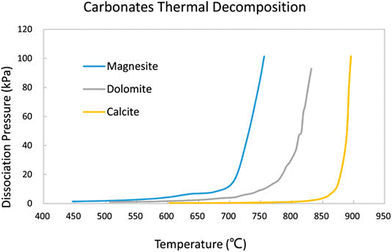 |
| Fig. 9 Carbonates thermal decomposition.23 | |
3.2.3 Calcium sulfo-aluminate cement (CSA).
These types of cement contain high concentrations of alumina. Bauxite, limestone, gypsum, and other materials are combined to create CSA clinker in the kiln.26 The primary components of cement are gypsum, belite (C2S) phase and Ye'elimite (C4A3S). CSA compositions have been shown in Table 5.27 However, there is a real restriction that limit its applicability, the excessive demand for aluminum in raw materials for the production of CSA clinkers, leads to higher costs compared to OPC, since 2004 the output of classical CSA has remained constant at 1.2–1.3 Mt annually.13
Table 5 Mineralogizes of OPC and CSA27
Phase/cement |
OPC |
CSA |
C2S |
Yes |
Yes |
C2A |
Yes |
Yes |
C3S |
Yes |
No |
C3A |
Yes |
Traces |
C4A3S |
No |
Yes |
3.3 Carbon capture, usage, and storage (CCUS)
Carbon capture, usage, and storage (CCUS) is a set of technologies designed to reduce carbon dioxide (CO2) emissions (decarbonization) from industrial processes and power generation. The process starts with capturing CO2 emissions at their source, such as from industrial facilities and power plants. Various methods can be used to capture the CO2, such as pre-combustion, post-combustion, and oxy-fuel combustion. Once captured, CO2 can be utilized in several ways instead of being released into the atmosphere.28 It can be used for enhanced oil recovery (EOR), where CO2 is injected into oil fields to extract additional oil, or converted into useful products like chemicals, fuels, or building materials. Additionally, CO2 can be used in greenhouses to promote plant growth. The final step is transporting and storing the captured CO2 in geological formations, such as depleted oil and gas fields or deep saline aquifers. This long-term storage ensures that CO2 remains trapped underground and does not contribute to atmospheric CO2 levels.29
4. Green cement via electrolysis
4.1 Solar thermal electrochemical method
Licht et al.30 developed a novel thermal chemistry method based on variations in oxide solubility to generate CaO without CO2 emission. The solubility of CaO, which dissolves as calcium hydroxide, is three orders of magnitude greater than CaCO3 (6 × 10−5 m). Surprisingly, this scenario is inverted at high temperatures in molten carbonates, allowing for the endothermic, electrolytic one-pot synthesis and precipitation of CaO (Fig. 10a). The low energy electrolysis of limestone resulted in the production of lime, O2, and reduced carbonate with CO2 emission. Low-temperature electrolysis prefers capture of CO2 as solid carbon at the cathode, eqn (3).
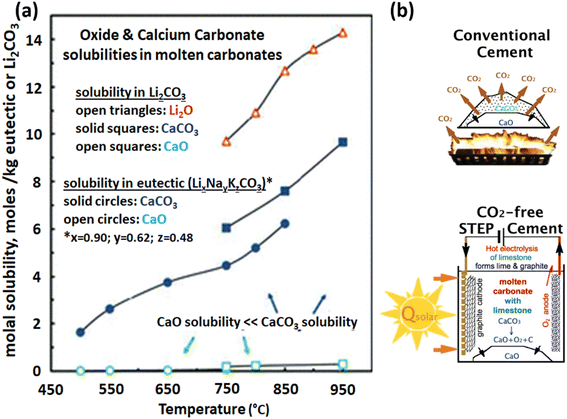 |
| Fig. 10 (a) The low solubility of calcium oxide, compared to calcium carbonate and lithium oxide solubility in molten carbonates and (b) conventional thermal decomposition production of lime versus direct solar conversion of calcium carbonate to calcium oxide.30 | |
In contrast, high-temperature electrolysis lowers the electrolysis potential (the main advantage) and produces CO, which is a helpful reagent, eqn (4). The electrolysis was conducted by using two types of carbonates: lithium carbonate and an alkali carbonate mix, each of them showed low solubility of CaO. Also, the electrolyte is replenished by adding these carbonates for continuing CaO formation. Besides the added carbonate, an amount of CaCO3 was added, precipitating the required CaO product. A comparison between schematic diagrams of conventional thermal decomposition of CaCO3 and solar thermal conversion of CaCO3 to CaO is shown in Fig. 10b.
Below 800 °C:
| CaCO3 + Qsolar + Eelectrolysis → CaOs + O2 + C | (3) |
Below 800 °C:
| CaCO3 + Qsolar + Eelectrolysis → CaOs + 1/2O2 + CO | (4) |
4.2 Seawater electrochemical method
Badjatya et al.31 proposed a process using membrane-free electrolyzers (derived from solar and wind energy) to enable the production of magnesium-based cement by converting Mg2+ in seawater into Mg(OH)2 precursors. Cement can be produced by converting Mg (OH)2 into MgO, which is a low-carbon alternative to Portland cement. Reactive magnesium oxide, that is subject to carbon curing in conditions that are slightly CO2-rich and at room temperatures, produces concrete based on hydrated magnesium carbonate which could achieve compressive strengths of at least 40 MPa, which is durable enough for structural applications.32
Also, this process, compared to OPC manufacturing, consumes lower calcination temperatures (700–1000 °C versus 1450 °C),33 and does not involve direct CO2 emissions. In this process, as shown in Fig. 11, Mg was first extracted from seawater using electrochemical reactors to increase seawater's pH level (by adding NaOH) so that Mg2+ can combine with hydroxyls and create easily separable insoluble Mg (OH)2 particles. According to principle of Le Châtelier, raising the pH causes the state of equilibrium to move to the right, converting Mg2+ into insoluble Mg(OH)2 particles. Initially, the Mg (OH)2 creates a colloidal suspension that gives the solution a milky color, but as the particles continue to grow and become larger, precipitation occurs as gravity pulls the larger particles downward as shown in Fig. 12. It also shows how the amount of NaOH affected the harvested Mg(OH)2. The electrolyzer additionally generates a briny hydrochloric acid, part of this acidic stream is used to make the demineralized water neutral prior being reintroduced to ocean.
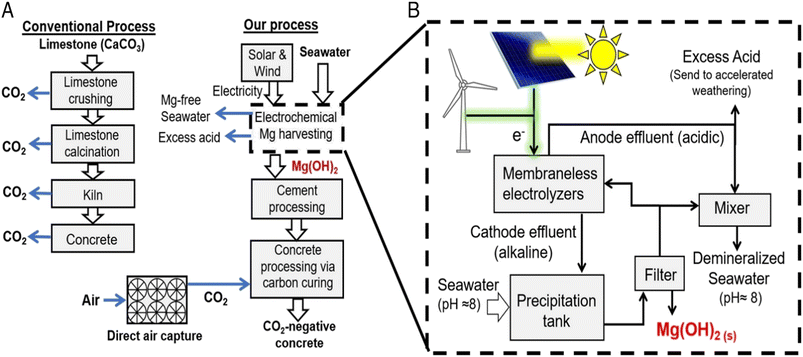 |
| Fig. 11 (A) Process flow diagrams for cement-from-seawater and conventional Portland cement processes. (B) Process flow diagram for the electrochemical technology to harvest hydroxides of alkali earth metal from seawater utilizing renewable source of energy.31 | |
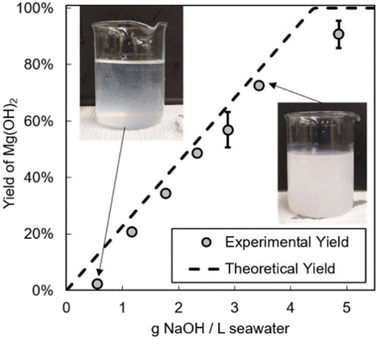 |
| Fig. 12 Theoretical and experimental yields of Mg(OH)2.31 | |
The authors mentioned that the reaction, as represented by eqn (5)–(8), is more effectively for producing acid and base in seawater. This is due to that the chlorine evolution is less favorable than the hydrogen oxidation reaction; compared to the potential needed for water electrolysis, the potential required for the cell is significantly lower, and O2 is not produced at the anode. Hence, there are no safety issues with combining O2 and H2.
| Oxidation half-reaction: H2 → 2H+ + +2e− | (5) |
| Reduction half-reaction: 2H2O + 2e− → H2 + 2OH− | (6) |
| Overall reaction: 2H2O → H+|anode + OH−|cathodeΔE | (7) |
|  | (8) |
Finally, the samples (the wet powders made of Mg(OH)2 derived from seawater) were then compacted into cylinders and cured inside a sealed incubator where they were exposed to a humidified CO2 environment (20% CO2 concentration by volume at 85% relative humidity) at 1 bar total pressure for 48 hours. CO2 curing results in the creation of carbonate phases with various morphologies, which may have an effect on the binding matrix's strength. Regarding energy use, as shown in Table 6, cement industry requires more energy in the case of Mg(OH)2 from seawater compared to Portland cement.31 However, Mg(OH)2 has zero CO2, and this suggested cement type is still very appealing since all energy inputs can be supplied in the form of low-carbon electricity produced from renewable energy sources.
Table 6 Comparison of energy use and CO2 emissions31
Mineral feed stock |
Energy use (kJ per ton of cement) |
CO2 emissions (kg per ton of cement) |
The CO2 footprint for production of seawater-derived Mg cement assumes that all electricity used to power Mg(OH)2 harvesting and processing is provided exclusively by a carbon free electricity generator.
|
Portland cement from CaCO3 |
3.8 × 106 |
793 |
Mg(OH)2 from seawater |
4.4 × 106 |
0a |
The lower alkalinity of Mg cements compared to Portland cements is a huge drawback, which prevents their use for reinforced concrete applications using conventional steel rebar that depend on the high alkalinity of Portland cement made of calcium to avoid corrosion. However, Mg cements are still suitable for unreinforced elements such as concrete masonry units, which comprise 15% of all concrete applications. According to the authors, for this carbon-negative cement production plan to actually happen, considerable advancements must be made: first, the process must be powered by low-cost renewable energy. Second, it is crucial to remember that magnesium-based cement is unsuitable to be used in steel-reinforced concrete since it provides little defense against iron-based rebar corrosion than Portland cement due to its lower alkalinity. A further obstacle to the widespread use of magnesium cement is the difficulty in sufficiently carbonating the thicker components utilized in more significant constructions because of the longer carbonation durations necessary for CO2 to diffuse into their interiors. Finally, based on their approach, we point out that an ideal location for a production facility would be along the shore, not far from the water.
4.3 Electrochemical decarbonization of CaCO3
Ellis et al.6 showed how the decarbonisation cell, which is used in the electrochemical process to create Ca(OH)2 from CaCO3, serves as both an electrolyzer and a chemical reactor. The authors offered numerous ideas for how to include an electrochemical decarbonization reactor into a cement factory that emits little to no carbon. The plant can be run on renewable energy, and the gases produced can be used for a variety of alternate uses. A few of these include the direct capture and sequestration of the inherently concentrated CO2 stream, the production of liquid fuel, the generation of electricity or heat from H2 (and optionally from O2) using fuel cells or combustion engines, and the provision of oxy-fuel for combustion engines in the cement sintering cycle. They observed that the electrochemical cell operates stoichiometrically 1 mol of CaCO3 is transformed into 1 mol of Ca(OH)2 when 2 mol of H+ produced during the electrolysis reaction, as shown in eqn (8) and (14). As a result, the yield and coulombic efficiency are at their greatest levels. In addition, eqn (14) provides the ratio of gases generated during stoichiometric operation. At the cathode, 1 mol of H2 is created for each mole of Ca(OH)2. At the anode, in comparison, 1 mol of O2 and 2 mol of CO2 are generated. The authors claim that at typical cement clinkering temperatures, Ca(OH)2 can produce alite, the main mineral in ordinary Portland cement. The anodic and cathodic half-cell reactions for the two-electrode setup in near-neutral water are as follows: | 2H2O + 2e− → H2 + 2OH− | (10) |
They found out that at a steady state, the electrochemical cell produced a difference in pH that easily can be seen upon adding a universal pH indicator to the cell compartment during operation. In such a system, water is typically created through the reaction of H+ and OH−:
The reactor replaces the process in eqn (11) with a decarbonization reaction. This occurs by adding CaCO3 to the anode part in the H-cell, where an acidic solution is produced during electrolysis. The chemical decarbonization reaction is demonstrated via the following sequence of reactions:
| CaCO3(s) ⇌ Ca2+(aq) + CO2−3(aq) (K = 6 × 10−9) | (12) |
| CO32− + H+ ⇌ HCO3− (K = 2.1 × 1010) | (12.1) |
| HCO3− + H+ ⇌ H2CO3 (K = 2.2 × 106) | (12.2) |
| H2CO3(aq) ⇌ CO2(g) + H2O (K = 5.9 × 102) | (12.3) |
In
eqn (12), the dissolved Ca
2+ is drawn to the anode and,
via reaction with OH
−, precipitates as Ca(OH)
2. Above pH 12.5 the reaction is more favourable:
| Ca2+ + 2OH− → Ca(OH)2 | (13) |
The total of the cell's chemical and electrochemical reactions is:
| 2CaCO3(s) + 4H2O(l) ⇌ 2Ca(OH)2(s) + 2H2(g) + O2(g) + 2CO2(g) | (14) |
The authors used SEM to observe the Ca(OH)2 particles and confirmed that they crystallize into three unique length scales. The biggest individual crystallites are tens of micrometres in size and Fig. 13A demonstrates that they exhibit the usual hexagonal prism shape of Ca(OH)2. Aggregates of even smaller crystallites with dimensions of a few micrometres (Fig. 13B and C) with a similar hexagonal-prism shape are the next in size order. Last but not least, there are precipitates with spherical nodular morphologies that, when magnified, show crystallites with a submicrometer size. (Fig. 13D and E). They concluded that the presence of three distinct Ca(OH)2 precipitate morphologies shows that the reactor has a wide range of nucleation and growth conditions. Nonetheless, a significant portion of the generated particles is below the standard criteria (<90 μm) for raw mixes utilized in cement production.
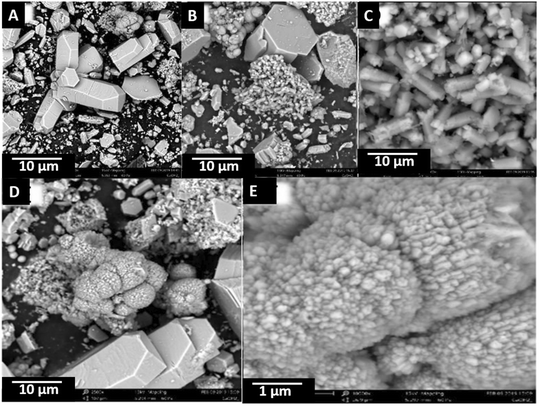 |
| Fig. 13 SEM imaging reveals that Ca(OH)2 crystallizes into three length scales. The largest crystallites of Ca(OH)2 have dimensions in the tens of micrometers (A), while smaller Ca(OH)2 crystallites form aggregates (B). Ca(OH)2 with micrometer dimensions is also present (C), as well as Ca(OH)2 with rounded nodule morphology (D). Additionally, sub-micrometer-scale crystallites are visible (E).6 | |
To determine the reactor's coulombic efficiency in relation to the stoichiometric limit, the authors carried out a variety of experiments. For each experiment, a fresh electrolyte containing 1 M of either NaNO3 or NaClO4 and the same starting quantity of CaCO3 powder was used in an H-cell reactor. To determine the quantity of CaCO3 that was lost due to chemical dissolution after operating the reactor at potentiostatic conditions (3.5 V) for periods ranging from one to fourteen hours, the cup containing CaCO3 was extracted from the reactor, dried, and weighed. Fig. 14A displays the findings from 13 experiments, where the number of moles of dissolved CaCO3 is plotted against the coulombs travelled (upper abscissa), which are calculated by integrating the current during the experiment, as well as the comparable H2 gas (lower abscissa), calculated under the assumption that the cell current and the rate of electrolysis are the same (i.e., no side reactions).
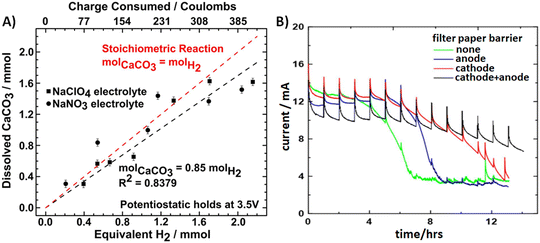 |
| Fig. 14 A) The Coulombic efficiency of the decarbonation reactor is shown by the black dashed line, which is the result of the least-squares fit to the data and corresponds to an efficiency of around 85%. On the other hand, the red dashed line represents the stoichiometric process that offers maximum conversion efficiency on a charge basis. B) Shows current vs. time for decarbonation cells held at 3.5 V, with a short open circuit hold every 1 h.6 | |
According to the stoichiometric reaction shown by the red dashed line in Fig. 14A, during the oxygen-evolution reaction two protons are generated at the anode and one CO32− (carbonate ion) was protonated. The error bars for every data point show the overall weighing error, depending on how precisely the scale was calibrated. When all of the data points are fitted using least-squares methods, the calculations show that the ratio of chemical to electrolysis reactions rates is 0.85, with a maximum value of 1. This shows that even with a laboratory-scale reactor that is not optimised, a high coulombic efficiency is still achievable. Based on the experiments presented in Fig. 14B, it can be inferred that passivating the cathode using Ca(OH)2 throughout extended reactor run times can be effective, as the results indicate a decrease in efficiency for both electrolytes at longer run times. They discovered that a few data points were over the line representing theoretical maximum efficiency. They believed that some unintentional CaCO3 loss caused this divergence while removing the CaCO3-containing cup from the reactor.
Zhang et al.9 reported the synthesis of Ca(OH)2 at the rate of 486 mg h−1 with an operating voltage of 2.9 V and a current density of 100 mA cm−2 with a current efficiency of about 100%, generating clean streams of O2, CO2, and H2 at the cathode, chemical, and anode compartments, respectively, as shown in Fig. 15. The results of the experiment demonstrated that the electrolyzer performance measures in the hypothetical future are realistic. As a result, it would seem that the efficiency of the electrolyzer is not the limiting factor for the economic viability of electrochemical cement manufacturing, which is instead reliant on factors like capital prices, energy accessibility, and pollution regulation. This analysis suggests that it may be advantageous to place this technology close to distributed renewable energy hubs where there are opportunities for integration with other new electrochemical technologies, access to inexpensive renewable electricity, networks for transporting H2, O2, and CO2, and access to distributed renewable energy hubs. The CO2(g) emissions related to cement clinker production can be reduced by 75%, thanks to these qualities taken as a whole. Their investigation demonstrated that, under certain market conditions, electrochemical cement production may achieve cost parity with conventional thermal processes. The system has the following reactions at anode (eqn (15)), chemical (eqn (16)), and cathode (eqn (17) and (18)):
| 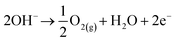 | (15) |
| CaCO3(s) + 2H+ ⇌ Ca2+(aq) + CO2(g) + H2O | (16) |
| 2H2O + 2e− → H2 + 2OH− | (17) |
| Ca2+ + 2OH− → Ca(OH)2 | (18) |
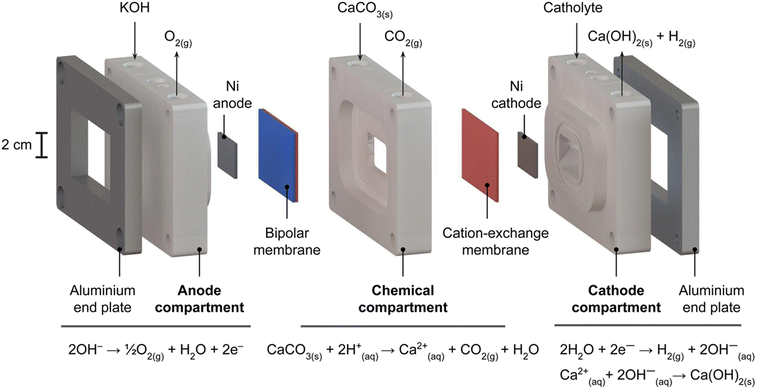 |
| Fig. 15 A device for the electrochemical synthesis of the precursors to cement clinker. A larger view of the anode, chemical, and cathode compartments in the flow electrolyzer described in this article. The cation-exchange membrane separates the chemical and cathode compartments, whereas a bipolar membrane divides the anode and chemical compartments. Both the anode and the cathode were made of nickel foam. The system's compartments' balanced reactions are displayed.9 | |
According to the authors, electrochemical cement production can attain cost parity with current thermal processes with a carbon tax of $85 per ton of CO2 emissions. This is based on an initial analysis of the running costs based on the cost of fuel, energy, and CO2 emissions (Fig. 16a). The variables utilized in this cost-parity instance (Table 7) are very similar to the electrolyzer performance measures reported in this study.9 The operational cost of producing electrochemical cement was subjected to a sensitivity analysis in order to determine the key factors influencing production costs. This study demonstrated that the key determinants of the operational costs for the manufacturing of electrochemical cement are the cost of energy, cell voltage, and current efficiency as shown in Fig. 16b.
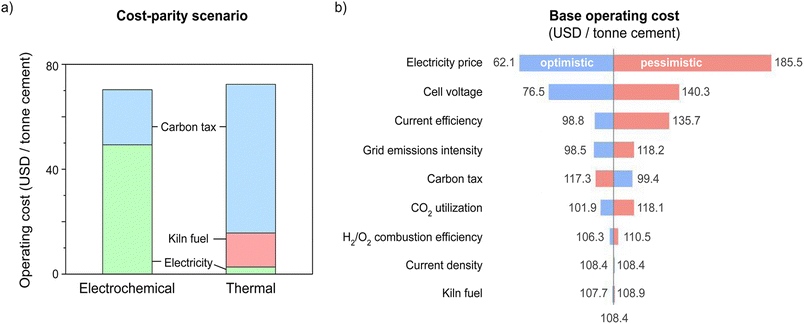 |
| Fig. 16 Operating costs for the manufacturing of cement using electrochemistry and thermal processes are analyzed and compared. Electricity, gasoline, and carbon cost are used to determine operating costs. (a) Operating cost comparison between electrochemical cement and thermal cement as detailed in Table 7 (b) analysis of the operational costs for producing electrochemical cement under a single parameter of sensitivity based on Table 8.9 | |
Table 7 The manufacturing of cement using electrochemistry and thermal processes has certain cost and life cycle parameters9
|
Pessimistic |
Base |
Optimistic |
Cost-parity scenario |
Calculated as a weighted average based on current kiln fuel use in Canada: petroleum coke (25%), coal (52%), fuel oil (5%), natural gas (18%).
For CaCO3-fed kiln (i.e., thermal pathway only).
|
Electrolyzer performance metrics |
Voltage (V) |
4 |
3 |
2 |
2.5 |
Current efficiency (%) |
70 |
90 |
100 |
100 |
CO2 utilization (%) |
50 |
80 |
100 |
100 |
Current density (Å cm−2) |
0.1 |
0.3 |
1 |
0.3 |
Energy and emissions factors |
Electricity cost ($ per kW−1 h−1) |
0.100 |
0.050 |
0.020 |
0.030 |
Grid emissions intensity (kg CO2 per kW h−1) |
0.250 |
0.175 |
0.100 |
0.150 |
Carbon tax ($ per ton CO2) |
60 |
85 |
110 |
85 |
H2/O2 combustion efficiency (%) |
70 |
80 |
90 |
88 |
Kiln fuel |
Mixa |
CH4 |
H2 |
CH4 |
Kiln thermal energy demandb (GJ per ton clinker) |
3.1 |
2.9 |
2.7 |
3.1 |
Xie et al.34 presented an electrochemical pulsed method-based integrated process for producing cement that transforms calcium carbonate (CaCO3) into calcium hydroxide (Ca(OH)2) and beneficial carbonaceous substances. The in situ investigation proved a proof-of-concept for cement synthesis without CO2 emissions by converting the CO2 produced during the CaCO3 dissolution into valuable carbonaceous compounds. The electrochemical conversion of CaCO3 into Ca(OH)2 and valuable carbonaceous compounds combines the chemical breakdown of CaCO3 with electrochemical water splitting and CO2 reduction reactions (Fig. 17A–C). First, as shown in Fig. 17C, a positive current is provided at electrode I in the left chamber (L), which is process 1. As a result, Fig. 17D shows that the OER and HER took place in the left (L) and right (R) chambers, respectively. Eqn (19)–(22) served as a representation of the specific processes. Note that NaClO4 is used as the electrolyte in this instance, creating a neutral environment. Eqn (19) and (20) state that chamber L produces H+ while chamber R produces OH−.
| 2H2O → O2 + 4H+ + 4e− | (19) |
| 2H2O + 4e− → 2H2 + 2OH− | (20) |
The H
+ produced in chamber L interacted with the CaCO
3 to yield CO
2 and Ca
2+. The produced CO
2 could be transformed into beneficial carbonaceous compounds, and the Ca
2+ migrated to the chamber's R side and interacted with OH
− to produce Ca(OH)
2, as illustrated in
eqn (19) and
(20) | CaCO3 + 2H+ → Ca2+ + CO2 + H2O | (21) |
| Ca2+ + 2OH− → Ca(OH)2 | (22) |
Then, as shown in
eqn (22) and
(24) below, the current is shifted to negative on electrode I to start process 2 (
Fig. 17C and E), where the OER process takes place in chamber R and the CO
2 reduction process takes place in chamber L.
| 2H2O + CO2 + me− → CxHyOy + OH− | (23) |
| 2H2O → O2 + 4H+ + 4e− | (24) |
The entire reaction in the two-chamber can be represented by
eqn (25) by adding processes 1 and 2 together.
| mH2O + xCaCO3 → xCa(OH)2 + nH2 + O2 + CxHyOy | (25) |
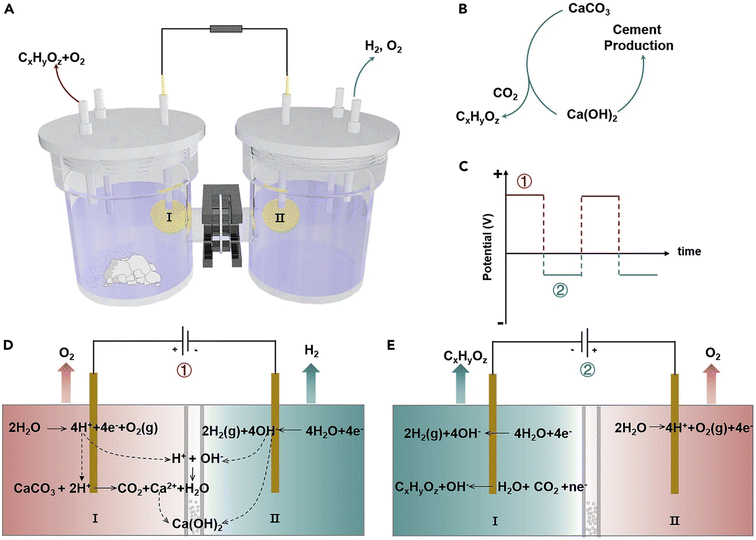 |
| Fig. 17 (A) Schematic representation of the electrochemical conversion of CaCO3 into Ca(OH)2 and useful carbonaceous compounds. (B) The process by which CaCO3 is transformed into Ca(OH)2 and useful carbonaceous compounds. (C) Schematic for the electrochemical process using pulsed chronopotentiometry. (D) The process by which CaCO3 is converted into Ca(OH)2 and CO2. (E) The procedure through which carbon dioxide is converted into useful carbonaceous compounds.34 | |
According to the authors, the procedure may be done regularly to manufacture Ca(OH)2 and worthwhile carbonaceous compounds. If the pulse duration is long enough, CaCO3 can be continuously converted into Ca(OH)2. Since the neutralization of the OH− and H+ in the system uses energy, it is crucial to optimize the generation and consumption of OH− and H+ by modifying the pulse duration.
In contrast to their previously published OER electrolyzer (>4 V), Mowbray et al.35 presented a novel cement electrolyzer that functioned at a much lower voltage (<2 V).9 The use of a CEM to transfer protons generated by the anodic HOR to the chemical compartment for CaCO3 breakdown is the distinctive characteristic of the novel electrolyzer, or “HOR electrolyzer” (Fig. 18a). A thermodynamically beneficial method of getting protons from the anode for reactions with CaCO3 is provided by the new electrolyzer design (Fig. 18b). In actuality, the HOR electrolyzer required a voltage of 4.2 V to operate at the same current density as the OER electrolyzer (Fig. 18b), but the OER electrolyzer only required a complete cell voltage (Vcell) of 1.77 V at 100 mA cm−2. Additionally, the HOR electrolyzer's total membrane and anode overpotential was 0.11 V at 100 mA cm−2, a notable decrease from the 1.14 V observed for the OER electrolyzer (Fig. 18b). The drop in cell voltage with the HOR electrolyzer design, according to the scientists, is a significant improvement over their prior findings.9
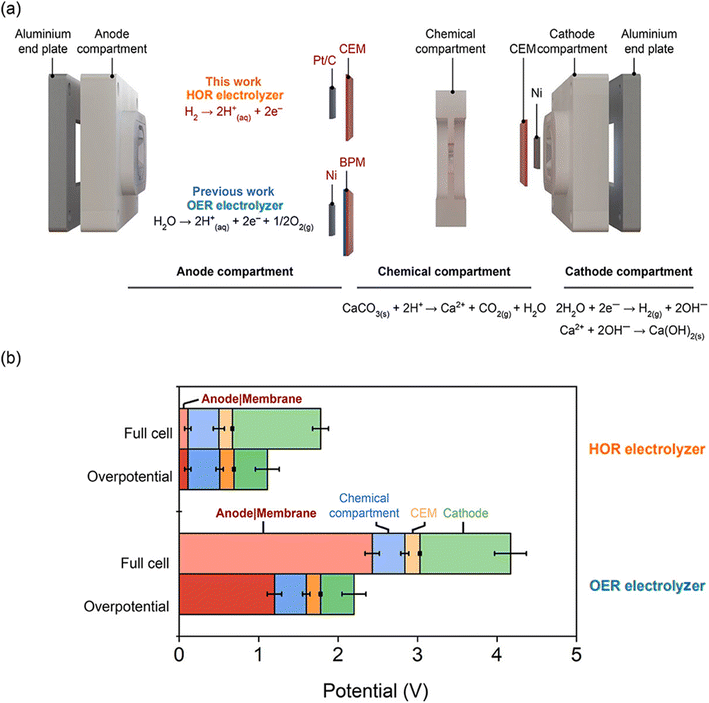 |
| Fig. 18 For the manufacture of clinker precursors, a comparison of HOR and OER electrolyzers is made. (a) Anode, chemical, and cathode chambers are shown in further detail in the cement electrolyzer architecture. (b) The difference in voltage across each element for the two electrolyzer setups. To compare the voltage related to proton production, the voltage across the anode and adjacent membrane are shown as a single component (labelled “Anode Membrane”).35 | |
Although the HOR electrolyzer uses less energy when the H2(g) byproduct is used, cement manufacturing produces more emissions as a result. The HOR pathway has an emission intensity (491 kg CO2 per tcement) that is about 10% greater than the OER pathway (520 kg CO2 per tcement). The authors claim that the decreased emissions intensity of the OER pathway is due to the use of H2 as a carbon-neutral kiln fuel. Hence, the generation of electricity is the primary source of emissions along this pathway (Fig. 19). The HOR pathway's emissions intensity comprises emissions from both the production of electricity and the burning of natural gas, which adds 219 kg CO2 per tcement. Both the production of electricity and the combustion of natural gas, which adds 219 kg CO2 per tcement to the HOR pathway's emissions intensity, are included. Therefore, when the model incorporates the price and emissions intensity of solar electricity generation, the emissions intensities of the HOR and OER routes are reduced to 291 kg CO2 per tcement and 149 kg CO2 per tcement, respectively (Table 8 and Fig. 19b).36–38 The carbon intensity of cement production has been lowered by >65% when compared to the standard thermal technique (820 kg CO2 per tcement). Additionally, the HOR and OER approaches emit fewer emissions than the large-scale cement companies' established emission goals (Lafarge Holcim: 475 kg CO2 per tcement by 2030, for example) (Table 9).35
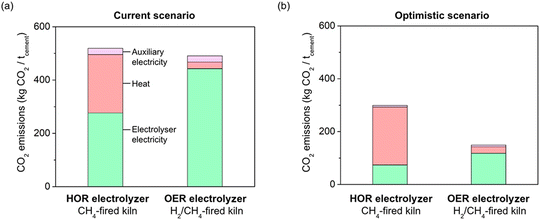 |
| Fig. 19 CO2 emissions intensity for HOR or OER electrolyzers used in electrochemical cement manufacture. In the HOR route, the Ca(OH)2-fed kiln is heated with natural gas (CH4) while the H2 stream produced at the cathode is recirculated to the anode. In the OER route, the Ca(OH)2-fed kiln is heated by the combustion of the H2 stream produced at the cathode and the O2 stream produced at the anode. In two alternative scenarios, emission intensities are predicted to indicate (a) present state of the market ($0.05 per kW h, 0.15 kg CO2 per kW h) and (b) a positive case with affordable solar electricity ($0.02 per kW h, 0.04 kg CO2 per kW h).35 | |
Table 8 Modified parameters in the scenarios of techno-economic analysis35
Parameter |
Current |
Optimistic |
Electricity price ($ per kW h) |
0.05 |
0.02 |
Grid emissions intensity (kg CO2 per kW h) |
0.15 |
0.04 |
Carbon price ($ per ton CO2) |
50 |
85 |
Table 9 Summary comparing the main characteristics of different routes for generating lime or cement with a focus on their environmental impact, energy requirements, and other relevant factors
Route |
Key characteristics |
Energy requirement |
CO2 emissions |
Key advantages |
Key challenges |
Thermal chemistry method (Licht et al.30) |
- Generates CaO without CO2 emission using variations in oxide solubility |
High-temperature electrolysis, energy-intensive |
Low (when using high-temperature electrolysis) |
- Low CO2 emission |
- High energy requirement |
- Electrolysis of limestone produces lime, O2, and reduced carbonate with low CO2 emission |
- High solubility of CaO in molten carbonates at high temperatures |
- Requires high temperatures for optimal performance |
- Utilizes solar energy |
Seawater electrochemical method (Badjatya et al.31) |
- Converts Mg2+ in seawater to Mg(OH)2 using membrane-free electrolyzers powered by solar and wind energy |
4.4 × 106 kJ per ton of cement |
Zero (if powered by carbon-free electricity) |
- Lower calcination temperatures |
- Higher energy requirement compared to Portland cement |
- Produces MgO, a low-carbon alternative to Portland cement |
- No direct CO2 emissions |
- Limited use in reinforced concrete due to lower alkalinity |
- Operates at lower calcination temperatures (700–1000 °C) |
- Uses renewable energy sources |
Electrochemical decarbonization of CaCO3 (Ellis et al.6) |
- Converts CaCO3 to Ca(OH)2 using an electrochemical cell |
Variable (depends on reactor setup and operation) |
Reduced by 75% for clinker production |
- Can be integrated with renewable energy |
- Requires optimization for large-scale use |
- Produces gases like CO2, H2, and O2, which can be utilized in various applications |
- Produces useful gases |
- High initial setup costs |
- High coulombic efficiency (∼85%) |
- High efficiency |
Pulsed electrochemical method (Xie et al.34) |
- Transforms CaCO3 into Ca(OH)2 and valuable carbonaceous compounds |
|
Reduced (if powered by renewable energy) |
- Produces valuable by products |
- Needs optimization for pulse duration |
- Utilizes a pulsed current for the electrochemical process |
- Continuous operation possible |
- Energy used in neutralizing OH− and H+ needs to be minimized |
- Potential for continuous operation |
- Lower voltage operation (<2 V) |
5. Electrodes and electrolytes used
In an electrochemical cell with 1 M NaClO4 with Ar saturation, Xie et al.34 used two Au electrodes. The O2 evolution process took place in the left chamber when a positive current of +30 mA is applied, whereas the hydrogen evolution reaction took place in the right chamber. As previously seen in Fig. 17, the CaCO3 decarbonation and Ca (OH)2 precipitation then occurred. In the process of reducing CO2, the current is turned negative (between −1.5 and −5 mA), and Fig. 20 displayed the applied current and related potentials. In this study, the electrochemical CO2 reduction process was mostly carried out using a range of metal catalyst electrodes, including Au, Ag, and Cu nanowire.
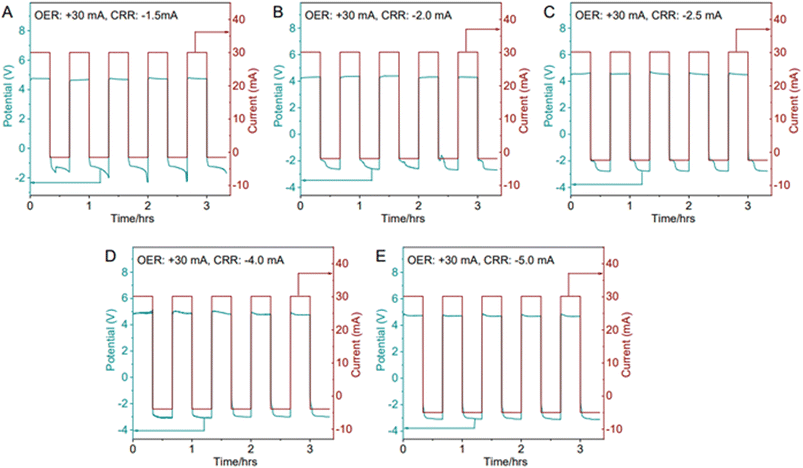 |
| Fig. 20 The potential and applied current density. (A) Positive current (ip) = +30 mA, negative current (in) = −1.5 mA, applied time of positive and negative current (tp and tn) tp = tn = 1200 s; (B) ip = +30 mA, in = −2.0 mA, tp = tn = 1200 s; (C) ip = +30 mA, in = −2.5 mA, tp = tn = 1200 s; (D) ip = +30 mA, in = −4.0 mA, tp = tn = 1200 s; (E) ip = +30 mA, in = −5.0 mA, tp = tn = 20 min.34 | |
However, two platinum electrodes were used by Ellis et al.,6 owing to their rationale that in both acidic and basic conditions, platinum exhibits a high level of catalytic activity when it comes to the production of hydrogen and oxygen. In addition, they suggested several inexpensive electrode materials that might be utilized, such as Pb, Al, or Sn should be used as the anode electrodes at pH equal 6 and stainless steel, Ni, or Cu as the cathode electrodes at pH equal 12.5. Ellis et al. utilized 1 M NaClO4 or NaNO3 as electrolytes in their system. After subjecting the system to electrolysis for a duration of 12 hours with a cell voltage of 2.5 volts (∼6 mA), a color gradient was observed. The anode was found to have an acidic solution while the cathode had an alkaline solution. The precipitation of Ca(OH)2 occurred in the cross tube when the cell voltage was raised to 9 volts to accelerate the reaction. According to their explanation, the salts they use as electrolytes are capable of dissolving and do not disintegrate when exposed to high voltage. It was also observed that, when the cell configuration was conducted without using a porous separator, Ca(OH)2 was observed to precipitate along the entire length of the cell, including on the platinum wire cathode. This precipitation process is expected to lead to passivation over time. However, by integrating a porous separator at the intersection of the chambers to limit convection, the cell was able to operate for more than 12 hours without experiencing cathode passivation, as depicted in Fig. 21.
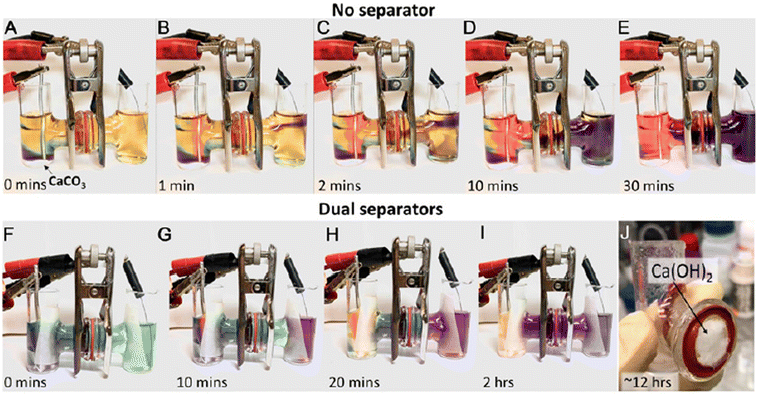 |
| Fig. 21 The decarbonation process of H-cells was monitored using platinum electrodes and 1 M NaNO3 in deionized water as the electrolyte, while pH indicator dyes provided color-coded information. The first set of images (A–E) captured the decarbonation of a cell with CaCO3 powder in the anode chamber, without any porous separator between the chambers. Images F–I depict a second decarbonation cell with porous fiber separators installed in both chambers to limit convection. The CaCO3 powder was held in a detachable cup, allowing for the monitoring of weight loss. The images demonstrate that the solutions did not separate into distinct layers. The final image (J) captured the precipitation of Ca(OH)2 in the cross-tube after 12 hours of electrolysis using a high cell voltage of 9 V, which was employed to hasten the reaction.6 | |
Zhang et al.9 used three-compartment cement electrolyser. Both anode and cathode were made of nickel foam. Cathode and chemical compartments were separated by nafion membranes of different thicknesses, and a bipolar membrane was utilized to isolate the anode from the chemical compartment. A 1 M KOH was used as an anolyte (to the anode compartment). An aqueous slurry of CaCO3 in 1 M CaCl2 was injected to the chemical compartment, and a 1 M KCl solution was used as catholyte (to the cathode compartment). Ca(OH)2 precipitation occurred in the cathode compartment. The three-compartment electrolyzer runs on a cell voltage of 2.9 V. However, the adhesion of Ca(OH)2 particles to the Nafion membrane and the porous electrode made it difficult to determine the precise amount of Ca(OH)2 produced using gravimetric methods. As a result, it was assumed that the rate of i-CO2 generation was equivalent to that of Ca(OH)2, as per eqn (16), in order to quantify the rate of Ca(OH)2 formation. The charachterization of size and morphology of Ca(OH)2 approved that all the generated Ca(OH)2 particles were lower than the standard 90 μm for raw mix in cement manufacture. A small quantity of Ca(OH)2 is generated during the electrochemical process, and it tends to accumulate at the Nafion membrane and cathode. This accumulation has a notable impact on the voltage levels. Therefore, to ensure low voltages and high energy efficiency, it is crucial to regularly remove the precipitated Ca(OH)2 from the membrane surface. Several techniques can be employed to prevent the build-up of solids and regulate their movement within the electrolyzer. These techniques include the use of porous separators, mechanical agitation, or periodic acid treatment, all of which help to maintain optimal performance and prevent operational issues caused by solid accumulation (Table 10).
Table 10 A summary table of the different electrochemical cells
Study |
Electrodes used |
Electrolytes |
Conditions |
Observations |
Xie et al.34 |
Two Au electrodes |
1 M NaClO4, Ar saturation |
+30 mA positive current (O2 evolution), −1.5 to −5 mA negative current (CO2 reduction) |
CaCO3 decarbonation and Ca(OH)2 precipitation occurred; electrochemical CO2 reduction using Au, Ag, and Cu nanowire catalysts |
Ellis et al.6 |
Two Pt electrodes |
1 M NaClO4 or NaNO3 |
Cell voltage: 2.5 V (∼6 mA) for 12 hours, 9 V for accelerated reactions |
Anode solution became acidic, cathode solution alkaline; Ca(OH)2 precipitation; porous separator used to prevent cathode passivation |
Zhang et al.9 |
Ni foam electrodes |
1 M KOH (anolyte), 1 M CaCl2 (chemical), 1 M KCl (catholyte) |
Cell voltage: 2.9 V |
Ca(OH)2 precipitation in cathode compartment; Nafion membranes used; accumulation of Ca(OH)2 affects voltage; methods needed to prevent solid build-up |
Mowbray et al.35 |
Pt/C-coated carbon paper anode, Ni foam cathode |
Humidified H2 (anode), 1 M CaCl2 (chemical), 1 M KCl (catholyte) |
Lower potentials compared to Zhang et al. |
Nafion 211 membrane used instead of bipolar membrane; same chemical and cathode compartments as Zhang et al.; differences in required energy and potential observed |
A similar cell design was conducted by Mowbray et al.35 was shown previously in Fig. 18, operates at lower potentials. The nickel foam anode was replaced with Pt/C-coated carbon paper, and the bipolar membrane was swapped out for a Nafion 211 membrane. However, the chemical and cathode compartments were kept the same. Instead of 1 M KOH used in the previous case, the anode compartment was supplied with humidified H2. The chemical compartment, on the other hand, was fed with an aqueous slurry of CaCO3 in 1 M CaCl2. The cathode compartment was fed with 1 M KCl same as the previous electrolyzer. The differences between the required energy and potential for the both electrolyzers was shown previously in Fig. 18b.
6. Conclusion and outlook
In conclusion, the electrochemical production of cement has emerged as a compelling and environmentally friendly method to address the risks associated with conventional cement production. This review has highlighted various strategies employed to promote sustainability and minimize CO2 emissions in the process. These strategies encompass the utilization of alternative fuels, alternative raw materials, and the application of electrochemical principles to cement production.
While electrochemical decarbonization of CaCO3 to Ca(OH)2 offers a pathway to produce green cement, there are notable challenges that need to be overcome. One such challenge is the effective collection of Ca(OH)2, as it tends to adhere to reactor walls, filter paper, or membranes. Advancements in system design are necessary to ensure the comprehensive retrieval of Ca(OH)2 throughout the process. Additionally, the development of efficient catalysts is crucial to enable low-voltage production, thereby enhancing commercial viability. Furthermore, the current systems are limited by low current densities due to the low solubility of CO2 in water, resulting in the release of a fraction of CO2 in gaseous form. The creation of industrially applicable flow cell designs that enhance production rates and optimize CO2 utilization is essential. Adequate provision of solid calcium carbonate to the system and efficient removal of Ca(OH)2 are also vital considerations. In summary, the electrochemical method holds great promise for sustainable cement manufacturing. However, significant challenges still remain. Therefore, it is imperative to further advance synthesis strategies and develop rational designs to meet the demands of sustainable cement production. By addressing these challenges, the way should be paved for a greener and more environmentally-friendly cement industry.
Abbreviations
PC | Portland cement |
OPC | Ordinary Portland cement |
CKD | Cement kiln dust |
C2S | Belite |
C3S | Alite |
C4A3S | Ye'elimite |
P2O5 | Phosphorus pentoxide |
HBC | High belite cement |
MHC | Moderate heat cement |
CSA | Calcium sulfo-aluminate |
C2A | Dicalcium aluminate |
C3A | Tricalcium aluminate |
M | Moles per kilogram solvent |
K | Equilibrium constant |
OER | Oxygen evolution reaction |
HOR | Hydrogen evolution reaction |
tcement | Ton of cement |
CEM | Cation exchange membrane. |
Conflicts of interest
The authors declare no competing financial interest.
References
-
Global Cement production in the net zero scenario, 2010–2030, IEA, 2024 Search PubMed.
- F. A. Rodrigues and I. Joekes, Environ. Chem. Lett., 2011, 9, 151–166 CrossRef CAS.
- M. Hanifa, R. Agarwal, U. Sharma, P. C. Thapliyal and L. P. Singh, J. CO2 Util., 2023, 67, 102292 CrossRef CAS.
- Global Cement Industry's GHG emissions. Global Efficiency Intelligence, 2022, https://www.globalefficiencyintel.com/cement-industry (Accessed May 26, 2024).
- S. J. Davis, N. S. Lewis, M. Shaner, S. Aggarwal, D. Arent, I. L. Azevedo, S. M. Benson, T. Bradley, J. Brouwer, Y. M. Chiang, C. T. M. Clack, A. Cohen, S. Doig, J. Edmonds, P. Fennell, C. B. Field, B. Hannegan, B. M. Hodge, M. I. Hoffert, E. Ingersoll, P. Jaramillo, K. S. Lackner, K. J. Mach, M. Mastrandrea, J. Ogden, P. F. Peterson, D. L. Sanchez, D. Sperling, J. Stagner, J. E. Trancik, C. J. Yang and K. Caldeira, Science, 2018, 360, eaas9793 CrossRef PubMed.
- L. D. Ellis, A. F. Badel, M. L. Chiang, R. J. Y. Park and Y. M. Chiang, Proc. Natl. Acad. Sci. U. S. A., 2020, 117, 12584–12591 CrossRef CAS PubMed.
- M. J. Orella, Y. Román-Leshkov and F. R. Brushett, Curr. Opin. Chem. Eng., 2018, 20, 159–167 CrossRef.
-
J. M. Cullen and J. M. Allwood, Steel, aluminum, and carbon: alternative strategies for meeting the 2050 carbon emission targets, Asamblea anual de Davos, 2009, pp. 2–7 Search PubMed.
- Z. Zhang, B. A. W. Mowbray, C. T. E. Parkyn, C. T. E. Waizenegger, A. S. R. Williams, E. W. Lees, S. Ren, Y. Kim, R. P. Jansonius and C. P. Berlinguette, Energy Environ. Sci., 2022, 15, 5129–5136 RSC.
- How Cement is Made, https://www.cement.org/cement-concrete/how-cement-is-made (Accessed May 26, 2024).
- D. N. Huntzinger and T. D. Eatmon, J. Cleaner Prod., 2009, 17, 668–675 CrossRef CAS.
- M. S. Imbabi, C. Carrigan and S. McKenna, Int. J. Sustainable Built Environ., 2012, 1, 194–216 CrossRef CAS.
- A. Naqi and J. Jang, Sustainability, 2019, 11, 537 CrossRef CAS.
- R. A. Feely, C. L. Sabine, K. Lee, W. Berelson, J. Kleypas, V. J. Fabry and F. J. Millero, Science, 2004, 305, 362–366 CrossRef CAS PubMed.
- G. Habert, C. Billard, P. Rossi, C. Chen and N. Roussel, Cem. Concr. Res., 2010, 40, 820–826 CrossRef CAS.
- N. Chatziaras, C. S. Psomopoulos and N. J. Themelis, Manag. Environ. Qual., 2016, 27, 178–193 CrossRef.
- M. Schneider, M. Romer, M. Tschudin and H. Bolio, Cem. Concr. Res., 2011, 41, 642–650 CrossRef CAS.
-
G. Habert, in Eco-Efficient Construction and Building Materials: Life Cycle Assessment (LCA), Eco-Labelling and Case Studies, Elsevier Inc., 2013, pp. 199–238 Search PubMed.
- T. Sui, L. Fan, Z. Wen and J. Wang, J. Civ. Eng. Archit., 2015, 9, 384–392 Search PubMed.
- E. Gartner and T. Sui, Cem. Concr. Res., 2018, 114, 27–39 CrossRef CAS.
- K. L. Scrivener, V. M. John and E. M. Gartner, Cem. Concr. Res., 2018, 114, 2–26 CrossRef CAS.
- S. A. Walling and J. L. Provis, Chem. Rev., 2016, 116, 4170–4204 CrossRef CAS PubMed.
- W. Chen, S. Elkatatny, M. Murtaza and A. A. Mahmoud, Front. Mater., 2021, 8, 754431 CrossRef.
- W. R. Eubank, J. Am. Ceram. Soc., 1951, 34, 225–229 CrossRef CAS.
- P. Maravelaki-Kalaitzaki and G. Moraitou, Cem. Concr. Res., 1999, 29, 1929–1935 CrossRef CAS.
- J. W. Phair, Green Chem., 2006, 8, 763–780 RSC.
- F. P. Glasser and L. Zhang, Cem. Concr. Res., 2001, 31, 1881–1886 CrossRef CAS.
-
What is carbon capture, usage and storage (CCUS) and what role can it play in tackling climate change?, London School of Economics and Political Science, 2023 Search PubMed.
- K. Novak Mavar, N. Gaurina-Međimurec and L. Hrnčević, Sustainability, 2021, 13, 1800 CrossRef.
- S. Licht, H. Wu, C. Hettige, B. Wang, J. Asercion, J. Lau and J. Stuart, Chem. Commun., 2012, 48, 6019–6021 RSC.
- P. Badjatya, A. H. Akca, D. V. Fraga Alvarez, B. Chang, S. Ma, X. Pang, E. Wang, Q. Van Hinsberg, D. V. Esposito and S. Kawashima, Proc. Natl. Acad. Sci. U. S. A., 2022, 119, e2114680119 CrossRef CAS PubMed.
- N. T. Dung and C. Unluer, Constr. Build. Mater., 2017, 143, 71–82 CrossRef CAS.
- V. S. S. Birchal, S. D. F. Rocha and V. S. T. Ciminelli, Miner. Eng., 2000, 13, 1629–1633 CrossRef CAS.
- Q. Xie, L. Wan, Z. Zhang and J. Luo, iScience, 2023, 26, 106015 CrossRef CAS PubMed.
- B. A. W. Mowbray, Z. B. Zhang, C. T. E. Parkyn and C. P. Berlinguette, ACS Energy Lett., 2023, 8, 1772–1778 CrossRef CAS.
- H. C. Kim, V. Fthenakis, J. K. Choi and D. E. Turney, J. Ind. Ecol., 2012, 16, S110–S121 CrossRef CAS.
- W. Cole, B. Frew, P. Gagnon, A. Reimers, J. Zuboy and R. Margolis, Energy, 2018, 155, 690–704 CrossRef.
- F. Asdrubali, G. Baldinelli, F. D'Alessandro and F. Scrucca, Renewable Sustainable Energy Rev., 2015, 42, 1113–1122 CrossRef.
Footnote |
† Equal contribution. |
|
This journal is © The Royal Society of Chemistry 2024 |