Thermally activated delayed fluorescence emitters for efficient sensitization of europium(III)†
Received
19th April 2024
, Accepted 30th May 2024
First published on 31st May 2024
Abstract
We demonstrate for the first time a unique approach to efficiently sensitize lanthanides(III) using photosensitizer ligands that show thermally activated delayed fluorescence (TADF). TADF ligands have very small singlet (S1) and triplet (T1) excited state energy splitting and S1/T1 energy levels are in optimum energy to the acceptor level of Eu(III) to enable high energy transfer efficiency. The synthesized Eu(III) coordination polymers with TADF ligands showed bright red luminescence with an outstanding sensitization efficiency of 90–94% and Φtot of 79–85% in poly(methyl methacrylate) encapsulated films. This rational approach of efficiently sensitizing lanthanides with TADF ligands demonstrates their great potential for imaging and optical communications applications.
Introduction
Luminescent materials continue to attract considerable interest owing to their widespread potential in optical devices and biomedical applications.1–5 Trivalent lanthanide(III) complexes captured extensive attention in luminescence technology given their unique photoluminescence (PL) characteristics and potential applications in displays,6 solar cells,7,8 anti-counterfeiting,9 luminescent probes for live cell imaging,10 and sensing.11 The distinctive PL features of Ln(III) complexes include high color purity (small full width at half maximum), large Stokes shift, and long-lived excited state lifetime due to the Laporte forbidden and intra-configurational nature of f–f transitions.12 However, the f–f transitions of Ln(III) exhibit very weak absorbance (molar extinction coefficient, ε < 10 M−1 cm−1), and thus direct photoexcitation of f–f transition is inefficient. This is known to be overcome by antenna sensitization with suitable organic ligands having high absorption coefficients to exploit the inherent PL features of Ln(III) complexes for various applications.12,13 Numerous classes of sensitizer ligands have been used for the complexion of Ln(III), however, the choices of ligands are very limited to obtain high energy transfer efficiency.14–18 For lanthanide complexes, the luminescence quantum efficiency is the product of sensitization efficiency (ηsens) and the intrinsic lanthanide emission quantum yield (ΦLn); (Φtot = ηsens × ΦLn = ηsens × kr/(kr + knr); kr and knr are the radiative and non-radiative decay rate constants, respectively).19,20 The high Φtot values in Ln(III) complexes were realized by introducing an asymmetric coordination environment resulting in a large kr. Anionic β-diketonates are widely used as antennae ligands owing to their stable coordination with Ln(III) cations and large polarizability resulting in large kr. Rigid neutral ancillary ligands with low vibrational quenching decrease the knr. Thus Ln(III) complexes with β-diketonate and low vibrational neutral ligands like phosphine oxides are effective for achieving near unity Φtot.20 Despite the intriguing luminescent properties and applications of lanthanide complexes, designing chelating antennae/ancillary ligands with large absorption coefficients and stable coordination to Ln(III) ions to develop highly efficient lanthanide emitters is still challenging.
Upon photoexcitation, ligands undergo intersystem crossing (ISC) from the lowest singlet excited state (S1) to the lowest triplet excited state (T1), thereby transferring their electronic energy to the Ln(III) ion. Organic ligands with a small singlet (S1)-triplet (T1) energy gap (ΔEST) and longer T1 lifetime show a high ISC yield. Further, to realize efficient ligand to Ln(III) energy transfer (ηsens) and avoid the back energy transfer, the T1 state of the ligands should lie ∼2000–3500 cm−1 above the energy-accepting states (EAS) of the Ln(III).21–24 Expanding the π-conjugation length of ligands leads to a decrease in T1 energy level, however, in many cases, the limitation arises due to the metal-to-ligand back energy transfer owing to the very small ΔE(T1-EAS).20 Recently Hasegawa and co-workers showed low energy photosensitized emission of Eu(III) complex containing stacked-coronene nanocarbon ligand with a ΔEST (3700 cm−1) and longer T1 lifetime (∼40 ms) which allows for equilibration (forward and backward energy transfer) between the T1 state of ligand and EAS state of Eu(III) that led to an emission quantum yield of 61%.25 A rational design for novel ligands with small ΔEST and an adequate ΔE(T1-EAS) is important in obtaining Ln(III) complexes with efficient energy transfer for improving the luminescence quantum yield.
Eu(III) coordination polymers with rigid multi-dimensional networks are known to show superior thermal and optical properties ideal for optical devices.26 Hasegawa and co-workers reported Eu(III) coordination polymers containing hexafluoroacetylacetonate (hfa) and thiophene-based bidentate phosphine oxide bridging ligands with their thermal stability up to ∼320 °C and a Φtot of 60% with 80% ηsens in the solid state.27 A similar Eu(III) coordination polymer containing hfa and furan-based bidentate phosphine oxide bridging ligands exhibited 64% Φtot with 88% ηsens in the solid state.28 They also reported a highly thermally stable (>300 °C) Eu(III) coordination polymer containing hfa and biphenylene-based bidentate phosphine oxide bridging ligands showing 29% Φtot with 40% ηsens in the solid state.29 Bünzil and co-workers reported Eu(III) containing hfa and bidentate carboxylic ancillary ligands with a ΦLn in the range of 77–100%, Φtot in the range of 18–51% and ηsens in the range 23–51% in the solid state.30
Our new strategy envisages that ligands with minimal ΔEST may be constructed with donor–acceptors such that the ligand molecule exhibits thermally activated delayed fluorescence (TADF) properties.31,32 TADF ligands are then coordinated with lanthanide metals to develop unique new TADF-ligand-anchored Ln(III) β-diketonate complexes. The key point is that the triplet energies of TADF ligands can be easily tuned by facile structural modifications and the ΔEST of the TADF-sensitizer is only a few hundred cm−1. Subtle molecular design by choosing antennae TADF-ligands having appropriate energy level matching induces intramolecular energy transfer from ligands to Ln(III), enabling highly efficient energy transfer (Fig. 1).
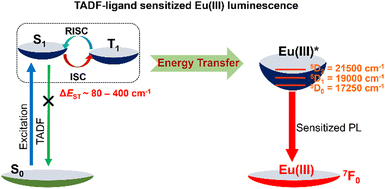 |
| Fig. 1 Strategy for the energy transfer pathway in TADF-ligand sensitized Eu(III) emission. | |
Here we report the synthesis and optical properties of three novel Eu(III) β-diketonates with neutral ancillary TADF-ligands (Scheme 1). The ligands 1L, 2L, and 3L consist of a spiro-(fluorine-9,9′-xanthane) (SFX) unit anchored with weakly electron-accepting diphenylphosphine oxides and diarylamine donors. The S1 and T1 energy levels of 1L, 2L, and 3L were fine-tuned by varying the electron-donating strength of diarylamine donors. The design, synthesis, efficient TADF properties, and their application in OLEDs of 1L, 2L and 3L have been reported by us recently.33 The presence of a diphenylphosphine oxide chelating unit endows them with the ability to coordinate with Ln(III). Promisingly, the excited (S1 and T1) state energies of TADF-ligands show optimum energy matching with Eu(III) energy-accepting states. Further, the matching excited state energy levels of theonyltrifluoroacetonate (tta) and the TADF ligands, can accelerate the simultaneous transfer of energy to the Eu(III). Novel Eu(III) coordination polymers 1, 2, and 3 containing tta and SFX-based TADF ligands (1L, 2L, and 3L) were designed, synthesized, and studied for their PL characteristics as a proof of concept of our strategy. The present newly developed Eu-TADF coordination polymers exhibit Eu(III) centered bright red luminescence with a dominant narrow emission band at 611 nm in solution, solid state, and poly-(methyl methacrylate) (PMMA) films. High intrinsic emission quantum yield (∼90%) and sensitization efficiency of ∼94% were achieved in the TADF ligand-sensitized Eu(III) coordination polymers in PMMA encapsulated films.
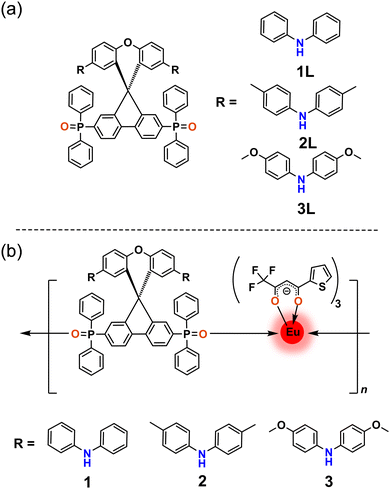 |
| Scheme 1 (a) Chemical structures of TADF-ligands. (b) Chemical structures of Eu(III) coordination polymers with tta and TADF-ligands. | |
Experimental section
General methods
All the chemicals were purchased from commercial suppliers (Sigma Aldrich, Fisher Scientific, or Fluorochem, UK) and used as received unless otherwise mentioned. The reactions were performed using standard Schlenk-line techniques under the N2 atmosphere. NMR spectroscopy was carried out using Bruker Pro500 spectrometers. 19F and 31P NMR spectra were all recorded in deuterated benzene and chemical shifts are reported in parts per million. Mass spectra were recorded with Xevo QTOF (Waters) high resolution, accurate mass tandem mass spectrometer equipped with Atmospheric Solids Analysis Probe (ASAP) and Bruker MicroToF 2. All spectra were recorded using electrospray (ESI) ionization. IR spectra were measured at room temperature on a PerkinElmer Spectrum 65 FT-IR spectrometer in a 4000–450 cm−1 spectral range. Thermo-Gravimetric Analysis (TGA) curves of compounds were collected in a NETZSCH STA 449 F1 apparatus at a heating rate of 5 °C min−1 under a nitrogen atmosphere.
Synthesis
TADF ligands 1L, 2L and 3L33 and Eutta3.2H2O18 were synthesized according to our recently reported procedure. A mixture of Eutta3.2H2O (0.05 mmol) and appropriate TADF ligands (0.05 mmol), 1L for 1, 2L for 2, and 3L for 3 in HPLC grade acetone was stirred for 2 hours at room temperature and the solvent was evaporated and the compound was dried under vacuum. The resultant residue was recrystallized from a 1
:
1 mixture of acetone and pentane, to get the pure compounds as pale yellow (1), yellow (2), and dark yellow (3) powders in ∼95% yield. The complex was characterized by 31P and 19F NMR spectroscopy.
Coordination polymer 1
19F NMR (500 MHz, C6D6-d6): δ (ppm) −79.7; 31P NMR (500 MHz, C6D6-d6): δ (ppm) 76.5. ESI-MS [M+Na]+ calculated (C97H64Eu1F9N2O9S3Na1) 1905.2209; found 1905.2223.
Coordination polymer 2
19F NMR (500 MHz, C6D6-d6): δ (ppm) −79.5; 31P NMR (500 MHz, C6D6-d6): δ (ppm) −76.3. ESI-MS [M+H]+ calculated (C101H73Eu1F9N2O9P2S3) 1939.3016; found 1939.3030.
Coordination polymer 3
19F NMR (500 MHz, C6D6-d6): δ (ppm) −79.5; 31P NMR (500 MHz, C6D6-d6): δ (ppm) −75.9. ESI-MS [M+H]+ calculated (C101H73Eu1F9N2O13P2S3) 2003.2813; found 2003.2827.
Photophysical characterization
Optically dilute solutions of compounds were prepared in HPLC grade solvent for UV-Vis absorption and emission analysis. UV-Vis absorption spectra of solution samples were recorded on a Shimadzu UV-1800 double beam spectrophotometer, at room temperature. UV-Vis absorption spectra measurements in diffuse reflectance mode were made on powder samples using a JASCO V-670 spectrophotometer with an integrating sphere attachment. Non-absorbing BaSO4 was used as the reference and the powders were mixed with BaSO4 and were used for the measurements. UV-Vis absorption spectra of PMMA films were also measured on JASCO V-670 spectrophotometer. Steady-state emission and time-resolved emission spectra were recorded with a Fluoromax-P spectrofluorimeter (Horiba–Jobin–Yvon) at 298 K. The concentration of the lanthanide coordination polymers was such as to give an absorbance of around 0.1 at the excitation wavelength, both in solution and polymer matrix. Excitation and emission spectra were measured using the spectrofluorimeter with a continuous xenon lamp excitation source, in photon counting mode. Spectra were corrected for variations in excitation intensity and the response of the detector. Lifetimes were measured using a pulsed xenon lamp source, with pulse width <50 μs, and time-gated detection. Total luminescence quantum yields (Φtot) of complexes were determined by comparative method solution. Quinine sulphate is used as the standard dye for samples solution state, whose quantum yield (Φr) in 1N H2SO4 was determined to be 54.6% using the absolute method.34 Following equation (eqn (1)) was used for the calculation of quantum yield | 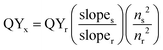 | (1) |
Where s and r denote the compound and reference samples, slope is the calculated from the graph of integrated emission versus absorbance and n is the refractive index of the solution.
Absolute luminescence quantum yield of solid powders and PMMA encapsulated films were measured using Quanta–Phi (Horiba–Jobin–Yvon) integrating sphere. PMMA films of Eu(III) coordination polymers were prepared by mixing the Eu polymer (2 wt%) and PMMA in toluene followed by spin-casting on a quartz substrate.
Total luminescence quantum yields (Φtot) of complexes is the product of ligand sensitization efficiency (ηsens) and the intrinsic luminescence quantum efficiency of the Ln(III) ion (ΦLn).19ΦLn, could not be determined experimentally because of the very low intensity of f–f transition, but it can be calculated from the ratio of the lanthanide luminescence lifetime, τLn, and its pure radiative lifetime, τR. The latter have been calculated from eqn (3), according to method described by Werts et al.
| 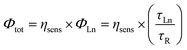 | (2) |
| 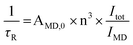 | (3) |
Where
η represents the refractive index (an average refractive index of 1.5 was used for solid state measurements) of the medium.
AMD,0 is the spontaneous emission probability for the
5D
0/
7F
1 transition
in vacuo (14.65 s
−1), and
Itot/
IMD signifies the ratio of the total integrated intensity of the corrected Eu
3+ emission spectrum to the integrated intensity of the magnetic dipole
5D
0/
7F
1 transition.
Further the radiative (kr) and non-radiative (knr) decay rate constant were calculated by the following equations (eqn (4) and eqn (5))20
|  | (4) |
|  | (5) |
Results and discussion
Synthesis and characterization
The Eu(III) complexes with TADF ligands were synthesized by a modified literature procedure,18 that involves mixing equimolar amounts of Eutta3.2H2O complex with the corresponding TADF ligands (1L for 1, 2L for 2, and 3L for 3) in acetone solution under ambient conditions (Scheme 2). The Eu(III) complexes are stable under ambient conditions and soluble in common organic solvents like toluene, DCM, DCE, CHCl3, and THF. Complexes were characterized by 31P, 19F NMR, ESI-MS, and FT-IR (Fig. S1–S12, ESI†). A shift in the 31P NMR resonance from ∼28 ppm of the TADF ligands33 to ∼ −80 ppm in complexes confirms the coordination of P
O with Eu(III), in line with related literature reports35 (Table S1, ESI†). Further, a shift in the 19F NMR in complexes compared to the free tta ligand also confirms the coordination of tta molecules with Eu(III). ESI-MS showed the molecular ion peak corresponding to the repeating unit in the coordination polymeric structures 1, 2 and 3, similar to other Eu(III) coordination polymers reported in literature.27,28 IR stretching frequencies for P
O were shifted from 1190 cm−1 in ligands to 1175 cm−1 in complexes and for C
O was shifted from 1645 cm−1 in tta to 1610 cm−1 in Eu-coordination polymers, clearly pointing to the presence of TADF ligands and tta in the coordination sphere (Fig. S10–S12, ESI†). Several attempts to recrystallize the Eu(III) coordination polymers were unsuccessful. Coordination polymers 1–3 are thermally stable up to 270 °C (Fig. S13, ESI†).
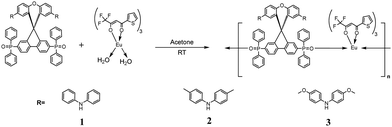 |
| Scheme 2 Synthesis of Eu(III) coordination polymers 1, 2 and 3. | |
Optical properties
The UV-Vis absorption and PL properties of Eu(III) coordination polymers 1–3 were studied in a toluene solution at room temperature. Coordination polymers 1–3 exhibit absorption profiles including strong peaks at 295, 322, and 340 nm (Fig. S15, ESI†). The ligand tta shows a strong broad absorption around 340 nm. The TADF-ligands 1L, 2L, and 3L exhibited a series of strong absorption bands at 290, 300, and 320 nm. The absorption spectra of Eu(III) coordination polymers are a combined effect of both tta and TADF ligands. The ligand tta shows emission in the region 400–500 nm with maxima around ∼450 nm36 while the TADF ligands show emission in the region 400–700 nm with emission maxima centred around 1L (∼490 nm), 2L (∼492 nm) and 3L (∼514 nm).33 Since both tta and TADF-ligands show absorption in the same spectral region, both can sensitize Eu(III) simultaneously, upon photoexcitation.
Steady-state PL spectra of polymers in toluene showed the characteristic emission profile of Eu(III),37 with narrow emission bands (FWHM∼10 nm) corresponding to the 5D0 to 7FJ (J = 0, 1, 2, 3 and 4) transitions observed around ∼578, ∼592, ∼611, ∼650 and ∼697 nm (Fig. 2a and S16, λex = 340 nm). The very intense, narrow signal around ∼611 nm corresponds to the hypersensitive 5D0 to 7F2 transition and is responsible for the bright red emission colour. The absence of ligand-centred broad emissions (Fig. S14, ESI†) in the PL spectra of complexes, indicates an efficient sensitization of Eu(III) by tta and TADF ligands. The excitation spectral profile collected by monitoring the f–f transitions of complexes matches the absorption spectral profile, evidencing that the excited state energy transfer occurs from TADF and tta ligands to Eu(III). The photosensitization parameters of 1–3 containing TADF ligands are summarized in Table 1 and details about the determination of the optical parameters are described in experimental section. The calculated ΦLn of the Eu(III) coordination polymers 1–3 are 50, 59, and 45%, and the Φtot values are determined to be 39, 42, and 29%, respectively in the toluene solution. The excited state energy transfer efficiency (ηsens) calculated from the ratio of Φtot and ΦLn are 78, 72, and 64%, respectively for 1, 2, and 3. Time-resolved PL (TRPL) decay of 1–3 follows a first-order kinetics with lifetimes in the range of 0.51–0.57 ms (Fig. 2b and Fig. S17, ESI†). The nearly identical kr (0.9 × 103 – 1.0 × 103) and knr (7.2 × 102 – 11.0 × 102) values imply the presence of active non-radiative deactivation pathways that contribute to reduced ΦLn and Φtot in solution state.
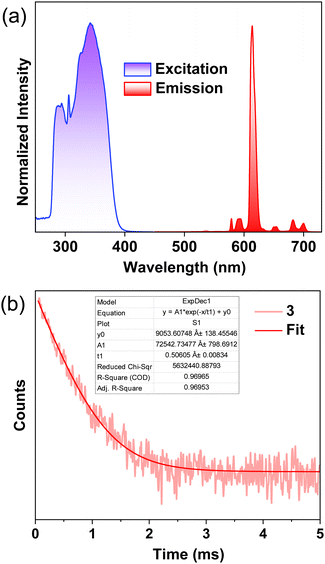 |
| Fig. 2 (a) Excitation and PL spectra and (b) TRPL of 3 in toluene (λex = 340 nm and λem= 611 nm). | |
Table 1 Photophysical properties of 1–3 (λex = 340 nm and λem = 611 nm)
|
τ
Ln
(ms) |
τ
R
(ms) |
Φ
Ln
(%) |
Φ
tot
(%) |
η
sen
(%) |
k
r × 103f (s−1) |
k
nr × 103g (s−1) |
Lanthanide luminescence lifetime obtained from TRPL spectra.
Radiative lifetime τR.
The intrinsic luminescence quantum yield ΦLn = τLn/τR.
Total luminescence quantum yields (Φtot).
Sensitization efficiency ηsen = Φtot/ΦLn.
Radiative decay rate constant kr = 1/τR.
Non-radiative decay rate constant, knr = (τR – τLn)/τR τLn.19,20
|
Toluene solution |
1
|
0.52 |
1.03 |
50 |
39 |
78 |
0.98 |
9.7 |
2
|
0.57 |
0.98 |
59 |
42 |
72 |
1.00 |
7.2 |
3
|
0.51 |
1.11 |
45 |
29 |
64 |
0.90 |
11.0 |
Solid powder samples |
1
|
0.53 |
0.64 |
83 |
56 |
67 |
1.6 |
3.2 |
2
|
0.48 |
0.64 |
74 |
59 |
80 |
1.6 |
5.4 |
3
|
0.66 |
0.96 |
68 |
57 |
84 |
1.0 |
4.5 |
PMMA encapsulated films |
1
|
0.62 |
0.68 |
90 |
85 |
94 |
1.5 |
1.4 |
2
|
0.62 |
0.70 |
88 |
79 |
90 |
1.4 |
1.8 |
3
|
0.63 |
0.69 |
91 |
84 |
92 |
1.4 |
1.4 |
The optical properties of the coordination polymers were also measured for solid powder samples. The absorption and excitation spectra of Eu(III) polymers in the solid state showed a broad absorption band in the region of 280–450 nm (Fig. 3a and Fig. S18, S19, ESI†). Coordination polymers 1–3 showed characteristic Eu(III) centred luminescence similar to that of the solution state. The absence of ligand-centred emission further supports the efficient sensitization of Eu(III) by TADF ligands. A mono-exponential emission decay profile with lifetimes in the range of 0.48–0.66 ms was noted in the solid state (Fig. 3b and Fig. S20, ESI†). Coordination polymers 1, 2, and 3 showed high ΦLn values of 83, 74, and 68%, and Φtot values are 56, 59, and 57% respectively in the solid state (Fig. S21–23, ESI†). The pure radiative lifetime, τR in compound 3 (0.96 ms) is longer compared to 1 (0.64 ms) and 2 (0.64 ms). This could be due to differences in solid state packing; compound 3 may have a less symmetric structure in the solid state and thus an increased rate of radiative f–f transition. Significantly enhanced ηsens of ∼84% for 3 obtained in the solid state is attributed to the efficient excited state energy transfer accelerated by TADF sensitization (Table 1). Notably, higher values of ΦLn in the solid state compared to the solution state could be due to the suppression of molecular motions, further substantiated by the low knr values (3.2 × 102 –5.4 × 102).
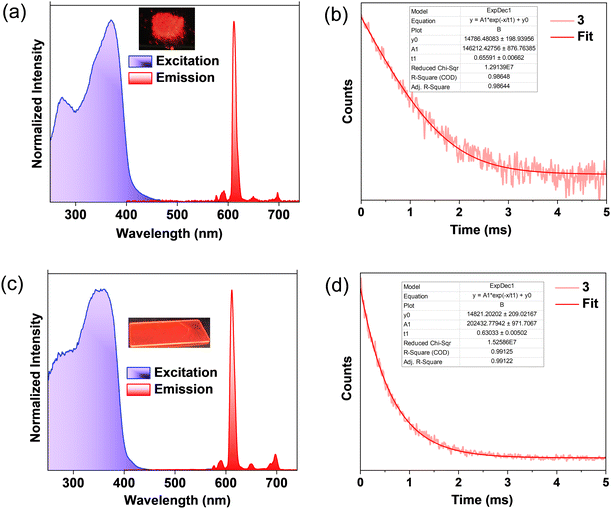 |
| Fig. 3 (a) Excitation and PL spectra of solid powders of 3 and (b) TRPL of solid powders of 3, (c) excitation and PL spectra of 3 in PMMA encapsulated films and (d) TRPL of 3 in PMMA encapsulated films (λex = 340 nm and λem = 611 nm). The inset shows the images of compound 3 under UV illumination (λex = 365 nm). | |
The PL properties were further measured in PMMA-encapsulated films of polymers 1–3. The UV-Vis absorption and excitation spectra of 2 wt% PMMA films showed absorption in the region 250–450 nm (Fig. 3c and Fig. S24, S25, ESI†). The PL spectra of 1–3 showed characteristic Eu(III) centered emission and no ligand-centered emissions were observed, further confirming the efficient excited state energy transfer from tta and TADF ligands to Eu(III) upon photoexcitation. The TRPL decay of 1–3 showed a first-order kinetic with a lifetime of 0.62 ms for 1 and 2 and 0.63 ms for 3 (Fig. 3d and Fig. S26, ESI†). In sharp contrast to solid samples, the ΦLn (∼90%) values for 2wt% PMMA films of 1–3 are observed to be higher than for Eu(III) coordination polymers reported.28–30,38–40 This strategic molecular design further endowed the complexes with a very high Φtot (∼79–85%) due to the remarkably high ηsens (∼90–94%). Reduced knr (1.4–1.8 × 102) values in films compared to the solution and solid state could be due to suppressed vibrational relaxation and reduced concentration quenching in the polymer matrix that results in high ΦLn. This high Φtot and ηsens of Eu coordination polymers with TADF ligands point to the potential of this unique molecular design strategy for photonic application.
In the excitation spectra of complexes in PMMA-encapsulated films, a clear tail extends into the visible region, which prompted us to investigate their PL properties under visible excitations. The characteristic Eu(III) centered red emission at 611 nm was observed for a range of excitation wavelength from 300–450 nm for 1–3 (Fig. S30, ESI†). In the visible excitation at 400 nm, the TADF sensitized Eu(III) coordination polymers 1, 2, and 3 showed a Φtot and ΦLn in the range of 36–39% and 88–91% in PMMA films (Table S2, ESI†). The sensitization efficiency was determined to be in the range of 41–43%. Visible light-sensitized luminescent lanthanide complexes are always demanding for developing less harmful bio-labelling or imaging agents for life sciences.1,10
To get more insight into the mechanistic aspects of energy transfer process in these complexes, the excited states (S1 and T1) energy level alignment of the ligands, Eu(III) and the possible energy transfer pathways are depicted in Fig. 4 and Fig. S36, S37 (ESI†). The excited state energies of different fragments are used for prediction of the possible sensitization mechanism, while assuming that the absorption is ligand centred and emission is Eu(III) localized. The S1 and T1 energy levels of TADF-ligands were experimentally determined from the onset of prompt and delayed emission spectra. The excited state energies of the TADF ligands are, 1L, S1 (22
987 cm−1) and T1 (22
584 cm−1), 2L, S1 (22
584 cm−1) and T1 (22
422 cm−1) and 3L, S1 (22
180 cm−1) and T1 (22
100 cm−1), above their ground (S0) state. All these ligands showed very small ΔEST of ∼400–80 cm−1 (0.05–0.01 eV) owing to the near orthogonal geometry of the donor–acceptor structures, the unique characteristics of TADF molecules.31 The S1 and T1 state energies of the tta ligand are 26
000 cm−1 and 21
280 cm−1, respectively.21 The excited state energy accepting levels of Eu(III), 5D2, 5D1, and 5D0 lie at 21
500 cm−1, 19
000 cm−1, and 17
250 cm−1, respectively above the 7F0 electronic ground level.22 The S1 and T1 excited states of the ligands have optimum energy to match the energy-accepting states of Eu(III) indicating that TADF ligands can act as efficient photosensitizers for Eu(III). The adequate energy gap between the TADF ligand (1L, 2L, and 3L) excited states and the Eu(III) excited state promotes the forward energy transfer to the metal and may prevent the backward energy transfer. For instance, as depicted in Fig. 4, the nearly isoenergic S1 (22
180 cm−1) and T1 (22
100 cm−1) levels of TADF-ligand 3L is ∼3100 cm−1 above the 5D1 state of Eu(III), this adequate energy difference enhances the rate of forward energy transfer, upon photoexcitation in 3. The T1 energy level of tta lies ∼2280 cm−1 above the 5D1 state of Eu(III), another forward energy transfer path to Eu(III) upon photoexcitation. Further, S1 and T1 energy levels of 3L lie in between the S1 and T1 energy levels of tta and thus there may be an additional energy transfer from the T1 excited state of 3L to the T1 state of tta and to the Eu(III) excited state, that results in an enhanced rate of excited state energy transfer to Eu and thus high Φtot.27,28 The nearly isoenergic S1 and T1 energy levels of 1L (S1, 22
987 cm−1; T1, 22
584 cm−1) and 2L (S1, 22
584 cm−1; T1, 22
422 cm−1) lie 3580 cm−1 and 3400 cm−1, respectively above the 5D1 state of Eu(III) in coordination polymers 1 and 2. Adequate energy difference enhances the rate of forward energy transfer, upon photoexcitation (Fig. S36 and S37, ESI†). There may be also an additional energy transfer from the T1 excited state of 1L and 2L to the T1 state of tta and to the Eu(III) excited state, as S1 and T1 energy levels of 1L and 2L lies in between the S1 and T1 energy levels of tta. Based on the energy level diagram it is clear that the TADF ligands can serve as antennae as well as ancillary ligand for sensitization of Eu(III) (Fig. 4 and Fig. S36, S37, ESI†).
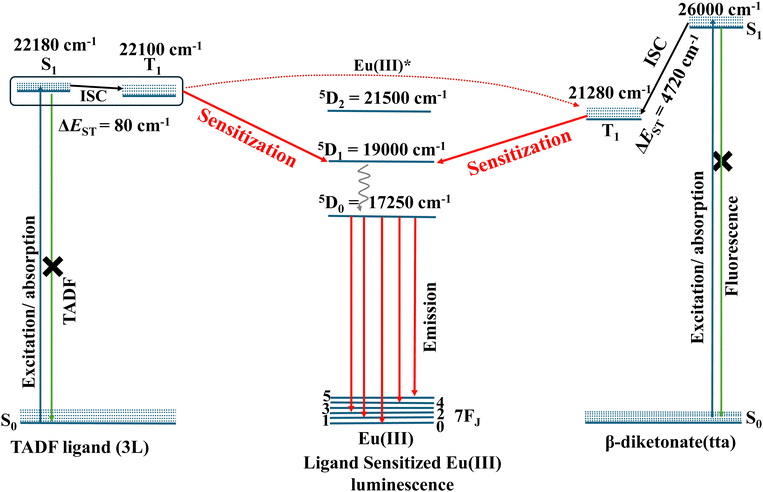 |
| Fig. 4 The mechanistic energy transfer pathways in the tta and TADF-ligand (3L) sensitized Eu(III) luminescence of coordination polymer 3 (energy levels are not to the scale). | |
Conclusions
We have demonstrated for the first time that TADF-molecules are efficient photosensitizers for Eu(III). The Eu(III) coordination polymers 1, 2, and 3 with TADF ligands showed high luminescence quantum yield (79–85%) and efficient sensitization (90–94%) in PMMA films. The appropriate energy matching criteria of excited state energy levels of TADF ligands and Eu(III) were found to enhance ΦLn and ηsens. Remarkably, efficient photosensitization (75–87%) was realized in the visible light excitation with TADF photosensitizer ligands. This novel approach will also apply to organo-lanthanide emitters in all fields such that our concept has the potential for a broad new sensitizer-ligand paradigm across related fields such as imaging or optical communications.
Conflicts of interest
There are no conflicts to declare.
Acknowledgements
This project is funded by European Union's Horizon 2020 research and innovation programme under the Marie Sklodowska Curie rant agreement H2020-MSCA-IF-2020-101025621 (TADF-LDS). We thank Prof. Anita C. Jones and Dr Iain Wright, School of Chemistry, The University of Edinburgh for support with photoluminescence and Integrating sphere facilities.
Notes and references
- S. V. Eliseeva and J.-C. G. Bünzli, Lanthanide luminescence for functional materials and bio-sciences, Chem. Soc. Rev., 2010, 39, 189–227 RSC.
- J. M. Ha, S. H. Hur, A. Pathak, J.-E. Jeong and H. Y. Woo, Recent advances in organic luminescent materials with narrowband emission, NPG Asia Mater., 2021, 13, 53 CrossRef CAS.
- Y. Im, M. Kim, Y. J. Cho, J.-A. Seo, K. S. Yook and J. Y. Lee, Molecular Design Strategy of Organic Thermally Activated Delayed Fluorescence Emitters, Chem. Mater., 2017, 29, 1946–1963 CrossRef CAS.
- J. Mei, N. L. C. Leung, R. T. K. Kwok, J. W. Y. Lam and B. Z. Tang, Aggregation-Induced Emission: Together We Shine, United We Soar!, Chem. Rev., 2015, 115, 11718–11940 CrossRef CAS.
- M. Ji and X. Ma, Recent progress with the application of organic room-temperature phosphorescent materials, Ind. Chem. Mater., 2023, 1, 582–594 RSC.
- F. Zinna, L. Arrico, T. Funaioli, L. Di Bari, M. Pasini, C. Botta and U. Giovanella, Modular chiral Eu(iii) complexes for efficient circularly polarized OLEDs, J. Mater. Chem. C, 2022, 10, 463–468 RSC.
- R. Datt, S. Bishnoi, H. K. H. Lee, S. Arya, S. Gupta, V. Gupta and W. C. Tsoi, Down-conversion materials for organic solar cells: Progress, challenges, and perspectives, Aggregate, 2022, 3, e185 CrossRef CAS.
- A. Gavriluta, T. Fix, A. Nonat, A. Slaoui, J.-F. Guillemoles and L. J. Charbonnière, Tuning the chemical properties of europium complexes as downshifting agents for copper indium gallium selenide solar cells, J. Mater. Chem. A, 2017, 5, 14031–14040 RSC.
- J. Andres, R. D. Hersch, J.-E. Moser and A.-S. Chauvin, A New Anti-Counterfeiting Feature Relying on Invisible Luminescent Full Color Images Printed with Lanthanide-Based Inks, Adv. Funct. Mater., 2014, 24, 5029–5036 CrossRef CAS.
- M. Cardoso Dos Santos, A. Runser, H. Bartenlian, A. M. Nonat, L. J. Charbonnière, A. S. Klymchenko, N. Hildebrandt and A. Reisch, Lanthanide-Complex-Loaded Polymer Nanoparticles for Background-Free Single-Particle and Live-Cell Imaging, Chem. Mater., 2019, 31, 4034–4041 CrossRef CAS.
- J.-C. G. Bünzli and S. V. Eliseeva, Intriguing aspects of lanthanide luminescence, Chem. Sci., 2013, 4, 1939–1949 RSC.
- J.-C. G. Bünzli and C. Piguet, Taking advantage of luminescent lanthanide ions, Chem. Soc. Rev., 2005, 34, 1048–1077 RSC.
- Y. Yang, J. Li, X. Liu, S. Zhang, K. Driesen, P. Nockemann and K. Binnemans, Listening to Lanthanide Complexes: Determination of the Intrinsic Luminescence Quantum Yield by Nonradiative Relaxation, Chem. Phys. Chem., 2008, 9, 600–606 CrossRef CAS.
- S. Miyazaki, K. Goushi, Y. Kitagawa, Y. Hasegawa, C. Adachi, K. Miyata and K. Onda, Highly efficient light harvesting of a Eu(III) complex in a host–guest film by triplet sensitization, Chem. Sci., 2023, 14, 6867–6875 RSC.
- A. S. Kalyakina, V. V. Utochnikova, M. Zimmer, F. Dietrich, A. M. Kaczmarek, R. Van Deun, A. A. Vashchenko, A. S. Goloveshkin, M. Nieger, M. Gerhards, U. Schepers and S. Bräse, Remarkable high efficiency of red emitters using Eu(III) ternary complexes, Chem. Commun., 2018, 54, 5221–5224 RSC.
- M. S. Khan, R. Ilmi, W. Sun, J. D. L. Dutra, W. F. Oliveira, L. Zhou, W.-Y. Wong and P. R. Raithby, Bright and
efficient red emitting electroluminescent devices fabricated from ternary europium complexes, J. Mater. Chem. C, 2020, 8, 5600–5612 RSC.
- R. Ilmi, X. Li, N. K. Al Rasbi, L. Zhou, W.-Y. Wong, P. R. Raithby and M. S. Khan, Two new red-emitting ternary europium(III) complexes with high photoluminescence quantum yields and exceptional performance in OLED devices, Dalt. Trans., 2023, 52, 12885–12891 RSC.
- O. Moudam, B. C. Rowan, M. Alamiry, P. Richardson, B. S. Richards, A. C. Jones and N. Robertson, Europium complexes with high total photoluminescence quantum yields in solution and in PMMA, Chem. Commun., 2009, 6649–6651 RSC.
- M. H. V. Werts, R. T. F. Jukes and J. W. Verhoeven, The emission spectrum and the radiative lifetime of Eu3+ in luminescent lanthanide complexes, Phys. Chem. Chem. Phys., 2002, 4, 1542–1548 RSC.
- Y. Kitagawa, M. Tsurui and Y. Hasegawa, Bright red emission with high color purity from Eu(III) complexes with π-conjugated polycyclic aromatic ligands and their sensing applications, RSC Adv., 2022, 12, 810–821 RSC.
- S. Sato and M. Wada, Relations between Intramolecular Energy Transfer Efficiencies and Triplet State Energies in Rare Earth β-diketone Chelates, Bull. Chem. Soc. Jpn., 1970, 43, 1955–1962 CrossRef CAS.
- M. Latva, H. Takalo, V.-M. Mukkala, C. Matachescu, J. C. Rodríguez-Ubis and J. Kankare, Correlation between the lowest triplet state energy level of the ligand and lanthanide(III) luminescence quantum yield, J. Lumin., 1997, 75, 149–169 CrossRef CAS.
- M. Pietraszkiewicz, M. Maciejczyk, I. D. W. Samuel and S. Zhang, Highly photo- and electroluminescent 1,3-diketonate Eu(III) complexes with spiro-fluorene-xantphos dioxide ligands: synthesis and properties, J. Mater. Chem. C, 2013, 1, 8028–8032 RSC.
- R. Devi, R. Boddula, J. Tagare, A. B. Kajjam, K. Singh and S. Vaidyanathan, White emissive europium complex with CRI 95%: butterfly vs. triangle structure, J. Mater. Chem. C, 2020, 8, 11715–11726 RSC.
- Y. Kitagawa, F. Suzue, T. Nakanishi, K. Fushimi, T. Seki, H. Ito and Y. Hasegawa, Stacked nanocarbon photosensitizer for efficient blue light excited Eu(III) emission, Commun. Chem., 2020, 3, 3 CrossRef CAS.
- Y. Hasegawa, S. Shoji and Y. Kitagawa, Luminescent Eu(III)-based Coordination Polymers for Photonic Materials, Chem. Lett., 2022, 51, 185–196 CrossRef CAS.
- Y. Hirai, T. Nakanishi, Y. Kitagawa, K. Fushimi, T. Seki, H. Ito and Y. Hasegawa, Luminescent Europium(III) Coordination Zippers Linked with Thiophene-Based Bridges, Angew. Chem., Int. Ed., 2016, 55, 12059–12062 CrossRef CAS.
- Y. Hirai, T. Nakanishi, Y. Kitagawa, K. Fushimi, T. Seki, H. Ito and Y. Hasegawa, Triboluminescence of Lanthanide Coordination Polymers with Face-to-Face Arranged Substituents, Angew. Chem., Int. Ed., 2017, 56, 7171–7175 CrossRef CAS.
- K. Miyata, T. Ohba, A. Kobayashi, M. Kato, T. Nakanishi, K. Fushimi and Y. Hasegawa, Thermostable Organo-phosphor: Low-Vibrational Coordination Polymers That Exhibit Different Intermolecular Interactions, ChemPlusChem, 2012, 77, 277–280 CrossRef CAS.
- S. V. Eliseeva, D. N. Pleshkov, K. A. Lyssenko, L. S. Lepnev, J.-C. G. Bünzli and N. P. Kuzmina, Highly Luminescent and Triboluminescent Coordination Polymers Assembled from Lanthanide β-Diketonates and Aromatic Bidentate O-Donor Ligands, Inorg. Chem., 2010, 49, 9300–9311 CrossRef CAS.
- H. Nakanotani, T. Higuchi, T. Furukawa, K. Masui, K. Morimoto, M. Numata, H. Tanaka, Y. Sagara, T. Yasuda and C. Adachi, High-efficiency
organic light-emitting diodes with fluorescent emitters, Nat. Commun., 2014, 5, 4016 CrossRef CAS.
- M. Y. Wong and E. Zysman-Colman, Purely Organic Thermally Activated Delayed Fluorescence Materials for Organic Light-Emitting Diodes, Adv. Mater., 2017, 29, 1605444 CrossRef.
- N. Sharma, M. Maciejczyk, D. Hall, W. Li, V. Liégeois, D. Beljonne, Y. Olivier, N. Robertson, I. D. W. Samuel and E. Zysman-Colman, Spiro-Based Thermally Activated Delayed Fluorescence Emitters with Reduced Nonradiative Decay for High-Quantum-Efficiency, Low-Roll-Off, Organic Light-Emitting Diodes, ACS Appl. Mater. Interfaces, 2021, 13, 44628–44640 CrossRef CAS.
- W. H. Melhuish, Quantum efficiencies of fluorescence of organic substances: Effect of solvent and concentration efficiencies of fluorescent solute1, J. Phys. Chem., 1961, 65, 229–235 CrossRef CAS.
- V. Divya, R. O. Freire and M. L. P. Reddy, Tuning of the excitation wavelength from UV to visible region in Eu3+ -β-diketonate complexes: Comparison of theoretical and experimental photophysical properties, Dalton. Trans., 2011, 40, 3257–3268 RSC.
- H. Xu, L.-H. Wang, X.-H. Zhu, K. Yin, G.-Y. Zhong, X.-Y. Hou and W. Huang, Application of Chelate Phosphine Oxide Ligand in Eu Complex with Mezzo Triplet Energy Level, Highly Efficient Photoluminescent, and Electroluminescent Performances, J. Phys. Chem. B, 2006, 110, 3023–3029 CrossRef CAS.
- K. Binnemans, Interpretation of europium(III) spectra, Coord. Chem. Rev., 2015, 295, 1–45 CrossRef CAS.
- V. Jornet-Mollá, C. Dreessen and F. M. Romero, Robust Lanthanoid Picolinate-Based Coordination Polymers for Luminescence and Sensing Applications, Inorg. Chem., 2021, 60, 10572–10584 CrossRef.
- Y. Hasegawa, S. Tateno, M. Yamamoto, T. Nakanishi, Y. Kitagawa, T. Seki, H. Ito and K. Fushimi, Effective Photo- and Triboluminescent Europium(III) Coordination Polymers with Rigid Triangular Spacer Ligands, Chem. – Eur. J., 2017, 23, 2666–2672 CrossRef CAS.
- N. Koiso, Y. Kitagawa, T. Nakanishi, K. Fushimi and Y. Hasegawa, Eu(III) Chiral Coordination Polymer with a Structural Transformation System, Inorg. Chem., 2017, 56, 5741–5747 CrossRef CAS.
Footnote |
† Electronic supplementary information (ESI) available: [NMR spectra, HRMS, IR, TGA, optical properties and sensitization mechanism are included in the supporting information]. See DOI: https://doi.org/10.1039/d4cp01610f |
|
This journal is © the Owner Societies 2024 |
Click here to see how this site uses Cookies. View our privacy policy here.