A molecular beam study of CO oxidation on Pd clusters supported on alumina: the effect of cluster size†
Received
8th March 2024
, Accepted 2nd May 2024
First published on 3rd May 2024
Abstract
The activity in CO oxidation of regular arrays of Pd clusters, in the size range of 5–180 atoms, supported on alumina has been studied by using a molecular beam method. The Pd clusters are grown on a nanostructured ultrathin alumina film on Ni3Al(111) providing a hexagonal array of clusters with a narrow size distribution. The steady state turnover frequency (TOF) is measured as a function of the mean cluster size. On average the TOF increases with size. Below about 20 atoms, the TOF increases rapidly and for larger clusters it grows slowly and reaches a value close to those obtained on bulk single crystals at 180 atoms. The size effect is explained by the smaller activity of Pd atoms in contact with the alumina support.
1. Introduction
Nowadays, one of the main goals in heterogeneous catalysis research is to reduce the consumption of Pt-group metals that are rare and expensive. One way to reduce the precious metal content in the catalysts is to reduce the size of the metal particles. However, the catalytic activity often depends on the particle size, and therefore if we want to use small clusters as catalyst it becomes necessary to precisely know the dependence of the steady activity as a function of cluster size. CO oxidation on metals by the Pt group is one of the most studied catalytic reactions.1 On single crystals (Pt, Pd) Ertl and co-workers have evidenced that the reaction mechanism is of the Langmuir–Hinshelwood type by using molecular beam methods.2 The reaction is considered to be structure insensitive because the catalytic activity, for given reaction parameters, is almost independent of the surface structure.2 Following a Boudart statement, a structure insensitive reaction should not depend on the metal particle size.3 Later, Boudart and Poppa have studied the CO oxidation reaction, at low pressure, on Pd nanoparticles grown under UHV on α-alumina single crystals.4 The size of the Pd particles was measured via TEM. They showed that in the CO-rich regime (low temperature) the catalytic activity per surface atom and per second (TOF: turnover frequency) is independent of particle size while in the O-rich regime (high temperature) the TOF is constant for large sizes but increases when particle sizes decrease from 4 to 1.5 nm. This fact, in contradiction to the expectations for a structure insensitive reaction, was later explained by an effect of reverse-spillover of CO on the alumina support.5,6 The reverse-spillover effect corresponds to the capture of physisorbed CO diffusing on the oxide support by metal clusters. It depends on the size and on the surface density of clusters. A detailed account of this effect can be found in a recent review.7 A molecular beam study of CO oxidation on Pd clusters (2.8, 6.8 and 13 nm), epitaxially grown on MgO (100), has shown similar results: an increase of the TOF when the particle size decreases.8 The reverse spillover fitted perfectly the TOF vs. temperature curve for the larger particles while for smaller ones, only a reasonably good agreement was obtained. This small discrepancy was attributed to an intrinsic size effect due the presence of different facets and surface defects while the reverse spillover was defined as an extrinsic size effect.9 By using the same molecular beam technique, a decrease of the TOF was observed in 1.8 nm Pd particles on alumina ultrathin films compared to 5.5 nm particles.10 All these surface science studies of CO oxidation on supported model catalysts were limited by a rather large size distribution (with a size dispersion at least 25% of the mean size).
In order to reduce the size dispersion, metal clusters can be grown epitaxially on a template constituted by a nanostructured substrate leading to regular arrays of clusters.7 For model catalysis studies, a particularly interesting support is an ultrathin alumina film obtained by high temperature oxidation of a Ni3Al (111) surface.11 On these arrays of clusters, the size dispersion is close to 1/√N, N being the mean number of atoms in the clusters.7 Another advantage of these arrays is that the effect of the reverse spillover on the reaction kinetics can be exactly calculated.7 The CO oxidation reaction has been studied on Pd arrays of clusters with a size of 181 ± 13 atoms by using a molecular beam method. After correction of the reverse-spillover effect the steady state TOF was found to be equal to that measured on Pd(111).12 A more recent study by the same method has shown that the TOF for clusters from 2 to 5 atoms was constant and that single atoms were not active.13 In the previous report13 the TOF for five atom clusters was higher than in the present study because the oxygen pressure was five times higher and the CO equivalent pressure was the same.
However, to know the activity of a cluster with a precise number of atoms the only way is to prepare the clusters in the gas phase and to select a particular size using a mass filter, before deposition on the substrate at low kinetic energy.14 Several groups have studied catalytic oxidation of CO of size-selected deposited Pd clusters between 1 and 30 atoms.14–18 However, in most of these studies the reactivity was studied via a thermal programmed reaction (TPR) of pre-adsorbed of CO and O2 molecules at low temperature. This method does not provide a steady state activity that could be compared with measurements on supported nanoparticles or single crystals. Meanwhile, steady state activity measurements have been obtained either by pulsed valves16 or by capillary tubes coming close to the sample surface.18 For the first study on Pd clusters of 8, 13 and 30 atoms supported on MgO(100) thin films, the activity per the total number of atoms in the clusters shows the maximum TOF for 13 atoms.16 However, as the 30 atom clusters are expected to be tridimensional only surface atoms are active then the real TOF must be larger. In the second study, the steady state activity per atom was measured for clusters of 5, 20 and 25 atoms deposited on alumina films and found to increase with cluster size.18 Another way to have a very narrow size distribution of nanoparticles is to use colloidal synthesis giving a size dispersion of below 6%. Murray and coworkers used this method for Pd nanoparticles (diameters of 2.5, 3.8 and 6 nm) supported on alumina and ceria powders.19 On alumina, no size effect was observed. On ceria, the TOF increased with decreasing size. In this last case, the size effect is explained by a larger activity at the periphery of the nanoparticles via a Mars–van Krevelen reaction mechanism20 that does not occur for non-reducible supports like alumina.
In this paper we measure by using a molecular beam method the steady state activity of CO oxidation on regular arrays of Pd clusters, with a low size dispersion, grown on alumina on the Ni3Al (111) surface. The mean size of the clusters is varied from 5 to 180 atoms. For small clusters (5 to 20 atoms), although the TOF can increase or decrease when the size grows, on average a rapid increase with size is observed. For large clusters the TOF increases slowly towards the value measured for extended Pd surfaces. The observed variations are discussed in terms of intrinsic size effects due to interface atoms and low coordination atoms and extrinsic size effects due to reverse-spillover of reactants.
2. Experimental section
2.1 Sample preparation
The alumina ultrathin films are prepared following the method of C. Becker.21 Ni3Al(111) surfaces are prepared by carrying out several cycles of argon sputtering and annealing at 1100 K. The temperature of the sample is precisely measured using a thermocouple in contact with the Ni3Al single crystal and using an optical pyrometer. The alumina ultrathin film is obtained by high temperature (1000 K) oxidation of the Ni3Al surface under pure oxygen (5 × 10−8 mbar, total exposure of 45 L). The quality of the alumina film is in situ checked by low electron energy diffraction (LEED). Pd clusters are epitaxially grown, by deposition at 373 K, of a flux of Pd atoms (2.3 ± 0.03 × 1012 atoms per s cm2) issued from a water cooled Knudsen cell. The Pd flux is measured in situ before each deposition using a quartz microbalance. During Pd deposition, the pressure in the UHV chamber remains in the 10−10 mbar range. The Pd clusters grow exclusively on the ‘dot’ structure of the alumina film forming a hexagonal array of clusters with a Poisson distribution (the size dispersion ΔN/N = 1/√N%, N being the mean number of atoms in the clusters). The density of clusters is constant and is equal to the density of nodes of the ‘dot’ structure (ns = 6.5 × 1012 cm−2).11 The quality and the reproducibility of the organization of the clusters has been checked in situ by STM and GISAXS (grazing incidence X-ray scattering) in previous studies.11,22
2.2 Reactivity measurements
The reactivity of the Pd clusters is studied at constant temperature with a background pressure of isotopically labeled oxygen (18O2) and a CO molecular beam. The CO beam is produced by supersonic expansion and is collimated in order that all CO molecules impinge only the sample surface. The intensity of the CO beam (JCO = 2 × 1013 cm−2 s−1, equivalent pressure: PCO = 6.8 × 10−8 mbar) is modulated by a programmable shutter. The background pressure in the vacuum chamber increases to 5 × 10−10 mbar when the CO beam is on. The intensity of the flux of molecules leaving the substrate (CO2 and unreacted CO) are measured by a quadrupole mass spectrometer working in a pulse-counting mode. Before introducing 18O2 into the chamber the intensity of the flux of CO leaving the sample is measured (at T > 473 K the adsorption of CO on the Pd clusters is fully reversible then the desorbing flux is equal to the impinging flux). Generally, we divide the CO2 flux (VCO2) by the CO impinging flux in order to be insensitive to a change of the mass spectrometer sensitivity; this ratio is called the global reaction probability (RPg). To measure the CO2 resulting from the CO oxidation reaction we detect the mass 46 corresponding to 12C16O18O produced by the Langmuir–Hinshelwood mechanism. We have shown previously that no CO2 is produced via the Mars–van Krevelen mechanism.13 It is important to note that for each new synthesis of a cluster array, there is a phase of 20 to 30 minutes during which the activity of the clusters increases before reaching a stationary activity. This period depends on the size and the temperature at which the clusters have been produced. This ‘activation period’ is explained by the reorganization of the cluster shape under the reactive atmosphere.
3. Experimental results
3.1 Effect of substrate temperature
Fig. 1 displays the global reaction probability (RPg = VCO2/JCO) of CO for an isotropic pressure of oxygen PO2 = 1 × 10−8 mbar and a pulse of CO, as a function of time and for different substrate temperatures, measured for clusters containing 80 ± 9 atoms. It can be seen that after a transient period when the CO beam is turned on, a steady state is installed at about 30 seconds. The variations of the steady state value of the CO2 production (VSS) as a function of substrate temperature are plotted in Fig. 2.
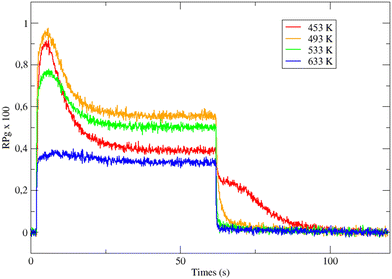 |
| Fig. 1 Time evolution of the CO2 production in response to a molecular beam pulse of CO in a background of oxygen (1.1 × 10−8 mbar) on clusters of 80 ± 9 atoms at various temperatures. | |
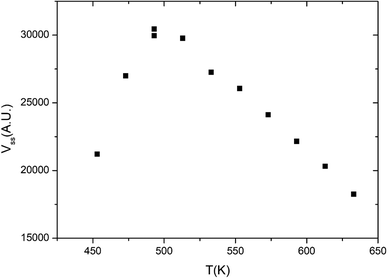 |
| Fig. 2 Steady state CO2 production as a function of temperature on Pd clusters of 80 ± 9 atoms. PO2 = 1 × 10−8 mbar, PCO (equivalent) = 6.8 × 10−8 mbar. | |
When temperature increases the steady state production of CO2 increases rapidly and reach a maximum near 500 K, then it slowly decreases. This is a typical evolution already observed on Pd extended surfaces2 and on supported nanoparticles4,8,12 or clusters.18 At low temperature, the CO coverage is large and impedes the dissociative adsorption of oxygen leading to low reaction rates. When temperature increases the steady state CO coverage decreases and the reaction rate grows. This is the ‘CO-rich’ regime. At high temperature, the oxygen coverage is large and close to saturation but the CO coverage is low and decreases rapidly because of fast CO desorption explaining why the CO2 production drops; this is the ‘O-rich’ regime. The CO2 transient peak, observed on Fig. 1 at the beginning of the CO pulse for T ≤ 533 K occurs because PO2/PCO is smaller than 0.5. When the CO beam is on, the O-coverage (θO) is close to saturation and CO coverage (θCO) increases rapidly with time then the θO/θCO ratio decreases down to the optimal value for the reaction at the maximum of the peak then it continues to decrease towards the steady state value. At the highest temperature (633 K) θO/θCO is always larger than the optimal value then the transient peak is no longer observed. On Fig. 1 at 453 K when the CO beam is closed, a CO2 production is still observed for about 30 s. This second peak of CO2 is due to a high CO coverage inhibiting oxygen adsorption at steady state; then when the CO beam is turned off, the oxygen coverage increases rapidly increasing the reaction rate until the consumption of all adsorbed CO. The second peak of CO2 production has been already observed on Pd nanoparticles.8,9,23–25 It is not only due to the inhibition of oxygen adsorption at high CO coverage but it also occurs when defects are present on the Pd nanoparticles.9,23,25
3.2 Effect of oxygen pressure
Fig. 3 displays CO2 pulses obtained on Pd clusters containing 180 ± 13 atoms at 533 K and for various oxygen pressures. We can see that a CO2 transient peak is present at lower oxygen pressures and not for the largest oxygen pressures when PO2/PCO > 0.5. Fig. 4 shows that at 533 K the steady state production of CO2 increases with oxygen pressure.
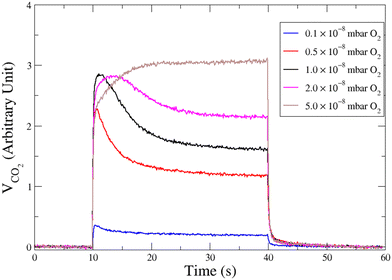 |
| Fig. 3 Time evolution of the CO2 production in response of a molecular beam pulse of CO in a background of oxygen with various pressures, on clusters of 180 ± 13 atoms at 533 K. | |
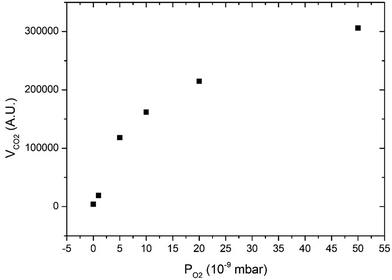 |
| Fig. 4 Steady state production of CO2 as a function of oxygen pressure at 533 K on clusters of 180 ± 13 atoms. | |
3.3 Size effect
In Fig. 5 the global reaction probability is plotted as a function of the mean cluster size (N). Below about 10 atoms, the activity (RPg) evolves slowly but for larger sizes it increases more rapidly. We can also calculate the activity per cluster (Aclu) that is equal to (RPg JCO)/ns. In fact, it is equal to 3.077 RPg. The activity per cluster increases non-linearly with cluster size. This behavior was expected because, when the size of the clusters increases the number of exposed atoms per cluster increases. In order to correct this effect we calculate the activity per deposited atoms (Aat) by dividing Aclu by N. On Fig. 6 we can see that, on average, Aat increases rapidly with cluster size below about 20 atoms then Aat slowly decreases. In fact, at small sizes, fluctuations of the reactivity are visible. These fluctuations are due several reasons. Firstly, there is a distribution of the cluster size even it is small. It is known from studies of purely size selected clusters14 that the activity depends on the exact number of atoms in the cluster14 in the ‘non scalable regime’. Secondly, for a given number of atoms a cluster can have different shapes (fluxionality) that correspond to different catalytic activities. However, despite these fluctuations there is, on average, a net increase of the activity when the mean size (N) increases up to about 20 atoms.
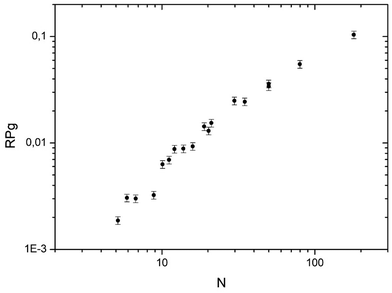 |
| Fig. 5 Activity in CO oxidation (RPg) at 533 K plotted as function of the mean number of atoms in the clusters (PO2 = 1 × 10−8 mbar and PO2/PCO = 0.19). | |
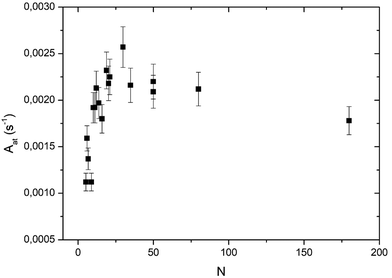 |
| Fig. 6 Activity per atom at 533 K as a function of the number of atoms (N) in the clusters. | |
At this point, we have to remind the reader that the larger clusters (80 and 180 atoms) have a 3D-shape and that the smallest ones are 2D allowing all the atoms to be active, in contrast to larger clusters. In fact, we have to calculate the activity per surface atoms that is the TOF. However, to calculate the TOF we have to know the exact shape of the clusters. In a previous study, we concluded that the clusters smaller than five atoms are 2D.13 From STM studies, we concluded that clusters containing at least 35 atoms are 3D.11 Assuming the shape of a truncated sphere, we found a contact angle of 92° meaning an almost hemispherical shape.11 For size-selected Pt clusters deposited on alumina ultrathin films on NiAl(110) the group of Watanabe has found, from STM studies, that the transition between monolayer and bilayer clusters occurs at around 20 atoms and the growth of the third layer appears at around 30 atoms.26 In order to derive the TOF we consider that clusters from 5 to 19 atoms are 2D, the 19-atom cluster being a regular hexagon. We also assume that from 20 to 31 atoms the second layer grows and that a third layer is present between 32 and 37 atoms. We call this model of clusters a ‘layer model’ (see ball models for clusters of 19, 31 and 37 atoms in the ESI†).
Taking into account the model for the cluster shape it is possible to calculate the number of surface atoms (Ns) and then the TOF that is equal to Aat × (N/Ns). For larger clusters, we will use the hemispherical model. The number of surface atoms divided by the total number of atoms is Ns/N = 3Novo/R. No is the number of atoms per unit area (we take 1.53 × 1015 cm−2 corresponding to a Pd (111) surface) and vo the Pd atomic volume (1.47 × 10−23 cm3). The cluster radius R = (3vo/2π)1/3.N1/3. Fig. 7 displays the TOF values calculated from the two models (layer model and hemisphere model) the two models overlap for the point at N = 35 atoms. The layer model shows a rapid increase (more or less linear) of the TOF when the number of atoms increases from 5 to about 30 atoms. The hemispherical model shows a slow evolution toward a saturation of around 180 atoms. It is clear that the two models cannot be extrapolated over 35 atoms for the layer model and below 35 atoms for the hemispherical model.
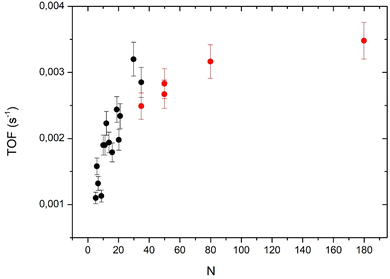 |
| Fig. 7 TOF at 533 K as a function of the mean number of atoms in the clusters. The number of surface atoms is calculated using the layer model (black points) or a hemisphere model (red points) for the cluster shape. | |
4. Discussion
4.1 Size effect for small sizes: the role of interface atoms (N = 5–30 atoms)
Fig. 7 shows two different behaviors for large and small clusters. For small clusters (below 30 atoms), the TOF decreases rapidly when cluster size decreases (despite some fluctuations already discussed about Fig. 5). This drop in the activity could be due to the interaction of interface Pd atoms with the substrate that modifies their electronic properties. Indeed, for a very close system, Pt clusters supported on an ultrathin alumina film on NiAl(110), the group of Watanabe has observed that the activity in CO oxidation was strongly reduced for 2D-clusters.26 However, if we consider that all interface atoms are less active than the other atoms one would expect a constant (low) TOF between 5 to 19 atoms (following the layer model) but it is not the case. We have also to take into account for the coordination of the interface atoms with neighboring Pd atoms: higher this coordination number lower the decrease of activity by the interaction with the substrate. Watanabe et al.26 considered that Pt atoms at the periphery of the first atomic layer are cationic, and so, show little activity in CO oxidation. Without theoretical calculations, it is difficult to take precisely account of the effect of the coordination. Following this model, for clusters larger than 20 atoms only interfacial atoms at the periphery of the clusters would have a reduced activity. When the size further increases, the proportion of these periphery atoms decreasing rapidly, the effect of the reduced activity of periphery atoms would be less important.
4.2 Size effect for large clusters (N = 35–180)
For large clusters the TOF increases slowly and tends to saturate at N = 180. Previously, we have measured the TOF for N = 181 ± 13 atoms and shown that after the correction of the reverse-spillover effect the TOF was nearly the same as that for a Pd(111) extended surface.12 In order to know if the increase of the TOF for large cluster is only due to the reverse-spillover effect we have calculated this effect (see the ESI†). As we are in the O-rich regime, the adsorption of CO is the rate limiting step for the reaction. It is legitimate to take into account the reverse spillover for CO (see the ESI†). For a mean size of 180 atoms the reverse spillover of CO increases the CO flux joining the clusters and then the TOF by a factor 1.5, but as the size decreases this effect increases the TOF more and more, and for a 35 atom cluster this increasing factor is 2.4. After removing the effect of the reverse-spillover, the corrected TOF still increases with cluster size (see Fig. S1 and S2 of the ESI†). Therefore, the reverse spillover of CO cannot explain the rise of activity measured between 35 and 180 atoms nor in the size range between 5 and 30 atoms. The weak activity of interface atoms could also explain the increase of activity with the cluster size observed in the whole size range.
Concerning very small clusters, the reverse spillover of CO can strongly increase the CO flux joining the clusters and the CO coverage could become large enough to move the reaction CO-rich regime. Thus, if we are in the CO-rich regime, in contrast, we have to consider the reverse spillover of oxygen. However, as we have discussed in a previous paper12 the reverse spillover of oxygen is negligible for Pd clusters on alumina. Previously, we had measured the CO adsorption energy by a molecular beam method and found that it showed a minimum value at around 140 atoms. Below this size the adsorption energy fluctuates depending on the exact number of atoms (it is the so called ‘non-scalable’ size regime) but on average it increases rather strongly when size decreases.22 Then, for small sizes (below 20 atoms) we cannot exclude that the decrease of activity would be due to CO poisoning.
5. Conclusions
The steady state activity in CO oxidation has been measured on arrays of Pd clusters with a mean size varying from 5 to 180 atoms and supported on an ultrathin alumina film on Ni3Al (111). Below about 20 atoms, on average the TOF increases rapidly with the mean cluster size. For clusters larger than 35 atoms, the TOF increases slowly towards the bulk value which is already reached at 180 atoms. For a mean size of between 5 and 180 atoms, the TOF increases by a factor of 3. This size effect can be decomposed with an intrinsic size effect and an extrinsic size effect. The intrinsic size effect is mainly attributed to a smaller activity of the atoms in contact with the support. Its effect on the TOF becoming less important when the cluster size increases explains the observed increases of the TOF. The extrinsic size effect is due to the reverse spillover of CO that increases the flux of CO arriving on the clusters; it depends on the cluster size, on the distance between clusters and on the substrate temperature. However, as it would result in a decrease of the TOF when cluster size increases it cannot be at the origin of the observed size effect.
Conflicts of interest
There are no conflicts to declare.
References
- H. J. Freund, G. Mejer, M. Scheffler, R. Schlögl and M. Wolf, CO oxidation as a prototypical reaction for heterogeneous processes, Angew. Chem., Int. Ed., 2011, 50, 10064–10094 CrossRef CAS PubMed.
- T. Engel and G. Ertl, Catalytic oxidation on platinum metals, Adv. Catal., 1979, 28, 1 CAS.
- M. Boudart and Adv Catal, Relat. Subj., 1969, 20, 153 CAS.
- S. Ladas, H. Poppa and M. Boudart, The adsorption and Catalytic oxidation of carbon monoxide an evaporated palladium particles, Surf. Sci., 1981, 102, 151–171 CrossRef CAS.
- F. Rumpf, H. Poppa and M. Boudart, Oxidation of carbon monoxide on palladium: role of the alumina support, Langmuir, 1988, 4, 722–728 CrossRef CAS.
- C. R. Henry, On the effect of the diffusion of carbon monoxide on the substrate during CO oxidation on supported palladium clusters, Surf. Sci., 1989, 223, 519–526 CrossRef CAS.
- C. R. Henry, Perspectives’ 2D-Arrays of nanoparticles as model catalysts, Catal. Lett., 2015, 145, 731–749 CrossRef CAS.
- C. Becker and C. R. Henry, Cluster size dependent kinetics for the oxidation of CO on a Pd/MgO(100) model catalyst, Surf. Sci., 1996, 352–354, 457–462 CrossRef CAS.
-
C. R. Henry, Reaction Dynamics on Supported Metal Clusters, in The Chemical Physics of Solid Surfaces: Surface Dynamics, ed. D. P. Woodruff, Elsevier, 2003, vol. 11, ch. 9, pp. 247–290 Search PubMed.
- I. Meusel, J. Hoffman, J. Hartmann, J. Libuda and H. J. Freund, Size dependent reaction kinetics on supported model catalysts: A molecular beam/IRAS study of the CO oxidation on alumina supported Pd particles, J. Phys. Chem. B, 2001, 105, 3567 CrossRef CAS.
- M. Marsault, G. Sitja and C. R. Henry, Regular arrays of Pd and PdAu clusters on ultrathin alumina films for reactivity studies, Phys. Chem. Chem. Phys., 2014, 16, 26458–26466 RSC.
- G. Sitja and C. R. Henry, Molecular beam study of the oxidation of carbon monoxide on a regular array of Palladium clusters on alumina, J. Phys. Chem. C, 2017, 121, 10706 CrossRef CAS.
- G. Sitja and C. R. Henry, Activity of Pdn (n = 1–5) clusters on alumina film on Ni3Al (111) for CO oxidation: a molecular beam study, J. Phys. Chem. C, 2021, 125, 13247–13253 CrossRef CAS.
-
T. M. Bernhardt, U. Heiz and U. Landman, Chemical and catalytic properties of size-selected free and supported clusters in ‘Nanocatalysis’, ed. U. Heiz and U. Landman, Springer-Verlag, Berlin, 2007 Search PubMed.
- U. Heiz, A. Sanchez, S. Abbet and W. D. Schneider, Tuning the oxidation of carbon monoxide using nanoassembled model catalysts, Chem. Phys., 2000, 262, 189 CrossRef CAS.
- C. Harding, S. Kunz, V. Habibpour, V. Teslenko, M. Arenz and U. Heiz, Dual pulsed-beam controlled mole fraction studies of the catalytic oxidation of CO over supported Pd nanocatalysts, J. Catal., 2008, 255, 234–240 CrossRef CAS.
- W. E. Kaden, T. Wu, W. A. Kunkel and S. L. Anderson, Electronic structure controls reactivity of size selected Pd clusters adsorbed on TiO2 surfaces, Science, 2009, 326, 826–829 CrossRef CAS PubMed.
- M. D. Kane, F. S. Roberts and S. L. Anderson, Mass-selected supported cluster catalysts: Size effects on CO oxidation activity, electronic structure, and thermal stability of Pdn/alumina (n ≤ 30) model catalysts, Int. J. Mass Spectrom., 2014, 370, 1–15 CrossRef CAS.
- M. Cargnello, V. V. T. Doan-Nguyen, T. R. Gordon, R. E. Diaz, E. A. Stach, R. J. Gorte, P. Fornasiero and C. B. Murray, Control of metal-support interface. Role of ceria catalyst, Science, 2013, 341, 771–773 CrossRef CAS PubMed.
- G. Spezzati, A. G. Benavidez, A. T. DeLaRiva, Y. Su, J. P. Hofmann, S. Asahina, E. J. Olivier, J. H. Neethling, J. T. Miller, A. K. Datye and E. J. M. Hensen, CO oxidation by Pd supported on CeO2(100) and CeO2(111) facets, Appl. Catal., B, 2019, 243, 36–46 CrossRef CAS.
- A. Rosenhahn, J. Schneider, C. Becker and K. Wandelt, Oxidation of Ni3Al(111) at 600, 800, and 1050 K investigated by scanning tunneling microscopy, J. Vac. Sci. Technol., 2000, 18, 1923–1927 CrossRef CAS.
- G. Sitja, S. Le Moal, M. Marsault, G. Hamm, F. Leroy and C. R. Henry, Transition from molecule to solid state: reactivity of supported metal clusters, Nano Lett., 2013, 13, 1977–1982 CrossRef CAS PubMed.
- L. Piccolo, C. Becker and C. R. Henry, Kinetic modelling of the CO oxidation reaction on supported metal clusters, Euro. Phys. J. D, 1999, 9, 415–419 CrossRef CAS.
- J. Libuda, I. Meusel, J. Hoffmann, J. Hartmann, L. Piccolo, C. R. Henry and H. J. Freund, The CO Oxidation Kinetics on Supported Pd Model Catalysts: A molecular Beam/In-situ IRAS study, J. Chem. Phys., 2001, 114, 4669–4684 CrossRef CAS.
- J. Hoffmann, I. Meusel, J. Hartmann, J. Libuda and H. J. Freund, Reaction kinetics on heterogeneous model catalysts: The CO oxidation on alumina-supported Pd particles, J. Catal., 2001, 204, 378 CrossRef CAS.
- A. Beniya, S. Higashi, N. Ohba, R. Jinnouchi, H. Hirata and Y. Watanabe, CO oxidation activity of non-reducible oxide-supported mass-selected few-atom Pt single-clusters, Nat. Commun., 2020, 11, 1888 CrossRef CAS PubMed.
|
This journal is © the Owner Societies 2024 |