Photo-induced structural dynamics of o-nitrophenol by ultrafast electron diffraction†
Received
22nd December 2023
, Accepted 13th May 2024
First published on 16th May 2024
Abstract
The photo-induced dynamics of o-nitrophenol, particularly its photolysis, has garnered significant scientific interest as a potential source of nitrous acid in the atmosphere. Although the photolysis products and preceding photo-induced electronic structure dynamics have been investigated extensively, the nuclear dynamics accompanying the non-radiative relaxation of o-nitrophenol on the ultrafast timescale, which include an intramolecular proton transfer step, have not been experimentally resolved. Herein, we present a direct observation of the ultrafast nuclear motions mediating photo-relaxation using ultrafast electron diffraction. This work spatiotemporally resolves the loss of planarity which enables access to a conical intersection between the first excited state and the ground state after the proton transfer step, on the femtosecond timescale and with sub-Angstrom resolution. Our observations, supported by ab initio multiple spawning simulations, provide new insights into the proton transfer mediated relaxation mechanism in o-nitrophenol.
1. Introduction
The photolysis of nitroaromatic hydrocarbons has sparked significant scientific interest as a possible anthropogenic source of atmospheric nitrous acid (HONO),1 a highly reactive precursor to OH radical formation which in turn plays a key role in the formation of photochemical smog in the troposphere.2,3 The study of ortho-substituted nitroaromatic compounds, of which o-nitrophenol is an example, has focused primarily on their unique intramolecular charge transfer known to facilitate intramolecular proton transfer (IPT).4–6 Proton transfer reactions are involved in the control of fundamental processes in biological systems such as the proton-coupled electron transfer in water oxidation by photosystem II,7 the light-driven proton pump in bacteriorhodopsin,8,9 and light emission from green fluorescent protein.10 Moreover, photochemical IPT reactions have found applications in fluorescence-based sensors,11 laser dyes,12 organic light-emitting diodes,13 solar collectors,14 and molecular logic gates.15 The ortho-substituted nitroaromatic o-nitrophenol is a prototypical model system for the study of IPT mediated photolysis. O-nitrophenol is a bifunctional push–pull molecule with both hydrogen-atom donor and acceptor groups near each other. In the ground state (S0) o-nitrophenol exhibits a planar geometry and an intramolecular six-membered H-chelate ring formed by strong hydrogen bonding16 between the hydrogen-donating hydroxyl (–OH) and electron-withdrawing nitro (–NO2) groups. In the presence of UV-vis light, o-nitrophenol undergoes IPT via the transfer of the proton from the hydroxyl group to the adjacent nitro group, giving rise to a nitrous acid (HONO) motif. The newly formed aci-nitro isomer of o-nitrophenol, henceforth referred to as aci-nitro,17 can undergo dissociation to release HONO.18 The photo-induced dynamics and photolysis products of o-nitrophenol have been investigated extensively. Takezaki et al. based on solution phase time-resolved transient absorption experiments, proposed the involvement of the triplet manifold in the relaxation dynamics.19 Ernst et al. used femtosecond transient absorption spectroscopy in solution and gas-phase time-resolved photoelectron spectroscopy to capture evidence of HONO formation. They proposed proton transfer occurs in the S1 (ππ*) state, driven by the strong charge transfer character, and that internal conversion to the ground state occurs via a conical intersection accessible through the deplanarization of the newly formed HONO group. An alternative relaxation pathway involving sub-picosecond intersystem crossing (ISC) to the upper triplet manifold and HONO release was also proposed.20 Using time-resolved photoelectron spectroscopy with 29.5 eV probe pulses from a high harmonic light source, Nitta et al. followed the photolysis of o-nitrophenol from excitation to HONO release. They report sub 100 fs proton transfer in the S1 state followed by ISC to the triplet manifold, where the aci-nitro deplanarizes and releases HONO in the sub-picosecond timescale.21 Theoretical studies by Xu et al., proposed three competing relaxation pathways involving the triplet manifold: non-hydrogen transfer on a time scale of 300 fs, tunnelling hydrogen transfer on a time scale of 10 ps, and direct hydrogen transfer on a time scale of 40 fs.22 Despite numerous spectroscopic observations and computation studies,17,23,24 the complex relaxation pathway taken by o-nitrophenol in the presence of UV-vis light remains the topic of much interest and speculation. This is in part due to the lack of experimental verification of the nuclear dynamics along the reaction coordinate. For example, the deplanarization of aci-nitro, proposed by Ernst et al. to enable access to the S1/S0 conical intersection, and subsequently reported by Nitta et al. to facilitate ISC, has never been resolved experimentally. Here, we report the direct imaging of the photo-relaxation dynamics of o-nitrophenol, following optical excitation to the S1(ππ*) excited state with 330 nm femtosecond laser pulses, using a combination of mega-electron-volt gas-phase UED (MeV-UED)25,26 and ab initio multiple spawning (AIMS)27,28 simulations. Although generally insensitive to electronic structure dynamics and thus unable to challenge or validate the involvement of the triplet manifold in o-nitrophenol dynamics, MeV-UED is directly sensitive to nuclear structure,29–33 and therefore uniquely placed to offers unprecedented insights into the nuclear motions and timescales associated with the photo-relaxation of o-nitrophenol. Complemented by AIMS simulations, experimentally resolved nuclear dynamics here revealed provide a much-needed structural view on the photo-induced dynamics of o-nitrophenol Fig. 1.
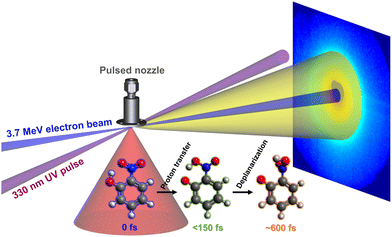 |
| Fig. 1 Schematic of the UED experimental setup with ball-and-stick representations of key experimentally resolved geometries (ground state o-nitrophenol, aci-nitro isomer and deplanarized aci-nitro isomer). A 330 nm UV pulse photoexcites a gas plume of o-nitrophenol delivered to the experimental chamber by a pulsed nozzle. An incident 3.7 MeV electron beam probes the gas volume and the resulting diffraction patterns are detected on a back-illuminated phosphor screen detector. | |
2. Experimental and computational details
2.1. Mega-electron volt gas-phase ultrafast electron diffraction
The experimental setup is described in detail elsewhere.25,26 Briefly, the third harmonic of the 800 nm Ti:sapphire laser system is used to produce electron bunches containing approximately 104 electrons at the photocathode of an RF-photo injector. The electron bunches are accelerated to a kinetic energy of 3.7 MeV by an RF cavity, ejected from the RF-photo injector at a repetition rate of 180 Hz and focused to a spot size of 146 × 206 μm full-width at half-maximum at the experimental chamber interaction region. O-nitrophenol, purchased from Sigma Aldrich, was delivered to the interaction region without further purification using a solenoid pulsed valve with 100 μm diameter orifice. The reservoir of o-nitrophenol was heated to 166 °C and a temperature gradient of approximately 30 °C was maintained between the reservoir and the valve to prevent condensation along the sample transport lines. The gas plume of o-nitrophenol was illuminated with a 330 nm pump laser generated using an optical parametric amplifier (OPA) and delivered to the interaction region with a spot size of 219 × 176 μm full-width at half-maximum at an energy of 30 μJ. The duration of the 330 nm pump laser pulse was estimated to be 100 fs based on the 70 fs pulse duration measured at the OPA input. The instrument response function of the UED setup was estimated at 150 fs.26 The time-delay between the arrival of the optical pump and electron probe pulses was varied using the delay stage on the pump path. Scattered electrons were detected using a phosphor screen imaged by an electron-multiplying charge-couple device (EMCCD) camera. Time-dependent diffraction patterns were recorded as 20 s exposures for delay time points between −2 and 2 ps. Multiple scans were performed to achieve a total integration of 18 minutes per delay point and at each scan the order of the delay points was randomized to avoid systematic errors. Details on the data processing are described in Section 1 of the (ESI†).
2.2. Genetic structure fitting algorithm
A genetic structural fitting algorithm (GSFA) was used to retrieve structural information directly from the experimental scattering signal.34–36 In the GSFA, a gene pool of structures with randomly-chosen structural parameters was generated based on a parametric model of o-nitrophenol. Structures for which calculated difference diffraction signals were in good agreement with experimental data were selected and propagated to the next generation. The diversity of the gene pool was preserved through cross-over and mutation and, after 100 generations, the structure corresponding to the lowest χ2 value was taken as the fitted output. The GSFA was repeated several times for each time delay to retrieve a pool of representative structures. For a detailed description of the GSFA retrieval see Section 8 in the ESI.†
2.3.
Ab initio multiple spawning simulations
The nonadiabatic dynamics of o-nitrophenol were explored using ab initio multiple spawning27,28 (AIMS) simulations. Electronic surfaces were described using the floating occupation molecular orbitals (FOMO)-complete active space configuration interaction (CASCI) method with a FOMO temperature β = 0.2 and a 2,2 active space (2 electrons in 2 orbitals) in TeraChem with the 6–31g** basis set.37 This electronic structure method was validated against SA-2-XMS(0.2)-MR-CASPT2(2,2)/6-31g*38 and EOM-CCSD/cc-pVDZ39 single-point energy calculations at two critical points: the Franck–Condon point and the S1/S0 MECI geometries optimized using FOMO-CASCI. All three methods showed consistent energetics for structural relaxation to the S1/S0 minimal-energy conical intersection (MECI). However, the FOMO(0.2)-CASCI method, which largely neglects dynamic electron correlation, overestimated the HOMO–LUMO gap by 1.6 eV, and reported a factor of 2 larger energy difference between the Franck–Condon geometry and the S1/S0 MECI compared to MS-CASPT. Despite a 1 eV overestimation in the energy difference between the Franck–Condon geometry and the S1/S0 MECI, likely to yield steeper gradients and faster dynamics, FOMO(0.2)-CASCI preserved the qualitative relationships between different geometries key to the dynamics. Although these considerations (and agreement with experiment as detailed below) suggest that more accurate methods including dynamic correlation and larger active spaces might not be needed for a semi-quantitative description of o-nitrophenol photodynamics, this should be verified with the corresponding simulations when improvements in algorithms and hardware make them feasible. For now, FOMO(0.2)-CASCI offers an adequate treatment of conical intersections and good compromise between stability and affordability relative to CASPT2 and CASSCF. See ESI† Section 9 for more details on the electronic structure validation.
Initial conditions AIMS dynamics were obtained by using a Franck–Condon geometry and Hessian computed with B3LYP/6-31g** to sample a harmonic Wigner distribution comprised of 1000 geometries and momenta. The oscillator strengths and vertical excitation energies were then calculated for each of the sampled geometries using FOMO(0.2)-CASCI(2,2)/6-31g**. These values were used to calculate the photoabsorption cross-section with a 0.025 eV FWHM Lorentzian broadening of each point. Monte Carlo sampling of the photoabsorption spectra was used to select 50 subsamples for the initial conditions, centered at the 331 nm absorption band with a 0.3 eV distribution and adjusted to account for the blue shift (1.6 eV) in the theoretical spectrum with respect to experiment. The AIMS simulations were propagated for 1 ps with a 0.25 fs timestep using FOMO(0.2)-CASCI(2,2)37 for 50 initial conditions subsampled from the geometries used to generate the photoabsorption spectrum. The nonadiabatic coupling matrix elements (NACMEs) were evaluated analytically.
The potential involvement of ultrafast intersystem crossing via triplet states, reported in previous theoretical studies,22 was investigated by running additional dynamics on a subset of initial conditions with Generalized Ab Initio Multiple Spawning (GAIMS),40 a type of AIMS that incorporates spin orbit couplings to account for intersystem crossing in addition to internal conversion. The active space was extended to include relevant triplet states by using the FOMO(0.2)-CASCI(4,3)/6-31g** electronic structure method. We found that the computed spin–orbit couplings between singlet and triplet manifolds were too weak to impact the short time-scale dynamics (see ESI† Fig. S9 for a plot of the T1 triplet surface population as a function of time). Of course, this does not preclude intersystem crossing at longer times.
3. Results
Fig. 2a shows the steady-state pair-distribution function (PDF) obtained by real-space transformation of the static diffraction patterns from gas phase o-nitrophenol. The peaks in the PDF correspond to the internuclear distances in the molecule scaled by the atomic scattering amplitudes. The experimental results are compared with the simulated PDF based on the diffraction signal obtained from the initial conditions of our AIMS simulations. A detailed description of simulation of diffraction signals is presented in Section 5 of the ESI.† The experimental and simulated PDFs in Fig. 2a show excellent quantitative agreement. The main contributions to the PDFs are the bonded distances (C–C, C–N, C–O, N–O) captured under peak α and nearest-neighbour non-bonded distances (C–C, C–O, O–O) under peak β, centered at 1.3 Å and 2.5 Å, respectively. These two sets of distances are illustrated with color-coded arrows overlaid onto the S0-state equilibrium geometry of o-nitrophenol inset in Fig. 2a.
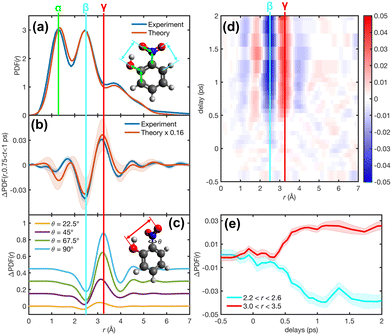 |
| Fig. 2 UED results and comparisons between experimental and theoretically predicted pair distribution functions. Experimentally measured (light blue) and theoretically predicted (orange) (a) PDF for ground-state o-nitrophenol and (b) ΔPDFs between 0.75 and 1 ps. The two strongest features in the PDFs [α (green), β (cyan)] and the strongest features in the ΔPDF [γ (red)] are marked with vertical lines. The physical significance of these features is illustrated with color-coded arrows representing interatomic distances overlaid onto the molecular structures in panels a and c. The fraction of molecules excited by the pump laser pulse is needed for direct comparison of the AIMS (“Theory”) and experimental results. We treat this as an adjustable parameter and the depicted results reflect 16% excitation efficiency (see ESI† Section 13 for details). (c) Simulated ΔPDF for the incremental torsion of the C–N bond in the ground-state equilibrium geometry of o-nitrophenol. θ represents the angle between the ON and CC bonds resulting from the torsion of the ONCC dihedral, dONCC. (d) False-color plot of the experimental ΔPDF as a function of time delay. (e) Temporal evolution of the two strongest features in the ΔPDF [β (cyan) γ (red)]. The shaded areas in panel b and e represent one standard deviation of the ΔPDF. | |
The experimental difference PDF (ΔPDF), which is the difference between delay-dependent PDF and static PDF, is used to evaluate the temporal evolution of the interatomic distances. A change of interatomic distance appears as negative contributions under the original distance and positive contribution at the new distance. The average experimental ΔPDF for time delays between 1 and 2 ps, shown in Fig. 2b, is in good qualitative agreement with the simulated signal from our AIMS simulations. Based on this agreement and the amplitude of the difference signal, we estimate to have photoexcited ≈16% of the molecules in the probed volume. The ΔPDF signal shows a bleach of the peaks associated with bonded and nearest-neighbor distances (1.3 and 2.5 Å; peaks α and β, respectively) and the growth of a peak around 3.3 Å, labelled as peak γ. The simulated ΔPDF signal for an incremental out-of-plane torsion of nitro group in o-nitrophenol, presented in Fig. 2c, show a qualitatively identical bleach of the distances centered around 1.3 and 2.5 Å and an increase in distances around 3.3 Å. Contributions to peak γ are composed predominantly of non-bonded distances between the oxygens in the hydroxyl and nitro groups which appear when the nitro group twists out-of-plane of the ring. We, therefore, assign the ΔPDF signal between 1 and 2 ps to the loss of planarity through activation of the out-of-plane torsion of the nitro group around the C–N bond axis. The onset of strong ΔPDF features consistent with the deplanarization of o-nitrophenol is shown Fig. 2d as a false-colour plot of the experimental ΔPDF as a function of time delay. The experimental ΔPDF, which reflects time-dependent changes in interatomic distances, shows a delay of approximately 400 fs between the onset of features assigned to nitro group torsion and time-zero. The delayed onset of changes at the two interatomic distances involved in the nitro group torsion and deplanarization of o-nitrophenol (peaks β and γ) are shown in Fig. 2e. The lack of strong signal in the ΔPDF between the experimental time-zero - determined independently using the Debye–Waller profile of a photo-excited Silica target - and 400 fs suggests that small amplitude structural changes occur prior to the loss of planarity. No evidence for photodissociation was found in the 2 picosecond time-window captured by the UED experiment. This observation is corroborated by the AIMS simulations, which observed <5% photodissociation in the sampled 1 picosecond time-window. For this reason, our interpretation of both experimental and theoretical results will focus exclusively on the sub-picosecond photo-relaxation dynamics of o-nitrophenol.
4. Discussion
Our AIMS simulations show that o-nitrophenol dynamics are dominated by intramolecular excited state proton transfer followed internal conversion to the ground state. As proposed by Ernst et al.,20 internal conversion occurs following the out-of-plane rotation of the newly formed HONO group, i.e. deplanarization. Triplet involvement via ultrafast intersystem crossing was not observed, contrary to previous theoretical work by Xu et al.22 Results from GAIMS simulations, which incorporates spin orbit couplings to account for intersystem crossing, were consistent with spin–orbit couplings that are too low to generate significant population transfer to the triplet manifold. We tentatively speculate that previous studies may have overestimated the population transfer with respect to spin orbit coupling. Although uniquely sensitive to nuclear motions, UED is alas unable to distinguish whether motions arise from the ground-state, single-excited state, or triplet manifold population. Therefore, our interpretation of UED results is focused primarily on the nuclear structure dynamics. Our description of electronic structure dynamics is, therefore, based solely on AIMS simulations, which notwithstanding the lack of triplet manifold involvement, captured motions in remarkable qualitative agreement with those experimentally retrieved from UED data.
To elucidate further the observed structural changes in the ΔPDFs, we employed a genetic structural fitting algorithm (GSFA)34–36 to retrieve the temporal evolution of key internal coordinates from the experimental reciprocal-space difference signal directly. The GSFA was used to investigate the deplanarization of o-nitrophenol through the experimental retrieval of the dihedral angle between the plane of the nitro group and the plane of the ring, dONCC. The temporal evolution of the dONCC, shown in Fig. 3a, reports on the rapid torsion of the dihedral angle from near-planar ≈20° around time-zero to a near-perpendicular ≈100° after approximately 500 fs. Fig. 3a also shows a strong correlation between the temporal evolution of the experimentally retrieved dONCC angle and the increase of distances between 3.0 and 3.5 Å (peak γ) in the experimental ΔPDF. This observation strongly corroborates the assignment of peak γ to nitro group torsion and validates the use of GSFA retrieval in the interrogation of the nuclear dynamics of o-nitrophenol. The temporal evolution of the experimentally retrieved dONCC angle shows no evidence of nitro group re-planarization on our experimental time-window of 2 ps. Most importantly, our results provide unambiguous experimental evidence for the de-planarization of o-nitrophenol which has been hypothesized to facilitate internal conversion back to the ground state.
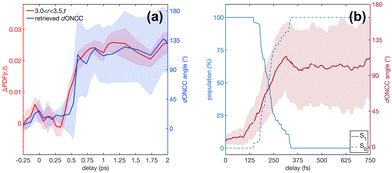 |
| Fig. 3 Panel (a) shows the experimental temporal evolution of the ΔPDF feature γ (red) overlaid onto the GSFA retrieved dONCC dihedral angle (blue). The shaded areas represent one standard deviation or the ΔPDF signal and one standard deviation across the ensemble of geometries retrieved. Panel (b) shows the temporal evolution of the dONCC dihedral angle (maroon) from AIMS simulations overlaid on the population of the S1 (solid light blue) and S0 states (dashed light blue). Shaded area represents one standard deviation of all computed trajectories. | |
Analysis of the temporal evolution of the dONCC angle in our AIMS simulations shows a strong correlation with the depopulation of the S1 state, shown in Fig. 3b. This observation is consistent with the existence of a S1/S0 conical intersection (CI) characterized by a 90-degree torsion of the HONO group as previously reported.20 It also suggests that dONCC torsion is required to close the energy gap between the S0 and S1(ππ*) states. The temporal evolution of the dONCC angle can, therefore, provide a rough estimate of the excited-state lifetime and timescale with which the nuclear wavepacket reaches the crossing seam and is able to access the S1/S0 CI. For simplicity, we consider the moment dONCC reaches 90 degrees to indicate viable access to the CI. In the AIMS simulations this coincides with 50% depopulation of the S1 state (see Fig. 3b). The temporal evolution of the experimentally retrieved dONCC suggests that the CI is accessed ≈550 fs post-photoexcitation. It is important to note that uncertainties in the determination of the experimental time-zero limit the precision of this estimation to ±100 fs. Nevertheless, our experimental data suggest a significant delay between photo-excitation and photo-relaxation. The capture of structural rearrangements consistent with internal conversion and the absence of UED signal consistent with photodissociation, i.e. HONO release (see ESI† Section 6 for a description of the UED signal for photodissociation) suggests that dissociation occurs on the electronic ground state and outside our experimental window of 2 picoseconds. Additional UED experiments on the nanosecond timescale are required to monitor HONO release. These studies do not need to use MeV electrons, as they do not require sub-picosecond temporal resolution.
Application of the same analysis criteria to the dONCC in our AIMS simulations shows that the CI is accessed in ≈250 fs. We attribute the ≈300 fs discrepancy between the timescale of CI access retrieved from the experimental UED data and that reported in our AIMS simulations to a combination of the experimental time-zero uncertainty mentioned above and inaccuracies in the electronic structure theory. Regarding the latter, FOMO-CASCI overestimates the S1(ππ*) state energy and thus predicts a steeper slope from the Franck–Condon region to the S1/S0 CI. Comparison of FOMO-CASCI and more accurate electronic structure methods including dynamic correlation is provided in Fig. S6 (ESI†) and Section 9 of the ESI.† We conclude that the difference in the simulated and measured timescales for dONCC torsion is likely within the theoretical and experimental error bars, although it would be of interest to decrease both the experimental and theoretical error bars in the future. Nevertheless, the simulations and experiment show the same key motions, albeit with a slightly different timescale.
We also note that the timescale for CI access captured in our UED experiment is significantly longer than those observed in previous spectroscopic measurements. For example, results from time-resolved photoelectron spectroscopy (TRPES) in o-nitrophenol optically excited with 350 nm light suggest access to the CI region occurs in 130 fs.20 TRPES, a technique which relies on photoionization to determine the lifetime of excited states, is particularly susceptible to artifacts induced by changes in ionization potential along the reaction coordinate. In cases where the ionization potential increases along the reaction coordinate the excited-state lifetime may appear artificially shortened. Computational exploration of the ionization potential of o-nitrophenol along the reaction coordinate towards internal conversion, presented in Fig. S7 of the ESI,† shows a significant increase in ionization potential as the wavepacket moves away from the Franck–Condon region, which could explain the differences in excited-state lifetime captured by TRPES and UED.
We now turn our attention to the analysis of the nuclear motions preceding de-planarization and CI access. Our AIMS simulations show that the de-planarization is preceded by intramolecular proton transfer between the hydroxyl (–OH) and nitro (–NO2) groups to form acid-nitro (HONO motif) in the 25 fs timescale. This observation is consistent with the barrierless IPT reaction proposed in previous works.21 In molecules with adjacent hydrogen donor and acceptor groups forming a hydrogen-bonded intramolecular H-chelate ring, proton transfer is often accompanied by changes in the molecular backbone. These motions can involve the alternation of single and double bonds41 and/or transient compression of the distance between the acceptor and donor atoms,42–44 where the latter dominates in the IPT reaction of o-nitrophenol. The transient compression of the donor–acceptor separation in the S1 state eliminates the energy barrier to the transfer of a proton present at the equilibrium donor–acceptor distance (see Fig. S8 in the ESI†). Our AIMS simulations show transient compression of the donor–acceptor separation, rOCON, from 2.55 and 2.37 Å, as depicted in Fig. 4b. Most importantly, simulations show that the moment of proton transfer, here defined as the time point at which the proton is equidistant from the hydroxyl oxygen and nitro oxygen atom, coincides with the moment that rOCON reaches its minimum value (≈2.35 Å). We, therefore, propose that rOCON can be used to interrogate the presence of nuclear motions consistent with IPT in o-nitrophenol. We do not expect the UED signal to capture the IPT reaction by resolving the distances involving the hydrogen atom being transferred (rOCH and rONH in Fig. 4a) as those produce weak scattering signals due to the low electron scattering cross section of hydrogen. Instead, we aim to infer the occurrence of IPT from structural changes in the molecular backbone. To this effect, we once again employ GSFA to retrieve the temporal evolution of the rOCON coordinate from the experimental signal directly. The experimentally retrieved rOCON separation, depicted in Fig. 4c, shows a transient 0.2 Å compression immediately after time-zero, which is qualitatively consistent with the IPT signature captured in the AIMS simulations (Fig. 4b). The transient loss of distances centered around 2.55 Å (the equilibrium rOCON) caused by the transient donor–acceptor compression which accompanies IPT is captured in the experimental ΔPDF as a slight bleach in the signal between 2.4 and 2.6 Å around time-zero (see Fig. 4c inset). Uncertainties in the time-zero determination and the limited instrument response (150 fs)26 prevent the experimental assignment of the exact IPT timescale. Nonetheless, our experimental results are qualitatively consistent with the fast barrierless IPT reported in the literature21 and captured by our AIMS simulations. The sudden increase in rOCON at ≈500 fs shown in Fig. 3c is a result of the HONO group torsion and deplanarization which enables the aci-nitro form to access the S1/S0 CI. Based on the experimentally retrieved temporal evolution of the rOCON separation and our assignment of IPT and CI signatures - transient compression and sudden increase, respectively - we report a delay of approximately 400 fs between IPT and CI access. We assign this surprisingly long delay to the substantial slowdown of the nuclear wavepacket as it traverses a region of the potential energy surface containing the excited-state [S1(ππ*)] minimum of the post-IPT aci-nitro. The optimized minimum-energy geometry of the S1(ππ*)-state aci-nitro isomer of o-nitrophenol is provided in Table S8 of the ESI† alongside potential energy surface scans along the reaction coordinate revealing the change in gradient responsible for the slowdown of the wavepacket (See Fig. S7, ESI† and Section 10 in the ESI† for more details on the potential energy surface scans). A molecular movie depicting the key nuclear motions during IPT, deplanarization and internal conversion back to the ground state has been compiled using the experimentally-retrieved structures and is shown as Movie 1 in the ESI.† This movie provides a qualitative representation of the dynamics captured in the UED data, with frames displaying the average structure at a given time determined by GSFA.
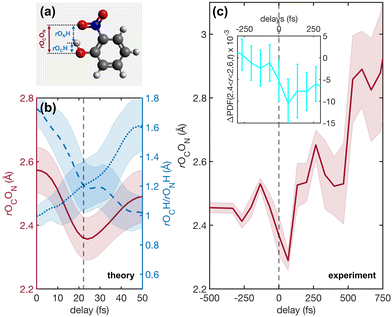 |
| Fig. 4 Panel (a) shows a schematic representation of three internal coordinates involved in the intramolecular proton-transfer of photo-excited o-nitrophenol: rOCON, rOCH and rONH. Panel (b) shows the temporal evolution of rOCON (solid maroon), rOCH (dotted light blue) and rONH (dashed light blue) from AIMS simulations. Panel (c) shows the temporal evolution of the experimentally retrieved rOCON. The shaded areas in panels (b) and (c) represent one standard deviation of all computed trajectories and one standard deviation across the ensemble of geometries retrieved by the genetic structural fitting algorithm, respectively. The inset in panel (c) shows the temporal evolution of the experimental ΔPDF signal between 2.4 and 2.6 angstrom. The moment of proton-transfer is represented by the vertical dashed grey lines in panels (b) and (c). | |
5. Conclusions
In summary, we have spatiotemporally resolved the key nuclear motions which allow o-nitrophenol to relax non-radiatively from the S1(ππ*) excited state to the S0 state. We have followed the formation of a de-planarized aci-nitro isomer of o-nitrophenol which enables access to the CI and internal conversion back to the ground-state ≈400 fs after photoexcitation. Moreover, we have experimentally resolved the transient compression of the donor–acceptor distance, a motion integral to the reaction coordinate for barrierless proton-transfer in nitrophenol. All experimentally retrieved motions were found to be in good qualitative agreement with those captured by AIMS simulations. The methodology described in this work, which leverages the ability of genetic structure-fitting algorithms to the retrieve subtle structural changes imperceptible from the standard analysis of the UED difference real-space signal, lays the framework for the study of more complex systems known to undergo intramolecular proton transfer, such as 2-(2′-hydroxyphenyl)benzothiazole (HBT)42,43,45 and 2-(2′-hydroxyphenyl)benzoxazole (HBO).46
Author contributions
JPFN and MC designed the experiment. JPFN prepared the experimental samples. JPFN, JY, IAZ, TJAW, BM, XS, ML, KH, RL, XJW and SW carried out the ultrafast electron diffraction experiment and collected the data. MW, RP, TJM performed the ab-initio multiple spawning simulations. CR performed potential energy surface scans. JPFN analysed the data with inputs from KW, TJM, TJAW, MW and MC. JPFN wrote the manuscript with input from all coauthors.
Conflicts of interest
There are no conflicts to declare.
Acknowledgements
The SLAC MeV UED facility is supported in part by the US Department of Energy, Office of Basic Energy Sciences, SUF Division Accelerator & Detector R&D program, the Linac Coherent Light Source Facility, and SLAC under contract no. DE-AC02-05-CH11231 and DE-AC02-76SF00515. JPFN, KW, BM and MC were funded through: AMOS program, Chemical Sciences, Geosciences, and Biosciences Division, Office of Basic Energy Sciences, Office of Science, US Department of Energy under grant no. DE-SC0014170. This work was supported by the AMOS program within the U. S. Department of Energy Office of Science, Basic Energy Sciences, Chemical Sciences, Geosciences and Biosciences Division.
Notes and references
- J. Kleffmann, Chem. Phys. Chem., 2007, 8, 1137–1144 CrossRef CAS PubMed.
- S.-B. Cheng, C.-H. Zhou, H.-M. Yin, J.-L. Sun and K.-L. Han, J. Chem. Phys., 2009, 130, 234311 CrossRef PubMed.
- S. Gligorovski, R. Strekowski, S. Barbati and D. Vione, Chem. Rev., 2015, 115, 13051–13092 CrossRef CAS PubMed.
- S. J. Formosinho and L. G. Arnaut, J. Photochem. Photobiol., A, 1993, 75, 21–48 CrossRef CAS.
- A. Douhal, F. Lahmani and A. H. Zewail, Chem. Phys., 1996, 207, 477–498 CrossRef CAS.
- N. Agmon, J. Phys. Chem. A, 2005, 109, 13–35 CrossRef CAS PubMed.
- T. J. Meyer, M. H. V. Huynh and H. H. Thorp, Angew. Chem., Int. Ed., 2007, 46, 5284–5304 CrossRef CAS PubMed.
- S. Hayashi, E. Tajkhorshid and K. Schulten, Biophys. J., 2002, 83, 1281–1297 CrossRef CAS PubMed.
- T. Kobayashi, T. Saito and H. Ohtani, Nature, 2001, 414, 531–534 CrossRef CAS PubMed.
- R. Y. Tsien, Annu. Rev. Biochem., 1998, 67, 509–544 CrossRef CAS PubMed.
- J. Zhao, S. Ji, Y. Chen, H. Guo and P. Yang, Phys. Chem. Chem. Phys., 2012, 14, 8803–8817 RSC.
- P. Chou, D. McMorrow, T. J. Aartsma and M. Kasha, J. Phys. Chem., 1984, 88, 4596–4599 CrossRef CAS.
- Z. Zhang, Y.-A. Chen, W.-Y. Hung, W.-F. Tang, Y.-H. Hsu, C.-L. Chen, F.-Y. Meng and P.-T. Chou, Chem. Mater., 2016, 28, 8815–8824 CrossRef CAS.
- J. E. Kwon and S. Y. Park, Adv. Mater., 2011, 23, 3615–3642 CrossRef CAS PubMed.
- B. Das, S. Dey, G. P. Maiti, A. Bhattacharjee, A. Dhara and A. Jana, New J. Chem., 2018, 42, 9424–9435 RSC.
- K. B. Borisenko, C. W. Bock and I. Hargittai, J. Phys. Chem., 1994, 98, 1442–1448 CrossRef CAS.
- M. Nagaya, S. Kudoh and M. Nakata, Chem. Phys. Lett., 2006, 427, 67–71 CrossRef CAS.
- I. Bejan, Y. Abd El Aal, I. Barnes, T. Benter, B. Bohn, P. Wiesen and J. Kleffmann, Phys. Chem. Chem. Phys., 2006, 8, 2028 RSC.
- M. Takezaki, N. Hirota and M. Terazima, J. Phys. Chem. A, 1997, 101, 3443–3448 CrossRef CAS.
- H. A. Ernst, T. J. A. Wolf, O. Schalk, N. González-García, A. E. Boguslavskiy, A. Stolow, M. Olzmann and A.-N. Unterreiner, J. Phys. Chem. A, 2015, 119, 9225–9235 CrossRef CAS PubMed.
- Y. Nitta, O. Schalk, H. Igarashi, S. Wada, T. Tsutsumi, K. Saita, T. Taketsugu and T. Sekikawa, J. Phys. Chem. Lett., 2021, 12, 674–679 CrossRef CAS PubMed.
- C. Xu, L. Yu, C. Zhu, J. Yu and Z. Cao, Sci. Rep., 2016, 6, 26768 CrossRef CAS PubMed.
- M. Sangwan and L. Zhu, J. Phys. Chem. A, 2016, 120, 9958–9967 CrossRef CAS PubMed.
- L. Vereecken, H. K. Chakravarty, B. Bohn and J. Lelieveld, Int.
J. Chem. Kinet., 2016, 48, 785–795 CrossRef CAS.
- S. P. Weathersby, G. Brown, M. Centurion, T. F. Chase, R. Coffee, J. Corbett, J. P. Eichner, J. C. Frisch, A. R. Fry, M. Gühr, N. Hartmann, C. Hast, R. Hettel, R. K. Jobe, E. N. Jongewaard, J. R. Lewandowski, R. K. Li, A. M. Lindenberg, I. Makasyuk, J. E. May, D. McCormick, M. N. Nguyen, A. H. Reid, X. Shen, K. Sokolowski-Tinten, T. Vecchione, S. L. Vetter, J. Wu, J. Yang, H. A. Dürr and X. J. Wang, Rev. Sci. Instrum., 2015, 86, 073702 CrossRef CAS PubMed.
- X. Shen, J. P. F. Nunes, J. Yang, R. K. Jobe, R. K. Li, M.-F. Lin, B. Moore, M. Niebuhr, S. P. Weathersby, T. J. A. Wolf, C. Yoneda, M. Guehr, M. Centurion and X. J. Wang, Struct. Dyn., 2019, 6, 054305 CrossRef CAS PubMed.
- M. Ben-Nun and T. J. Martínez, J. Phys. Chem. A, 1999, 103, 10517–10527 CrossRef CAS.
- M. Ben-Nun, J. Quenneville and T. J. Martínez, J. Phys. Chem. A, 2000, 104, 5161–5175 CrossRef CAS.
- J. Yang, X. Zhu, T. J. A. Wolf, Z. Li, J. P. F. Nunes, R. Coffee, J. P. Cryan, M. Gühr, K. Hegazy, T. F. Heinz, K. Jobe, R. Li, X. Shen, T. Veccione, S. Weathersby, K. J. Wilkin, C. Yoneda, Q. Zheng, T. J. Martinez, M. Centurion and X. Wang, Science, 2018, 361, 64–67 CrossRef CAS PubMed.
- T. J. A. Wolf, D. M. Sanchez, J. Yang, R. M. Parrish, J. P. F. Nunes, M. Centurion, R. Coffee, J. P. Cryan, M. Gühr, K. Hegazy, A. Kirrander, R. K. Li, J. Ruddock, X. Shen, T. Vecchione, S. P. Weathersby, P. M. Weber, K. Wilkin, H. Yong, Q. Zheng, X. J. Wang, M. P. Minitti and T. J. Martínez, Nat. Chem., 2019, 11, 504–509 CrossRef CAS PubMed.
- K. J. Wilkin, R. M. Parrish, J. Yang, T. J. A. Wolf, J. P. F. Nunes, M. Guehr, R. Li, X. Shen, Q. Zheng, X. Wang, T. J. Martinez and M. Centurion, Phys. Rev. A, 2019, 100, 023402 CrossRef.
- M. Centurion, T. J. A. Wolf and J. Yang, Annu. Rev. Phys. Chem., 2022, 73, 21–42 CrossRef CAS PubMed.
- K. Hegazy, V. Makhija, P. Bucksbaum, J. Corbett, J. Cryan, N. Hartmann, M. Ilchen, K. Jobe, R. Li, I. Makasyuk, X. Shen, X. Wang, S. Weathersby, J. Yang and R. Coffee, Commun. Phys., 2023, 6, 325 CrossRef CAS.
- J. G. Kim, S. Nozawa, H. Kim, E. H. Choi, T. Sato, T. W. Kim, K. H. Kim, H. Ki, J. Kim, M. Choi, Y. Lee, J. Heo, K. Y. Oang, K. Ichiyanagi, R. Fukaya, J. H. Lee, J. Park, I. Eom, S. H. Chun, S. Kim, M. Kim, T. Katayama, T. Togashi, S. Owada, M. Yabashi, S. J. Lee, S. Lee, C. W. Ahn, D.-S. Ahn, J. Moon, S. Choi, J. Kim, T. Joo, J. Kim, S. Adachi and H. Ihee, Nature, 2020, 582, 520–524 CrossRef CAS PubMed.
- J. Yang, X. Zhu, J. P. F. Nunes, J. K. Yu, R. M. Parrish, T. J. A. Wolf, M. Centurion, M. Gühr, R. Li, Y. Liu, B. Moore, M. Niebuhr, S. Park, X. Shen, S. Weathersby, T. Weinacht, T. J. Martinez and X. Wang, Science, 2020, 368, 885–889 CrossRef CAS PubMed.
- S. Habershon and A. H. Zewail, ChemPhysChem, 2006, 7, 353–362 CrossRef CAS PubMed.
- P. Slavíček and T. J. Martínez, J. Chem. Phys., 2010, 132, 234102 CrossRef PubMed.
- J. Finley, P.-Å. Malmqvist, B. O. Roos and L. Serrano-Andrés, Chem. Phys. Lett., 1998, 288, 299–306 CrossRef CAS.
- T. Korona and H.-J. Werner, J. Chem. Phys., 2003, 118, 3006–3019 CrossRef CAS.
- B. F. E. Curchod, C. Rauer, P. Marquetand, L. González and T. J. Martínez, J. Chem. Phys., 2016, 144, 101102 CrossRef PubMed.
- J. D. Coe, B. G. Levine and T. J. Martinez, J. Phys. Chem. A, 2007, 111, 11302–11310 CrossRef CAS PubMed.
- S. Lochbrunner, A. J. Wurzer and E. Riedle, J. Phys. Chem. A, 2003, 107, 10580–10590 CrossRef CAS.
- R. De Vivie-Riedle, V. De Waele, L. Kurtz and E. Riedle, J. Phys. Chem. A, 2003, 107, 10591–10599 CrossRef CAS.
- C. Schriever, M. Barbatti, K. Stock, A. J. A. Aquino, D. Tunega, S. Lochbrunner, E. Riedle, R. De Vivie-Riedle and H. Lischka, Chem. Phys., 2008, 347, 446–461 CrossRef CAS.
- M. Barbatti, A. J. A. Aquino, H. Lischka, C. Schriever, S. Lochbrunner and E. Riedle, Phys. Chem. Chem. Phys., 2009, 11, 1406–1415 RSC.
-
J. Seo, S. Kim, S. Park and S. Y. Park, Bull. Korean Chem. Soc.
Footnotes |
† Electronic supplementary information (ESI) available. See DOI: https://doi.org/10.1039/d3cp06253h |
‡ Present address: Diamond Light Source Ltd, Didcot, UK. |
§ Present address: Department of Engineering Physics, Tsinghua University, Beijing, China. |
|
This journal is © the Owner Societies 2024 |