P-incorporated CuO/Cu2S heteronanorods as efficient electrocatalysts for the glucose oxidation reaction toward highly sensitive and selective glucose sensing†
Received
25th August 2023
, Accepted 20th November 2023
First published on 6th December 2023
Abstract
Currently, tremendous efforts have been made to explore efficient glucose oxidation electrocatalysts for enzymeless glucose sensors to meet the urgent demands for accurate and fast detection of glucose in the fields of health care and environmental monitoring. In this work, an advanced nanostructured material based on the well-aligned CuO/Cu2S heteronanorods incorporated with P atoms is successfully synthesized on a copper substrate. The as-synthesized material shows high catalytic behavior accompanied by outstanding electrical conductivity. This, combined with the unique morphology of unstacked nanorod arrays, which endow the entire material with a greater number of exposed active sites, make the proposed material act as a highly efficient electrocatalyst for the glucose oxidation reaction. Density functional theory calculations demonstrate that P doping endows P-doped CuO/Cu2S with excellent electrical conductivity and glucose adsorption capability, significantly improving its catalytic performance. As a result, a non-enzymatic glucose sensor fabricated based on our proposed material exhibits a broad linear detection range (0.02–8.2 mM) and a low detection limit (0.95 μM) with a high sensitivity of 2.68 mA mM−1 cm−2 and excellent selectivity.
Introduction
Nowadays, development of highly efficient electrocatalysts for the glucose oxidation reaction toward achieving improved sensitivity and selectivity of non-enzymatic electrochemical glucose sensors is necessary for people diagnosed with diabetes, as well as for the fields of environment, pharmaceutical, clinical diagnosis, and quality control.1–4 Transition metal-based nanostructured materials with their unique features of promising redox behavior, sufficient mechanical/chemical stability, and cost-effectiveness have been widely employed in designing and constructing glucose oxidation electrocatalysts.5,6 In addition to the mono-compounds,7,8 recent research is paying attention to the development of multiple composite-based electrocatalysts, which contain two or more transition metal-based components, owing to double advantages. The composites can fully inherit not only the advantages of individual compounds, but also their synergy. For example, Y. Gao and co-workers reported a good electrocatalyst for glucose sensing based on needle-like Co3O4–NiO nanocomposites. The proposed materials showed a desirable sensing performance, including two wide linear ranges (0.001–3.4 mM, 3.4–12.2 mM), a low detection limit of 0.81 μM, and a high sensitivity of 62 mA M−1 cm−2. Interestingly, the composites outperformed their individual components, such as Co3O4 and NiO, in terms of catalytic activity due to the synergy of Co3O4 and NiO.9 Similarly, a tremella-like NiS/CoS/NiCo2S4 hierarchical structure, as an advanced electrocatalytic material, has been developed for a non-enzymatic glucose sensor. The high performance of the proposed material for glucose oxidation could be attributed to its superior catalytic activity and good electrical conductivity.10 Among the transition metals that have been developed, copper and its compounds attracted much attention for the sensing applications, especially in the field of glucose detection, owing to their multiple oxidation states and good catalytic activities.11 Particularly, copper oxide (CuO) with a large specific surface area for forming a large number of accessible catalytic active sites, desirable catalytic properties, and decent potential for accelerating charge transfer has emerged as an interesting candidate.12–14 In addition, copper sulfide (Cu2S) with the advantage of its redox nature (Cu2+/Cu3+), which is responsible for its great capability in electron transfer, biocompatibility, and high catalytic activity, has also been considered as a promising electrocatalyst for glucose oxidation.1,15,16 Accordingly, the combination of CuO and Cu2S to form a CuO/Cu2S composite is expected to catalyze the glucose oxidation reaction well toward achieving improved performance for glucose sensing. However, the low electrical conductivity of both CuO and Cu2S is still a bottleneck for the entire CuO/Cu2S composite, and thus a further improvement of CuO and Cu2S conductivities is essential. In this regard, doping heteroatoms into CuO and Cu2S matrixes offers an ideal solution. In recent years, numerous attempts have been made to boost the conductivity of CuO and Cu2S by doping, for example Cl-doped CuO,17 S-doped Cu2O–CuO,17 W-doped CuS,18 and In3+-doped CuS.19 Amongst the dopants, phosphorus (P) has been considered an effective dopant.20,21 This is because that the lone-pair electrons in 3p orbitals and vacant 3d orbitals of the P atom can regulate the electronic structure of the host materials to boost their intrinsic properties. Nevertheless, to the best of our knowledge, incorporating P atoms into the CuO/Cu2S composite has not been studied yet in the field of glucose detection. Herein, an advanced nanostructured material based on P-doped CuO/Cu2S heteronanorods grown on copper foam (CF) (denoted as P-CuO/Cu2S h-NRs) is developed, analysed, and employed as a highly efficient electrocatalyst for glucose oxidation toward non-enzymatic glucose sening applications. The experimental results show that the catalytic performance of the P-CuO/Cu2S h-NRs catalyst for glucose sensing is better than that of its mono-counterparts: CuO nanorods (denoted as CuO NRs) and CuO/Cu2S heteronanorods without P incorporation (denoted as CuO/Cu2S h-NRs), and its parent: Cu(OH)2 nanorods (denoted as Cu(OH)2 NRs). This can be attributed to the enhanced electrical conductivity facilitated by P doping, more exposed active sites due to the unstacked nanorod structure, the full inheritance of excellent advantages of individual components CuO and Cu2S, and the synergy of the CuO/Cu2S composite. Notably, density functional theory (DFT) calculations reveal that density of states (DOS) of the P-CuO/Cu2S model extends over the Fermi level, suggesting its good charge transfer capability, which is in line with the experimental results. Also, the DFT calculations prove that the P doping endows P-CuO/Cu2S with optimal glucose adsorption capability, supported by more negative adsorption energy of P-CuO/Cu2S (−1.470 eV) than that of the pure CuO/Cu2S (−1.173 eV).
Experimental
Sample preparation
Firstly, we synthesize a Cu(OH)2 NR sample via the chemical oxidation method.22 CF (size 5 cm × 5 cm) was successively sonicated with acetone, ethanol, and acetic acid for 15 min each to assure the removal of surface impurities. Then, the cleaned CF was dipped in 100 mL of the reactant solution (NaOH/(NH4)2S2O8 (weight ratio: 4/1) and deionized (DI) water) to deposit Cu(OH)2 NRs over its surface. After the surface of the CF substrate was fully wrapped with a light blue Cu(OH)2 NR layer, the product was taken out, washed with DI water, and dried for 12 h at 60 °C. Secondly, the obtained Cu(OH)2 NR sample was subject to sulfidation by dipping in 0.1 M thiourea solution at 90 °C for 5 h. Finally, a thermal treatment was conducted for the obtained composite at 300 °C for 2 h in the presence of 0.5 mg of NaH2PO2, as the P source, to form a P-CuO/Cu2S h-NR sample. The controlled samples, such as CuO NRs and CuO/Cu2S h-NRs were fabricated using the same approach, and for the details of the synthesis protocols, see the ESI.†
Characterization
The morphological structure of catalysts was investigated by field-emission scanning electron spectroscopy (FE-SEM) using a Sigma/Carl Zeiss microscope. The microstructure data of the catalyst were obtained through transmission electron microscopy (TEM) and high-resolution TEM (HR-TEM) by using a JEOL-2100F electron microscope. Furthermore, we investigated the crystalline phase structure of catalysts through X-ray diffraction (XRD) analysis using a Benchtop X-ray powder diffractometer – a D2 phaser accompanied by Cu-Ka radiation (λ = 1.5406 Å).
Catalytic activity test
The catalytic activity test was performed on a GHI 830B electrochemical analyser (CH instruments) using a typical three-electrode configuration: Ag/AgCl, a Pt plate, and the as-synthesized material (working area: 1 cm × 1 cm) as the reference electrode, counter electrode, and working electrode, respectively, at room temperature. Cyclic voltammetric (CV) measurement was conducted at a scan rate of 50 mV s−1 in an electrolyte containing 0.1 M NaOH solution + 1 mM glucose to examine electrocatalytic properties for glucose oxidation. Electrochemical impedance spectroscopy (EIS) was also performed at open circuit potential in the frequency range from 0.01 Hz to 500 kHz in the same electrolyte solution to study charge transfer ability. Regarding the glucose detection test, chronoamperometric measurements were conducted by successive addition of various amounts of glucose to 0.1 M NaOH solution.
Results and discussion
Characterization of the samples
SEM and elemental analyses.
The synthesis procedure of the P-CuO/Cu2S h-NRs sample is illustrated in Scheme 1. At first, Cu(OH)2 nanorod arrays were in situ deposited on the CF surface by the chemical oxidation method; then the sulfidation was performed to partially convert Cu(OH)2 into a Cu2S phase by dipping it in thiourea solution at 90 °C; the product was finally annealed with NaH2PO2 to form P-doped CuO/Cu2S heteronanorod arrays anchored on the CF framework. The morphological studies of the as-synthesized samples were conducted by SEM. The SEM image of Cu(OH)2 NRs in Fig. S1b (ESI†) obviously visualizes the uniform deposition of the Cu(OH)2 nanorod arrays on CF without any agglomeration phenomenon. Such an unstacked nanorod structure can be anticipated to provide a large surface area with a high density of exposed catalytic active sites and promote electrolyte diffusion/penetration to extend the contact with internal active centres.23,24 The enlarged SEM image in Fig. S1b (ESI†) reveals that the Cu(OH)2 nanorods have smooth surfaces, and their average diameter is around ∼215 nm. The SEM images of the P-CuO/Cu2S h-NRs electrocatalyst are shown in Fig. 1a–c. The P-CuO/Cu2S h-NRs sample largely inherits the shape and morphological features from its parent Cu(OH)2 NRs, meaning that P-CuO/Cu2S h-NRs possesses all structural advantages of Cu(OH)2 NRs, including the feature of unstacked nanorods for enlarging contact between the catalyst and the electrolyte, and a one-dimensional nanostructure for accelerating charge transfer.25 Thus, the partial sulfidation and P doping processes do not affect the morphology of the catalyst. For comparison, the CuO/Cu2S h-NRs in the absence of P heteroatom doping were prepared and investigated by SEM. As seen in Fig. S2 (ESI†), like the Cu(OH)2 NR precursor, the CuO/Cu2S h-NRs sample shows uniform growth of nanorod arrays over the surface of the CF support; in the enlarged SEM image (Fig. S2b, ESI†), the individual CuO/Cu2S nanorod also exhibits the one-dimensional feature with smooth surfaces.
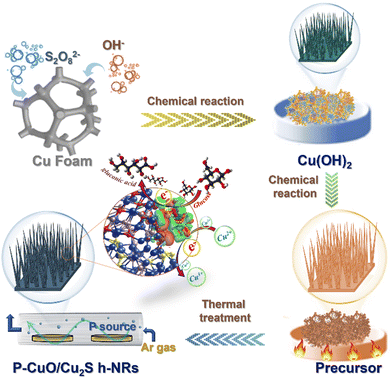 |
| Scheme 1 Schematic illustration of the fabrication of the P-CuO/Cu2S h-NRs sample. | |
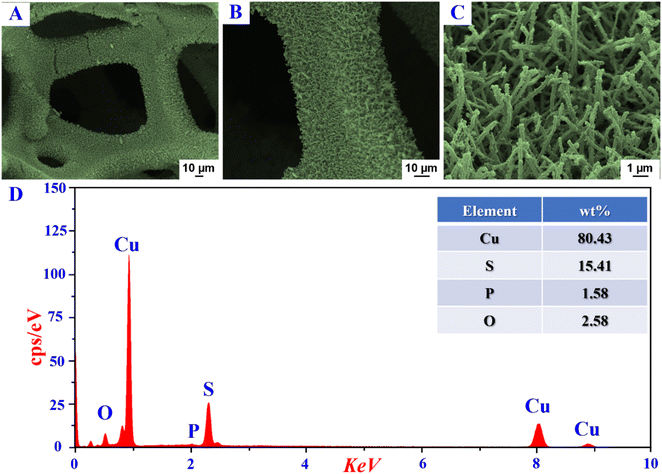 |
| Fig. 1 (a)–(c) SEM images at different magnifications and (d) EDS pattern of P-CuO/Cu2S h-NRs. | |
As compared with the CuO/Cu2S h-NRs, the P-CuO/Cu2S h-NRs still retained the rod-like morphology accompanied by its non-agglomeration feature even after treating under harsh conditions of phosphorization, once again confirming the non-influence of the P incorporation process on the morphological structure. Thereafter, chemical compositions of Cu(OH)2 NRs, CuO/Cu2S h-NRs, and P-CuO/Cu2S h-NRs were studied by energy dispersive X-ray spectroscopy (EDS). The EDS spectrum of Cu(OH)2 NRs (Fig. S3a, ESI†) shows the homogenous distribution of Cu and O elements, while the EDS spectrum of CuO/Cu2S h-NRs (Fig. S3b, ESI†) demonstrates the presence of Cu, O, and S elements. Regarding the P-CuO/Cu2S h-NRs (Fig. 1d), in addition to the Cu, O, and S elements, a very weak signal of the P element is observed in the EDS spectrum, as solid evidence for the successful incorporation of P atoms into the CuO/Cu2S composite. Inductively coupled plasma-optical emission spectrometry (ICP-OES) measurement was also conducted to quantify the actual content of elements in the P-CuO/Cu2S h-NRs material. The obtained results shown in Fig. S4 (ESI†) indicate that P-CuO/Cu2S h-NRs contained 82.64 wt% of Cu, 14.38 wt% of S, and 1.11 wt% of P, which are consistent with the EDS analysis results in shown in the inset of Fig. 1d.
TEM.
The details of the microstructure of the P-CuO/Cu2S h-NRs sample were obtained by using TEM measurement. The TEM results shown in Fig. 2a and b are in line with the SEM results, which clearly show the one-dimensional nanorod structure of the individual P-CuO/Cu2S nanorod. The HR-TEM images in Fig. 2c and d display two different kinds of lattice fringes. One lattice fringe has a distance of 0.22 nm (Fig. 2c), which should be assigned to the (111) plane of CuO, while the distance of another lattice fringe is 0.18 nm corresponding to the (220) plane of Cu2S (Fig. 2d). It is worth noting that the distance of lattice fringes belongs to the (111) plane of CuO and the (220) plane of Cu2S phases within the P-CuO/Cu2S h-NRs structure is smaller than those of the pure CuO (0.25 nm)26 and pure Cu2S (0.19 nm)27 reported in previous studies, suggesting that the doping of P slightly alters the crystalline structure of CuO and Cu2S. Indeed, it is demonstrated that the replacement of S and O atoms belonging to CuO and Cu2S, respectively, with smaller-diameter P atoms,28 leads to a slight lattice shrinkage.29–31 TEM-EDS mapping images of P-CuO/Cu2S h-NRs (Fig. 2e–h) reveal that the Cu, S, and O elements mix well within the composite; furthermore, the P element is uniformly incorporated into CuO/Cu2S. The lower density of P than those of the other elements reconfirms the small amount of the P dopant, which is in accordance with the SEM-EDS observations. Likewise, the corresponding EDS line scans of the P-CuO/Cu2S h-NRs sample (Fig. 2i) also exhibit the homogenous distribution of these four elements, corroborating the coexistence of CuO and Cu2O phases, as well as the successful incorporation of P into the CuO/Cu2S composite.
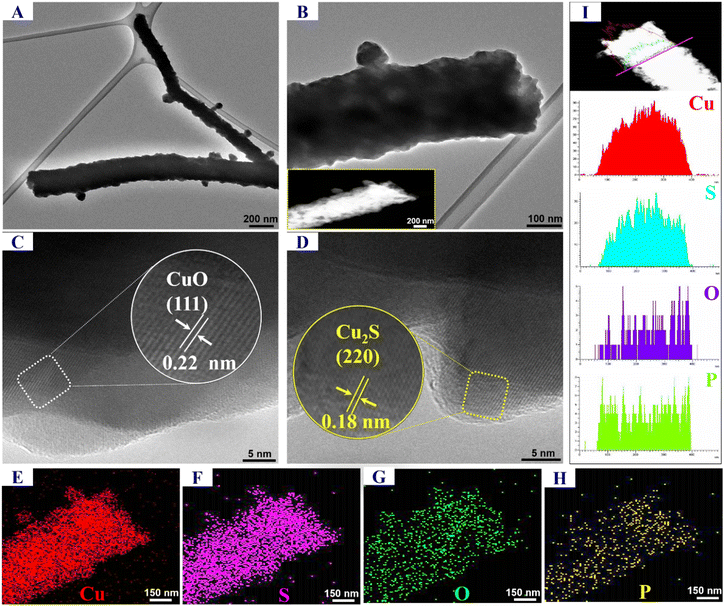 |
| Fig. 2 (a) and (b) TEM images at different magnifications, (c) and (d) HR-TEM images, and (e)–(h) EDS mapping images of P-CuO/Cu2S h-NRs (inset of (e): the STEM image of P-CuO/Cu2S h-NRs). | |
XRD.
Fig. 3a shows the XRD pattern of the P-CuO/Cu2S h-NRs material, which reveals two different kinds of diffraction peaks belonging to the CuO and Cu2S phases. The diffraction peaks corresponding to the monoclinic structured CuO (JCPDS 00-048-1548) are observed at 2-theta of 35.4, 35.5, 38.7, and 48.7° that can be attributed to the (002), (11−1), (111) and (20−2) phases,32 respectively, whereas the other diffraction peaks centred at 2-theta of 27.4, 31.8, 45.5 and 54.0° are well in line with the (111), (200), (220), and (311) planes of the cubic structure of the standard Cu2S (JCPDS 01-084-1770).33 Apart from the diffraction peaks of CuO and Cu2S, no impurity peaks can be observed from the XRD pattern of P-CuO/Cu2S h-NRs, suggesting the high purity of the P-CuO/Cu2S h-NRs material produced by our effective synthetic method. It is worth noting that the diffraction peak belonging to the CuO phase of P-CuO/Cu2S h-NRs at 2-theta of 35.5° has a slightly positive shift compared with the standard peak, as shown in Fig. S5a (ESI†). Likewise, we also found that the diffraction peak of the Cu2S phase at 2-theta of 45.5° in the P-CuO/Cu2S h-NRs material positively shifts in comparison with the Cu2S standard peak (Fig. S5b, ESI†). These phenomena are evidence for the successful integration of P to CuO/Cu2S. Because the ionic radius of P ions (0.044 nm) is smaller than those of the O ions (0.135 nm) and S ions (0.184 nm),28 the partial replacement of O and S with P leads to a lattice shrinkage and thereby causing a shift in the direction of higher angles of CuO and Cu2S diffraction peaks,34 which is in accordance with the HR-TEM observations. According to the XRD results, the crystal structures of CuO and Cu2S are determined and illustrated in Fig. 3b and c, respectively.
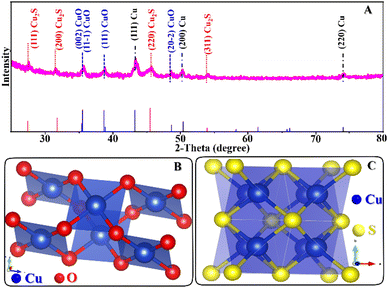 |
| Fig. 3 (a) XRD pattern of P-CuO/Cu2S h-NRs. (b) and (c) The crystal structure of CuO and Cu2S, respectively. | |
X-Ray photoelectron spectroscopy (XPS).
We carried out XPS measurement for P-CuO/Cu2S h-NRs and CuO/Cu2S h-NRs samples to determine their chemical composition and the valence state of the elements. The XPS survey spectra in Fig. S6a (ESI†) reveal the presence of Cu, S, and O elements in the CuO/Cu2S h-NRs sample, while the P-CuO/Cu2S h-NRs has the P element in addition to these elements, which is in line with the EDS results. The high-resolution XPS spectrum of Cu 2p in CuO/Cu2S h-NRs (Fig. S6b, ESI†) proves the formation of Cu+ belonging to Cu2S (peaks at binding energies of 932.8 and 952.2 eV) and Cu2+ arising from CuO (peaks at binding energies of 933.7 and 953.8 eV). Two additional satellite peaks centered at binding energies of 940.0 and 950.0 eV reconfirm the existence of Cu2+ in these samples.35 Importantly, the binding energies of Cu peaks are lower in the case of the P-CuO/Cu2S h-NRs (Fig. S6b and c, ESI†), which can be attributed to the modified electronic structure of Cu species due to the effect of P doping, resulting in a significantly improved catalytic performance.36 It is also worth noting that compared with those of pure CuO (the peaks of Cu2+ 2p3/2 and Cu2+ 2p1/2 at 933.8 and 954 eV, respectively)37 and pure Cu2S (the peaks of Cu+ 2p3/2 and Cu+ 2p1/2 at 932.8 and 952.1 eV, respectively),35 the shifts in binding energies are observed in the CuO/Cu2S h-NRs, indicating a strong electronic interaction between CuO and Cu2S that is of great significance to charge transfer toward boosting the electrochemical process.38 Thus, the XPS data demonstrate the decisive role of P doping and heterostructure in endowing the P-CuO/Cu2S h-NRs with an optimal electronic structure, which can promote both the reaction kinetics and intrinsic catalytic activity. Fig. S6d (ESI†) shows the S 2p XPS spectra of P-CuO/Cu2S h-NRs and CuO/Cu2S h-NRs. The peaks at binding energies of 160.8 and 162.0 eV correspond to S 2p3/2 and S 2p1/2 of Cu2S, respectively,39 reconfirming the presence of the Cu2S phase in these samples. On the other hand, the O 1s XPS spectra of P-CuO/Cu2S h-NRs and CuO/Cu2S h-NRs (Fig. S6e, ESI†) exhibit the two deconvoluted peaks of O 1s, including a peak located at a binding energy of 529.7 eV originating from lattice O of the Cu–O bond, evidencing the formation of a CuO phase, and another at a binding energy of 531.5 eV corresponding to the adsorbed O of OH– groups on the material surface.40 The successful incorporation of P atoms into CuO/Cu2S h-NRs is highlighted by the P 2p orbital (Fig. S6f, ESI†). The three deconvoluted P 2p peaks at binding energies of 130.3, 130.9, and 133.4 eV arose from P 2p3/2, P2p1/2, and P–O bonding owing to inevitable oxidation, respectively.41,42
Electrocatalytic performance
Catalytic effect of the products on the glucose oxidation reaction.
To examine the electrochemical properties of the P-CuO/Cu2S h-NRs for oxidation of glucose, we performed the CV measurement at a scan rate of 50 mV s−1 under alkaline conditions. The catalytic activities toward glucose oxidation of the control samples, such as Cu(OH)2 NRs, CuO NRs, and CuO/Cu2S h-NRs, were also investigated under the same conditions as reference points. Fig. 4a–d show the CV curves of Cu(OH)2 NR, CuO NR, CuO/Cu2S h-NRs, and P-CuO/Cu2S h-NRs catalysts in the 0.1 M NaOH solution without the addition of glucose (namely the blank electrolyte) and with the addition of 1 mM glucose (namely the glucose electrolyte). As seen, the CV curve of the Cu(OH)2 NR sample has only a large oxidation peak, reflecting an irreversible oxidation process. This can be assigned to the oxidation peaks of Cu2+ and Cu3+, accompanied by water electrolysis.43,44 Apart from the CV curve of Cu(OH)2 NRs, all CV curves of CuO NRs, CuO/Cu2S h-NRs, and P-CuO/Cu2S h-NRs exhibit a pair of broad redox peaks, which are consistent with the results reported in previous studies related to Cu-based nanostructured materials.2 A slight difference in the CV curves of these samples is due to their current response. The higher current response of CuO/Cu2S h-NRs than that of the CuO NRs suggests its better catalytic activity, which can be attributed to the formation of a heterostructure of CuO and Cu2S. However, the highest current response of P-CuO/Cu2S h-NRs confirms its best catalytic performance that is due to the heterostructure and P doping. Interestingly, once adding 1 mM glucose to the blank electrolyte, the oxidation peak currents of all Cu(OH)2 NR, CuO NR, CuO/Cu2S h-NRs, and P-CuO/Cu2S h-NRs materials are enhanced, proving that the glucose oxidation reaction may occur over the surface of these materials. From the CV curves, to further evaluate the catalytic activities of these products, we calculated their catalytic current response at various applied potentials, including 0.20 V to 0.40 V vs. Ag/AgCl, according to the following equation:where Ic, Ig, and Ib represent the catalytic current response, the oxidation peak current in the glucose electrolyte, and the oxidation peak current in the blank electrolyte, respectively. The results in Fig. 4e show that both the Cu(OH)2 NRs and CuO NRs have inferior catalytic properties for glucose oxidation, supported by their rather low catalytic current response, such as only 1.12 and 1.83 mA at 3.25 V, respectively. Regarding CuO/Cu2S h-NRs, this heterostructure shows higher catalytic currents than those of Cu(OH)2 NRs and CuO NRs at the same potential, such as 3.15 mA at 3.25 V, indicating its better catalytic activity, which can be ascribed to the synergy of CuO and Cu2S components. To further evaluate the role of the heterostructure in catalytic performance, we compared P-CuO/Cu2S h-NRs with the control samples, including P-doped CuO NRs, P-doped Cu2S NRs, and P-doped Cu(OH)2 NRs (Fig. S7, ESI†). As shown, all CV curves of the P-doped CuO NR, P-doped Cu2S NR, and P-doped Cu(OH)2 NR samples show the redox peaks in the potential range from 0.2 to 0.6 V, which are consistent with the CV results of P-CuO/Cu2S h-NRs and the previously reported Cu-based catalytic materials for glucose oxidation as mentioned above. However, the current response of the P-doped CuO NRs, P-doped Cu2S NRs, and P-doped Cu(OH)2 NRs is much lower than that of P-CuO/Cu2S h-NRs (Fig. S7d, ESI†), meaning their lower catalytic performance for the oxidation of glucose. This is because P-CuO/Cu2S h-NRs has mixed CuO/Cu2S possessing all advantages of CuO, Cu2S, and their synergistic effects, while the others are single compounds. In addition to the heterostructure, the incorporation of P into CuO/Cu2S h-NRs leads to a remarkable improvement of catalytic performance; the obtained P-CuO/Cu2S h-NRs exhibits the highest catalytic current response among four developed samples at the same potential, such as 5.05 mA at 3.25 V, demonstrating its highest catalytic performance for glucose oxidation. One of the reasons why the P-CuO/Cu2S h-NRs sample possesses advanced electrocatalytic behaviour is clarified through the investigation of its charge transfer ability by EIS measurement. For comparison, its counterparts (Cu(OH)2 NRs, CuO NRs, and CuO/Cu2S h-NRs) were also investigated by ESI measurement under the same conditions. Fig. 4f presents the Nyquist plots of the products; obviously, P-CuO/Cu2S h-NRs shows the smallest semicircle, meaning its most favourable charge transfer kinetics for the glucose oxidation process. Through fitting the Nyquist plots by an equivalent circuit containing charge transfer resistance (Rct), double layer capacitance (C), Warburg resistance (W), and series resistances (Rs) (inset of Fig. 4f), the Rct values are found to be 19.23, 16.46, 12.37, and 9.82 Ω for Cu(OH)2 NRs, CuO NRs, CuO/Cu2S h-NRs, and P-CuO/Cu2S h-NRs, respectively (Fig. S8, ESI†). The high Rct values of Cu(OH)2 NRs and CuO NRs alone imply the inefficient transfer of electrons within these structures, while the lower Rct value of CuO/Cu2S h-NRs suggests its superior electrical conductivity. This confirms that integrating Cu2S and CuO can accelerate electron transfer to catalytic active sites toward promoting kinetics of electrochemical reactions taking place on the surface of the CuO/Cu2S h-NRs electrocatalyst. As expected, P-CuO/Cu2S h-NRs has the smallest Rct value, confirming that the proposed material possesses the best electrical conductivity, which is consistent with its best catalytic activity mentioned above. This is because of the fact that the partial substitution of S and O atoms for P dopant atoms causes both lattice distortion of Cu2S and CuO as observed from the HR-TEM and XRD results and the change in charge density of Cu centers, leading to the improved charge transfer capability of the entire P-CuO/Cu2S h-NRs.45 Therefore, combining CuO and Cu2S to form a CuO/Cu2S composite, which fully inherits not only the advantages of individual components but also their chemical coupling effect, and P doping into CuO and Cu2S to reduce the electron transfer barrier have great potential for the glucose oxidation reaction.
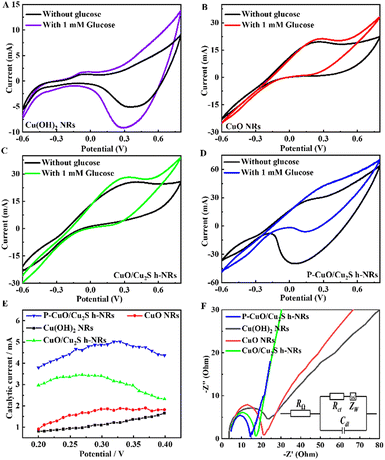 |
| Fig. 4 CV curves of (a) Cu(OH)2 NRs, (b) CuO NRs, (c) CuO/Cu2S h-NRs, and (d) P-CuO/Cu2S h-NRs without and with glucose. (e) The corresponding catalytic current responses of the products at different potentials. (f) EIS plots of the products (inset – an equivalent circuit model). | |
Catalytic effect of P-CuO/Cu2S h-NRs on the glucose oxidation reaction.
In addition to the advanced catalytic performance, the detection of glucose at different concentrations is an essential factor in evaluating how well the glucose oxidation catalysts perform in real applications. We used the CV measurement to determine the capability of P-CuO/Cu2S h-NRs in monitoring the different glucose concentrations. The CV curves of P-CuO/Cu2S h-NRs in 0.1 M NaOH medium with the addition of 0, 1, 2, 3, 4, 5, and 6 mM glucose at a constant scan rate of 50 mV s−1 are shown in Fig. 5a. It can be seen clearly that the oxidation peak current gradually enhances as the glucose concentration increases from 0 to 6 mM. Furthermore, the corresponding plot of peak current response vs. the glucose concentration (Fig. 5b) exhibits a good linear relationship between the peak current response and the glucose concentration. By linear fitting that plot, we found out a formula representing the relationship between the peak current and the glucose concentration as follows: | Ipo = 2.83 × CM + 13.93 | (2) |
where Ipo and CM are the oxidation peak current (μA) and the glucose concentration (mM), respectively. The R2 value is calculated to be 0.99. The obtained results make it clear that the P-CuO/Cu2S h-NRs catalyst for performing the glucose oxidation process has desirable efficiency at either low or high amounts of glucose in the electrolyte; in other words, the obtained results demonstrate that P-CuO/Cu2S h-NRs is greatly able to monitor glucose at different concentrations, which promotes the competitive potential of our proposed catalyst for practical applications. The CV measurement is also an effective method for analysing the reaction kinetics of glucose oxidation under the action of electrocatalysts.
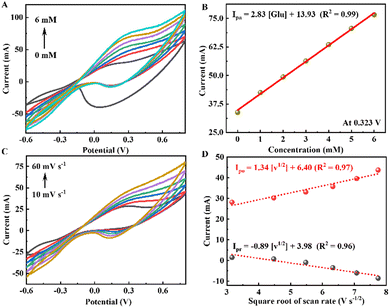 |
| Fig. 5 (a) CV curves of P-CuO/Cu2S h-NRs with different glucose concentrations. (b) The corresponding calibration plots between oxidation current response vs. glucose concentration. (c) CV curves of P-CuO/Cu2S h-NRs at different scan rates. (d) The corresponding plot between oxidation current response vs. square root of the scan rate. | |
In this work, we carried out the CV tests on the P-CuO/Cu2S h-NRs sample at different scan rates of 10, 20, 30, 40, 50, and 60 mV s−1 in the glucose electrolyte for the purpose of kinetic analysis. The CV curves in Fig. 5c reveal the rapid rising of the redox peak current response under greater applied potentials. Based on the obtained CV results, corresponding plots of oxidation and reduction peak current vs. the square root of the scan rates are constructed and presented in Fig. 5c, showing a good linear relationship. By linear fitting the plots, the relationship between the peak current and the square root is described according to the following formulas:
| Ipo = 1.34 × v1/2 + 6.40 | (3) |
| Ipr = −0.89 × v1/2 + 3.98 | (4) |
where
Ipo,
Ipr, and
v1/2 are the oxidation peak current (μA), the reduction peak current (μA), and the square root of the scan rate, respectively.
The R2 values are also determined to be 0.97 and 0.96 for the oxidation and reduction processes, respectively. The findings indicate that the catalytic oxidation of glucose taken place on the surface of the P-CuO/Cu2S h-NRs catalyst is governed by a diffusion-controlled mechanism.46
Glucose detection: sensitivity, selectivity, and stability.
Encouraged by the excellent catalytic properties of P-CuO/Cu2S h-NRs for the oxidation reaction of glucose, we used the as-prepared material for glucose sensing. It is well-known that catalysts should have high sensitivity and selectivity toward glucose to fulfil the requirements for enzymeless glucose sensing in real applications in addition to their superior catalytic activity. Consequently, the systematic tests of sensitivity and selectivity for P-CuO/Cu2S h-NRs were performed, and the results are shown in Fig. 6. Initially, we performed chronoamperometric measurement for P-CuO/Cu2S h-NRs at different applied potentials ranging from 0.0250 V to 0.400 V to determine the optimal working potential for glucose detection (Fig. 6a). Clearly, the current response for glucose oxidation increases as applied potential increases from 0.0250 V to 0.325 V; however, at a high applied potential of 0.350 V or higher (0.400 V), the responding current is reduced, proving that P-CuO/Cu2S h-NRs can deliver the highest current at an applied potential of 0.325 V. Thus, the optimal working potential for glucose detection with the help of the P-CuO/Cu2S h-NRs catalyst is found out to be 0.325 V. On the one hand, we subsequently carried out chronoamperometric measurement at that optimal working potential, along with the successive addition of different glucose concentrations to 0.1 M NaOH medium to explore the sensitivity, linear detection range, and limit of detection (LOD) value of the P-CuO/Cu2S h-NRs catalyst for glucose (Fig. 6b). Based on the findings, the calibration curve between the glucose concentration vs. oxidation current response is plotted, as shown in Fig. 6c. A broad linear detection range of 20 μM–8.2 mM is found for P-CuO/Cu2S h-NRs toward glucose. In that concentration region, a very high sensitivity of 2.68 mA mM−1 cm−2 is also calculated for P-CuO/Cu2S h-NRs. Next, the LOD value is determined according to the following equation:47where k = 3 (98.3% of confident level), sb reflects the standard deviation that is calculated based on CV measurement in the blank electrolyte, and m is the sensitivity. We found that the proposed P-CuO/Cu2S h-NRs catalyst possesses a high LOD value of 0.95 μM as well. The obtained results confirm that our proposed catalyst: P-CuO/Cu2S h-NRs has good sensitivity to glucose. More interestingly, P-CuO/Cu2S h-NRs outperforms other electrocatalysts based on the transition metals and their compounds for glucose oxidation reported elsewhere in terms of sensitivity and LOD values (Table S1, ESI†). Then, the sensitivity of P-CuO/Cu2S h-NRs was further evaluated by determining its response time by adding 1 mM glucose to 1.0 M NaOH solution during chronoamperometric testing. The control samples, including Cu(OH)2 NRs, CuO NRs, and CuO/Cu2S h-NRs, were also tested as reference points. Fig. S9 (ESI†) displays that the P-CuO/Cu2S h-NRs catalyst needs only ∼6 s to respond to the change of the glucose concentration, which is the shortest among those of the four synthesized materials, verifying its best glucose electrocatalytic behavior. On the other hand, the chronoamperometric measurement was also conducted for P-CuO/Cu2S h-NRs at an optimal working potential of 0.325 V, together with the successive addition of different analytes, apart from glucose, to 0.1 NaOH solution to evaluate its selectivity (Fig. 6d). In general, glucose is usually accompanied by inevitable substances, such as dopamine (DA), uric acid (UA), lactose, fructose, and KCl, in real applications, thus catalysts with excellent selectivity toward glucose are ideal for enzymeless glucose sensing.48 As expected, P-CuO/Cu2S h-NRs delivers a significant oxidation current response once adding glucose to the electrolyte. In contrast, the current responses delivered by P-CuO/Cu2S h-NRs when adding other analytes are minor. Indeed, it is found that the current responses of P-CuO/Cu2S h-NRs upon introduction of 10 μM uric acid (UA), 10 μM dopamine (DA), 10 μM Ascorbic Acid (AA), 10 μM Lactose (LA), 10 μM Fructose (FR), and 10 μM KCl are only 0.46, 1.35, 1.62, 2.51, 2.66, and 2.97% of its oxidation current response in the presence of glucose, as presented in the inset of Fig. 6d. Moreover, for the second glucose addition, the P-CuO/Cu2S h-NRs catalysts still deliver a high current response, which is comparable to the initial addition. The above findings suggest the superior selectivity of P-CuO/Cu2S h-NRs without the effect of coexisting substances on its catalytic performance for glucose detection, reconfirming the great potential of our proposed glucose electrocatalyst for practical applications.
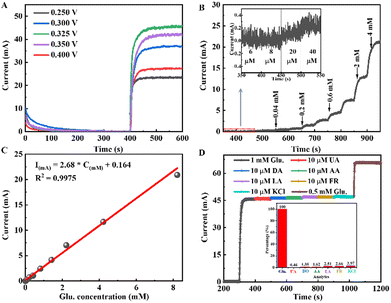 |
| Fig. 6 (a) Chronoamperometric curves of P-CuO/Cu2S h-NRs at different working potentials. (b) Chronoamperometric curve of P-CuO/Cu2S h-NRs with different glucose concentrations. (c) The corresponding calibration plot between the glucose concentration and oxidation current response. (d) Chronoamperometric curve of P-CuO/Cu2S h-NRs with the addition of different substances (inset – the corresponding responding currents). | |
Additionally, stability and durability are some of the most important criteria for the practicability of glucose catalysts. Hence, the stability measurement of P-CuO/Cu2S h-NRs for glucose oxidation was carried out by chronoamperometry in the glucose electrolyte (1 mM glucose + 0.1 M NaOH) at the optimal working potential of 0.325 V. The chronoamperometric curve in Fig. 7a displays that the current response of the P-CuO/Cu2S h-NRs catalyst is rather stable during chronoamperometric testing. The final current response is 92.9% of the initial one, even after sustained glucose oxidation for a long time of 10
000 s. The CV measurement at a scan rate of 50 mV s−1 in the glucose electrolyte is conducted to further investigate the stability of P-CuO/Cu2S h-NRs. According to the results in Fig. 7b, the CV curve at the 100th cycle almost overlaps with the initial one, suggesting the superior stability of the proposed catalyst. To further evaluate the stability of P-CuO/Cu2S h-NRs, we determined its peak catalytic currents versus cycle frequencies, including 1, 25, 50, 75, and 100 cycles. We found as shown in Fig. S10 (ESI†) that the oxidation current response of P-CuO/Cu2S h-NRs maintains 90.1% of the initial one after 100 successive cycles. These results demonstrate that the P-CuO/Cu2S h-NR catalyst is highly stable for glucose oxidation, which is beneficial for the long-term detection of glucose. Therefore, the findings mentioned above are strong evidence for the excellent catalytic activity of P-CuO/Cu2S h-NRs for glucose oxidation, promoting its potential for application in enzymeless glucose sensing, which meets all requirements of good sensitivity, high selectivity, and superior stability for real applications.
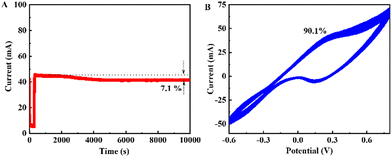 |
| Fig. 7 (a) Chronoamperometric curve of P-CuO/Cu2S h-NRs at a constant potential of 0.325 V for 10 000 s in the glucose electrolyte. (b) CV curves of P-CuO/Cu2S h-NRs for 100 cycles in the glucose electrolyte. | |
Theoretical study.
Finally, we carried out DFT calculations to gain more insights into glucose adsorption capability and the electronic properties of P-CuO/Cu2S h-NR, together with CuO/Cu2S h-NRs for comparison. The detailed computational method is provided in the ESI.† Initially, we calculated the adsorption energy (Eads) of glucose on CuO/Cu2S and P-CuO/Cu2S surfaces to find the active sites for the adsorption reaction. Here, glucose was placed on three surfaces: CuO, Cu2S, and the CuO/Cu2S interface. The calculated results are listed in Table S2 (ESI†). The glucose molecules have the most stable adsorption at the CuO/Cu2S interfaces with the most negative Eads. In contrast, CuO and Cu2S surfaces are inert to glucose absorption due to their almost negligible Eads values. Nobly, Fig. 8a and b reveal that P-doping results in more negative Eads of P-CuO/Cu2S compared to that of CuO/Cu2S (−1.470 eV vs. −1.173 eV), verifying that the glucose molecules are adsorbed more firmly on the P-CuO/Cu2S surface; in other words, P-CuO/Cu2S has better glucose adsorption capability than its counterpart without P doping. More importantly, the adsorption capability of P-CuO/Cu2S for glucose molecules even outperforms other electrocatalysts for glucose oxidation based on transition metal compounds reported in previous studies, such as CuS@Ni1Co2 layer double hydroxide with a glucose adsorption energy of −0.6 eV,41 basal Co4N with a glucose adsorption energy of −1.047 eV,42 and GNS-Co3O4 NGs with a glucose adsorption energy of −0.52 eV,43 highlighting that our developed material is a competitive catalyst for practical application in glucose sensors. P-doping also improves the glucose adsorption of CuO and Cu2S surfaces with Eads of −0.744 and −0.309 eV, although both are inert to glucose adsorption before P-doping. This evidence indicates that heterojunction formation and P-doping play essential roles in adsorption enhancement.
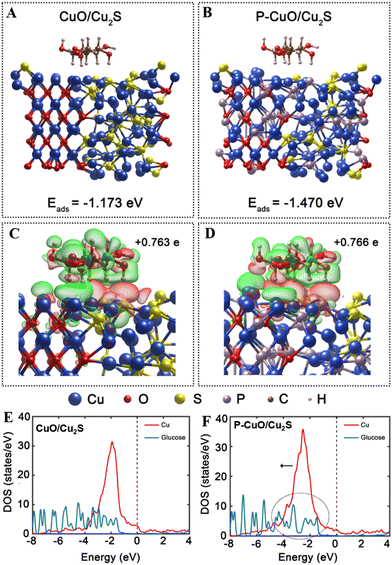 |
| Fig. 8 The optimized structure of (a) glucose-absorbed CuO/Cu2S and (b) glucose-absorbed P-doped CuO/Cu2S with adsorption energies (Eads). Differential charge density of (c) glucose-absorbed CuO/Cu2S and (d) glucose-absorbed P-doped CuO/Cu2S. Red and green isosurfaces indicate the charge accumulation and depletion, respectively. Partial DOS of (e) CuO/Cu2S and (f) P-CuO/Cu2S. Red and blue lines indicate Cu surface atoms and glucose, respectively. The Fermi level (EF) is set to zero. | |
Moreover, we calculated the differential charge density of glucose-absorbed P-CuO/Cu2S and glucose-absorbed CuO/Cu2S. The charge density map in Fig. 8c shows that after the adsorption of glucose on the surface of CuO/Cu2S, the electrons accumulate around the glucose molecules, suggesting that the adsorbed glucose can serve as a charge acceptor. After P doping, a similar phenomenon is observed from the charge density map of P-CuO/Cu2S, as seen in Fig. 8d. According to Bader charge analysis, the adsorbed glucose received +0.763 e and +0.766 e from CuO/Cu2S and P-CuO/Cu2S, respectively. In addition, Bader charge analysis was performed to find which elements in CuO/Cu2S and P-CuO/Cu2S are more likely to interact and form bonds with the glucose molecules. Table S3 (ESI†) shows the Bader charge transfer of each element to the glucose molecules in CuO/Cu2S and P-CuO/Cu2S. Cu transfers the most charge to glucose with an approximate value of 0.55 e−, implying that Cu is more likely to form bonds with the glucose molecules and plays a more critical role in glucose adsorption. To gain more understanding of the electronic structure of CuO/Cu2S before and after P-doping, the partial DOS for CuO/Cu2S and P-CuO/Cu2S models were calculated to compare and confirm their electronic properties (Fig. 8e and f). Here, only DOS of d orbitals of Cu surface atoms close to the glucose molecule are considered because P-doping only results in significant changes in the electronic structure of Cu. As shown in Fig. S11 (ESI†), the d-band center of CuO/Cu2S exhibits a downward shift below EF. In contrast, the p-band center of CuO/Cu2S is almost unchanged after P-doping, implying P-doping with changes in the electronic structure of Cu should play key roles in enhancing adsorption. In Fig. 8e and f, the downshift of Cu-d orbitals in P-CuO/Cu2S enhances the orbital overlapping between Cu and glucose, resulting in a stronger hybridization of electronic orbitals of Cu. This hybridization leads to stronger bonding and redistribution of electron density around the adsorption site. Consequently, a more favorable environment for glucose adsorption is created after P-doping. Besides, DOS P-CuO/Cu2S gets over the Fermi level and extends beyond the conduction band, indicating the good electrical conductivity for fast charge transport of P-CuO/Cu2S h-NR, which is consistent with the EIS results and recognized as one of the important reasons for the excellent glucose sensitivity of P-CuO/Cu2S h-NR.39,40 Therefore, the DFT calculations indicate that incorporating P into CuO/Cu2S boosts electron mobility for highly sensitive glucose detection and improves the glucose adsorption capability to enhance the reaction kinetics of glucose oxidation.
The reaction mechanism.
Fig. 9 illustrates the electrocatalytic mechanism of the P-CuO/Cu2S h-NRs catalyst for glucose oxidation. Previous studies demonstrated that the high valence state species in transition metal-based materials are real active centres toward glucose.16 The XPS data of P-CuO/Cu2S h-NRs confirms the coexistence of Cu+ and Cu2+ within this heterostructure. In an alkaline environment, Cu2+ is oxidized to Cu3+, which can act as active sites for oxidation of glucose.24 Remarkably, the XSP analysis also proves the strong electronic interactions between the CuO and Cu2S components of the heterostructure, as well as the effect of the P doping on the modulated electronic structure of Cu species, which is significantly beneficial to charge transfer ability and intrinsic activity of Cu sites in the entire P-CuO/Cu2S h-NRs materials. More interestingly, the DFT calculations verify that atoms at the heterointerface between CuO and Cu2S serve as active sites for glucose adsorption and oxidation. Because Cu at the heterointerface dominates the largest charge to form bonds with glucose molecules, these species are critical active sites in P-CuO/Cu2S h-NRs. The DFT results also show that the adsorption capability of Cu for glucose is considerably enhanced thanks to the introduction of P dopants. Therefore, all of the findings emphasize that P-doping and the heterostructure primarily contribute to the excellent catalytic activity of P-CuO/Cu2S h-NRs, and the Cu species at the heterointerface are the real active sites. Consequently, the electrocatalytic mechanism of glucose oxidation on the P-CuO/Cu2S h-NRs surface can be described as follows: (i) the first step is the chemical adsorption of glucose molecules on the surface of P-CuO/Cu2S h-NRs catalysts via C1–Cu3+ bonding, wherein Cu is located at the interface. (ii) In the second step, the adsorbed glucose is oxidized through a reaction of activated H species belonging to the HO− group of C1 and the HO− group of the electrolyte, forming gluconolactone. (iii) Finally, the gluconolactone molecule is desorbed from the catalyst surface and is simultaneously hydrolysed to generate gluconic acid. As anticipated, P-CuO/Cu2S h-NRs with an unstacked nanorod structure for exposing a large active surface area, accompanied by the excellent charge transfer ability for accelerating electron transfer to catalytic active sites, can effectively promote the glucose oxidation reaction.
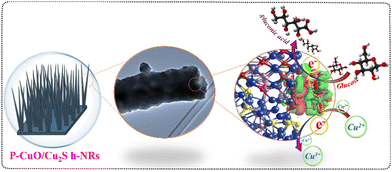 |
| Fig. 9 Schematic showing the proposed mechanism of the glucose oxidation process occurring on the surface of P-CuO/Cu2S h-NRs. | |
Conclusion
In summary, a P-CuO/Cu2S h-NR composite that contains P-doped well-aligned heterostructured CuO/Cu2S nanorods supported on three-dimensional copper foam has been successfully synthesized using a scalable approach. Benefiting from the quintessential structure of self-supporting one-dimensional nanorod arrays on the substrate without using an additive polymer binder for enlarging the contact area between the catalyst and the electrolyte/reactant molecules, promoting charge transfer to active centres that is more effective than that of other buck structures, and overcoming the negative effect of a polymer binder on catalytic performance, accompanied by the incorporation of P atoms into the CuO/Cu2S matrix, which brings a considerable improvement in the electrical conductivity of the entire material and glucose adsorption capability, the P-CuO/Cu2S h-NR catalyst shows excellent catalytic activity for glucose oxidation. Hence, P-CuO/Cu2S h-NR has desirable sensitivity, a broad linear detection range, a low LOD value, good selectivity, and extraordinary stability for glucose detection, which are favorable evidence for the great potential of our proposed catalyst for electrochemical determination of glucose in practical applications.
Conflicts of interest
There are no conflicts to declare.
Notes and references
- K. P. Sharma, M. Shin, G. P. Awasthi, M. B. Poudel, H. J. Kim and C. Yu, Chitosan polymer matrix-derived nanocomposite (CuS/NSC) for non-enzymatic electrochemical glucose sensor, Int. J. Biol. Macromol., 2022, 206, 708–717 CrossRef CAS PubMed.
- T. Yang, W. Zhang, J. Wu, S. Liu and Y. Zhao, Core-shell composite of CuS nanocages and NiCo layered double hydroxide nanosheets with modulated electron structure as “two birds with one Stone” glucose oxidation electrocatalysts, Appl. Surf. Sci., 2022, 600, 154205 CrossRef CAS.
- X. Ma, J. Chen, B. Yuan, Y. Li, L. Yu and W. Zhao, Three-dimensional hollow nickel phosphate microspheres with controllable hoya-like structure for high-performance enzymeless glucose detection and supercapacitor, Appl. Surf. Sci., 2022, 588, 152928 CrossRef CAS.
- L. He, J. Su, T. You, S. Xiao, Y. Shen, P. Jiang and D. He, Mn incorporation boosted NiO nanosheets as highly efficient anode for sensitive glucose detection in beverage, LWT, 2023, 182, 114807 CrossRef CAS.
- R. Gaur, S. Shahabuddin, N. Mukherjee and P. Chandra, Recent advances in nanostructured transition metal sulfide based sensors for environmental applications, Mater. Today Proc., 2022, 62, 6945–6949 CrossRef CAS.
- M. Huang, S. Feng, C. Yang, F. Wen, D. He and P. Jiang, Construction of an MnO2 nanosheet array 3D integrated electrode for sensitive enzyme-free glucose sensing, Anal. Methods, 2021, 13, 1247–1254 RSC.
- X. Wang, M. Wang, S. Feng, D. He and P. Jiang, Controlled synthesis of flower-like cobalt phosphate microsheet arrays supported on Ni foam as a highly efficient 3D integrated anode for non-enzymatic glucose sensing, Inorg. Chem. Front., 2020, 7, 108–116 RSC.
- M. Wang, X. Wang, S. Feng, D. He and P. Jiang, Amorphous Ni-P nanoparticles anchoring on nickel foam as an efficient integrated anode for glucose sensing and oxygen evolution, Nanotechnology, 2020, 31, 455503 CrossRef CAS PubMed.
- Y. Gao, Q. Yu, Y. Du, M. Yang, L. Gao, S. Rao, Z. Yang, Q. Lan and Z. Yang, Synthesis of Co3O4–NiO nano-needles for amperometric sensing of glucose, J. Electroanal. Chem., 2019, 838, 41–47 CrossRef CAS.
- D. Li, X. Zhang, L. Pei, C. Dong, J. Shi and Y. Xu, High-performance supercapacitors and non-enzymatic electrochemical glucose sensor based on tremella-like NiS/CoS/NiCo2S4 hierarchical structure, Inorg. Chem. Commun., 2019, 110, 107581 CrossRef CAS.
- M. Waqas, L. Wu, H. Tang, C. Liu, Y. Fan, Z. Jiang, X. Wang, J. Zhong and W. Chen, Cu2O Microspheres Supported on Sulfur-Doped Carbon Nanotubes for Glucose Sensing, ACS Appl. Nano Mater., 2020, 3, 4788–4798 CrossRef CAS.
- X. Wang, C. Hu, H. Liu, G. Du, X. He and Y. Xi, Synthesis of CuO nanostructures and their application for nonenzymatic glucose sensing, Sens. Actuators, B, 2010, 144, 220–225 CrossRef CAS.
- C. Batchelor-McAuley, Y. Du, G. G. Wildgoose and R. G. Compton, The use of copper(II) oxide nanorod bundles for the non-enzymatic voltammetric sensing of carbohydrates and hydrogen peroxide, Sens. Actuators, B, 2008, 135, 230–235 CrossRef CAS.
- Z. Zhuang, X. Su, H. Yuan, Q. Sun, D. Xiao and M. M. F. Choi, An improved sensitivity non-enzymatic glucose sensor based on a CuO nanowire modified Cu electrode, Analyst, 2008, 133, 126–132 RSC.
- Y. Fu and W. Jin, Facile synthesis of core-shell CuS–Cu2S based nanocomposite for the high-performance glucose detection, Mater. Sci. Eng. C, 2019, 105, 110120 CrossRef CAS PubMed.
- S. Radhakrishnan, H.-Y. Kim and B.-S. Kim, A novel CuS microflower superstructure based sensitive and selective nonenzymatic glucose detection, Sens. Actuators, B, 2016, 233, 93–99 CrossRef CAS.
- D. P. Jaihindh, P. Anand, R.-S. Chen, W.-Y. Yu, M.-S. Wong and Y.-P. Fu, Cl-doped CuO for electrochemical hydrogen evolution reaction and tetracycline photocatalytic degradation, J. Environ. Chem. Eng., 2023, 11, 109852 CrossRef CAS.
- J. Chen, H. Pan, Y. Chen, Z. Zhou, G. Jing and X. Zhao, Efficient activation of peracetic acid for abatement of tetracycline by W-doped CuS via regulating copper redox cycling, Chem. Eng. J., 2023, 464, 142693 CrossRef CAS.
- K. Vinotha, B. Jayasutha, M. J. Abel and K. Vinoth, In3+-doped CuS thin films: physicochemical characteristics and photocatalytic property, J. Mater. Sci. Mater. Electron., 2022, 33, 22862–22882 CrossRef CAS.
- Z. Xiao, Y. Wang, Y.-C. Huang, Z. Wei, C.-L. Dong, J. Ma, S. Shen, Y. Li and S. Wang, Filling the oxygen vacancies in Co3O4 with phosphorus: an ultra-efficient electrocatalyst for overall water splitting, Energy Environ. Sci., 2017, 10, 2563–2569 RSC.
- W. Yao, C. Tian, C. Yang, J. Xu, Y. Meng, I. Manke, N. Chen, Z. Wu, L. Zhan, Y. Wang and R. Chen, P-Doped NiTe2 with Te-Vacancies in Lithium–Sulfur Batteries Prevents Shuttling and Promotes Polysulfide Conversion, Adv. Mater., 2022, 34, 2106370 CrossRef CAS PubMed.
- S. Lv, J. Li, B. Zhang, Y. Shi, X. Liu and T. Wang, In-situ growth of hierarchical CuO@Cu3P heterostructures with transferable active centers on copper foam substrates as bifunctional electrocatalysts for overall water splitting in alkaline media, Int. J. Hydrogen Energy, 2022, 47, 9593–9605 CrossRef CAS.
- H.-C. Chen, Y.-C. Yeh and M.-H. Yen, Synthesis of Au or Ag/Cu2O/aluminum doped zinc oxide nanorods hybrid electrode for high sensitive non-enzymatic glucose sensor: Mechanism investigation of formation and surface plasmon resonance, Mater. Chem. Phys., 2022, 282, 125924 CrossRef CAS.
- M. Kuznowicz, T. Rębiś, A. Jędrzak, G. Nowaczyk, M. Szybowicz and T. Jesionowski, Glucose determination using amperometric non-enzymatic sensor based on electroactive poly(caffeic acid)@MWCNT decorated with CuO nanoparticles, Microchim. Acta, 2022, 189, 159 CrossRef CAS PubMed.
- Z. Li, J. Wu, L. Liao, X. He, B. Huang, S. Zhang, Y. Wei, S. Wang and W. Zhou, Surface engineering of hematite nanorods photoanode towards optimized photoelectrochemical water splitting, J. Colloid Interface Sci., 2022, 626, 879–888 CrossRef CAS PubMed.
- Y. Zhai, Y. Ji, G. Wang, Y. Zhu, H. Liu, Z. Zhong and F. Su, Controllable wet synthesis of multicomponent copper-based catalysts for Rochow reaction, RSC Adv., 2015, 5, 73011–73019 RSC.
- A. Singh, R. Manivannan and S. Noyel Victoria, Simple one-pot sonochemical synthesis of copper sulphide nanoparticles for solar cell applications, Arab. J. Chem., 2019, 12, 2439–2447 CrossRef CAS.
- R. D. Shannon, Revised effective ionic radii and systematic studies of interatomic distances in halides and chalcogenides, Acta Crystallogr., Sect. A: Cryst. Phys., Diffr., Theor. Gen. Crystallogr., 1976, 32, 751–767 CrossRef.
- M. Xiao, D. Han, X. Yang, N. Tsona Tchinda, L. Du, Y. Guo, Y. Wei, X. Yu and M. Ge, Ni-doping-induced oxygen vacancy in Pt-CeO2 catalyst for toluene oxidation: Enhanced catalytic activity, water-resistance, and SO2-tolerance, Appl. Catal., B, 2023, 323, 122173 CrossRef CAS.
- Y. Qin, F. Fang, Z. Xie, H. Lin, K. Zhang, X. Yu and K. Chang, La,Al-Codoped SrTiO3 as a Photocatalyst
in Overall Water Splitting: Significant Surface Engineering Effects on Defect Engineering, ACS Catal., 2021, 11, 11429–11439 CrossRef CAS.
- G. Wei, Z. Xu, X. Zhao, S. Wang, F. Kong and C. An, N-doped CoSe2 nanomeshes as highly-efficient bifunctional electrocatalysts for water splitting, J. Alloys Compd., 2022, 893, 162328 CrossRef CAS.
- C. Zhou, L. Xu, J. Song, R. Xing, S. Xu, D. Liu and H. Song, Ultrasensitive non-enzymatic glucose sensor based on three-dimensional network of ZnO-CuO hierarchical nanocomposites by electrospinning, Sci. Rep., 2014, 4, 7382 CrossRef CAS PubMed.
- M. Cao, H. Wang, P. Kannan, S. Ji, X. Wang, Q. Zhao, V. Linkov and R. Wang, Highly efficient non-enzymatic glucose sensor based on CuxS hollow nanospheres, Appl. Surf. Sci., 2019, 492, 407–416 CrossRef CAS.
- N. Le Minh Tri, D. Q. Trung, D. Van Thuan, N. T. Dieu Cam, T. Al Tahtamouni, T.-D. Pham, D. S. Duc, M. H. Thanh Tung, H. Van Ha, N. H. Anh Thu and H. T. Trang, The advanced photocatalytic performance of V doped CuWO4 for water splitting to produce hydrogen, Int. J. Hydrogen Energy, 2020, 45, 18186–18194 CrossRef CAS.
- Z. Han, G. Li, X. Zeng, Y. Zhu, N. Li, J. Zhang, W. Zhao and Q. Jiao, Electrochemical construction of Cu@NF frameworks and synthesis of self-supported microflower Cu2S@NF as bifunctional catalysts for overall water splitting, Int. J. Hydrogen Energy, 2022, 47, 15695–15705 CrossRef CAS.
- S. Huang, S. Ma, L. Liu, Z. Jin, P. Gao, K. Peng, Y. Jiang, A. Naseri, Z. Hu and J. Zhang, P-doped Co3S4/NiS2 heterostructures embedded in N-doped carbon nanoboxes: Synergistical electronic structure regulation for overall water splitting, J. Colloid Interface Sci., 2023, 652, 369–379 CrossRef CAS PubMed.
- S. Masudy-Panah, R. Siavash Moakhar, C. S. Chua, H. R. Tan, T. I. Wong, D. Chi and G. K. Dalapati, Nanocrystal Engineering of Sputter-Grown CuO Photocathode for Visible-Light-Driven Electrochemical Water Splitting, ACS Appl. Mater. Interfaces, 2016, 8, 1206–1213 CrossRef CAS PubMed.
- Y. Dang, G. Wang, G. Su, Z. Lu, Y. Wang, T. Liu, X. Pu, X. Wang, C. Wu, C. Song, Q. Zhao, H. Rao and M. Sun, Rational Construction of a Ni/CoMoO4 Heterostructure with Strong Ni–O–Co Bonds for Improving Multifunctional Nanozyme Activity, ACS Nano, 2022, 16, 4536–4550 CrossRef CAS PubMed.
- J. Wang, M. M. Rahman, C. Ge and J.-J. Lee, Electrodeposition of Cu2S nanoparticles on fluorine-doped tin oxide for efficient counter electrode of quantum-dot-sensitized solar cells, J. Ind. Eng. Chem., 2018, 62, 185–191 CrossRef CAS.
- T. Wang, X. Zhang, X. Zhu, Q. Liu, S. Lu, A. M. Asiri, Y. Luo and X. Sun, Hierarchical CuO@ZnCo LDH heterostructured nanowire arrays toward enhanced water oxidation electrocatalysis, Nanoscale, 2020, 12, 5359–5362 RSC.
- C. Liu, D. Jia, Q. Hao, X. Zheng, Y. Li, C. Tang, H. Liu, J. Zhang and X. Zheng, P-Doped Iron–Nickel Sulfide Nanosheet Arrays for Highly Efficient Overall Water Splitting, ACS Appl. Mater. Interfaces, 2019, 11, 27667–27676 CrossRef CAS PubMed.
- H. Lei, M. Chen, Z. Liang, C. Liu, W. Zhang and R. Cao, Ni2 P hollow microspheres for electrocatalytic oxygen evolution and reduction reactions, Catal. Sci. Technol., 2018, 8, 2289–2293 RSC.
- H. Sim, J.-H. Kim, S.-K. Lee, M.-J. Song, D.-H. Yoon, D.-S. Lim and S.-I. Hong, High-sensitivity non-enzymatic glucose biosensor based on Cu(OH)2 nanoflower electrode covered with boron-doped nanocrystalline diamond layer, Thin Solid Films, 2012, 520, 7219–7223 CrossRef CAS.
- L.-C. Jiang and W.-D. Zhang, A highly sensitive nonenzymatic glucose sensor based on CuO nanoparticles-modified carbon nanotube electrode, Biosens. Bioelectron., 2010, 25, 1402–1407 CrossRef CAS PubMed.
- K. Samdhyan, P. Chand and H. Anand, Effective doping of phosphorus in copper sulfide for high performance energy storage devices, J. Alloys Compd., 2023, 936, 168322 CrossRef CAS.
- X. Mei, Q. Wei, H. Long, Z. Yu, Z. Deng, L. Meng, J. Wang, J. Luo, C.-T. Lin, L. Ma, K. Zheng and N. Hu, Long-term stability of Au nanoparticle-anchored porous boron-doped diamond hybrid electrode for enhanced dopamine detection, Electrochim. Acta, 2018, 271, 84–91 CrossRef CAS.
- A. Saljooqi, T. Shamspur and A. Mostafavi, The MWCNT-Ag-PT GCE Electrochemical Sensor Functionalized With dsDNA for Mitoxantrone Sensing in Biological Media, IEEE Sens. J., 2019, 19, 4364–4368 CAS.
- D. Geng, X. Bo and L. Guo, Ni-doped molybdenum disulfide nanoparticles anchored on reduced graphene oxide as novel electroactive material for a non-enzymatic glucose sensor, Sens. Actuators, B, 2017, 244, 131–141 CrossRef CAS.
|
This journal is © the Owner Societies 2024 |
Click here to see how this site uses Cookies. View our privacy policy here.