DOI:
10.1039/D4CC03085K
(Feature Article)
Chem. Commun., 2024,
60, 11017-11033
Progress on critical cell fabrication parameters and designs for advanced lithium–sulfur batteries†
Received
25th June 2024
, Accepted 9th August 2024
First published on 12th August 2024
Abstract
Since 1990, commercial lithium-ion batteries have made significant strides, approaching their theoretical performance limits, albeit with escalating costs. To address these challenges, attention has shifted toward lithium–sulfur batteries, which offer higher theoretical energy densities and cost-effectiveness. However, lithium–sulfur cells face challenges such as active-material loss, excessive electrolyte usage, and rapid degradation of lithium–metal anodes. To overcome these issues, research has focused on optimizing cell configurations and fabrication parameters while exploring novel electrolytes and electrode materials. This feature article delves into the intrinsic material challenges and extrinsic engineering issues in current lithium–sulfur research and explores the development of advanced lithium–sulfur cells with crucial progress on high-loading sulfur cathodes, lean-electrolyte cells, and solid-state electrolytes. Moreover, it outlines the fundamental principles, structures, performances, and developmental trajectories indicated in research articles published after 2020, highlighting future research directions aimed at resolving key challenges for the practical application of lithium–sulfur cells.
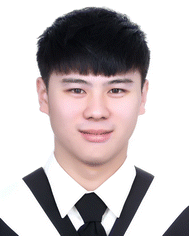
Cheng-Che Wu
| Cheng-Che Wu received his BS degree from the Department of Chemistry in 2021 and is currently a first-year PhD student in the Department of Materials Science and Engineering at the National Cheng Kung University under the supervision of Professor Sheng-Heng Chung. His PhD research focuses on the fabrication of battery materials, structural design of electrodes, and electrochemical evaluation for high-energy-density lithium–sulfur batteries. |
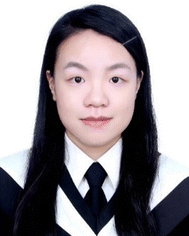
Tzu-Ching Chan
| Tzu-Ching Chan joined the group of Professor Sheng-Heng Chung in 2022. She received her master's degree in the Department of Materials Science and Engineering at the National Cheng Kung University in 2024, and now, she is a first year PhD student in the same institution. Her research topic focuses on the process of electrospinning, the fabrication of polymer electrolytes, the assembly of lithium–sulfur cells and electrochemical analyses of the batteries. |
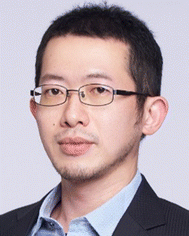
Sheng-Heng Chung
| Sheng-Heng Chung received his BS and MS degrees from the National Cheng Kung University and the National Tsing Hua University, respectively, and his PhD degree under the supervision of Professor Arumugam Manthiram at The University of Texas at Austin. In 2019, he started at the National Cheng Kung University as a Yushan Young Scholar and is currently an Associate Professor. His research interests include lithium–sulfur batteries, lithium-ion batteries, and electrochemical energy storage/conversion. |
1. Introduction
1.1. Lithium-ion batteries
Commercial lithium-ion cells, proposed in 1990, had stable insertion and extraction of lithium ions through (de)intercalation reactions between layered-oxide positive electrodes and graphite-based negative electrodes. This mechanism allows lithium-ion batteries to undergo reversible charging and discharging with high electrochemical utilization for outstanding energy densities of approximately 100–350 W h kg−1 and long-term stability over 1000 cycles during cycling and low self-discharge rates of less than 5% per month during resting.1–3 Due to their significantly higher energy density, longer cycle life, and more stable storage compared with other rechargeable batteries, lithium-ion batteries have emerged as the prevalent battery technology in the energy-storage market over the past 30 years. However, through continuous research and modification, the energy storage capacity of the active materials has been driven to approach a theoretical limit of 200–300 mA h g−1.3–5 Moreover, the escalating cost of the active materials for positive electrodes, the most expensive components of battery cells, have increased the cost of electrode and battery production. Given the limitations of battery performance and the increasing cost, the growth of the energy density of lithium-ion batteries has decreased from 7% to 2% per year. Thus, improving the performance and lowering the cost of lithium-ion batteries are crucial for achieving the demands of the expanding energy storage market.1–6
To advance energy storage technologies, next-generation batteries are being developed considering two key aspects: first, in terms of battery reaction mechanisms, electrochemical cells are being developed to exploit the full chemical energy of materials as electrical energy while maintaining high stability. Second, in terms of battery composition, solid-state electrolytes are being developed to fully leverage their excellent physicochemical and electrochemical stabilities to achieve long-term cycling stability and safety. These two main directions are expected to yield high energy density rechargeable batteries with 300–500 W h kg−1 and 700–800 W h L−1.4–8
1.2. Lithium–sulfur batteries
Lithium–sulfur cells, which have a similar cell configuration to that of lithium-ion cells, use sulfur as the electrochemical positive electrode (cathode) to achieve a high theoretical capacity of 1675 mA h g−1, outperforming existing solid-state electrodes. Sulfur is characterized by its high capacity, low cost, low toxicity, and abundance. Combined with a lithium-metal negative electrode (anode) possessing the lowest standard oxidation–reduction potential, electrochemical lithium–sulfur cells exhibit an outstanding theoretical energy density of 2600 W h kg−1 (ref. 7–9) and have the potential to attain high specific energy densities of 300–500 W h kg−1 and 700 W h L−1.10–13
The remarkable performance of lithium–sulfur cells is attributed to their unique conversion battery chemistry.8,14–17 During discharging, the sulfur (S8) in the cathode undergoes a chemical reaction with lithium ions in the electrolyte to form lithium sulfide (Li2S). This reaction that involves the delivery of two electrons by the low atomic weight sulfur allows for a ten-fold increase in capacity.14–16 Moreover, the conversion reactions of sulfur do not impose structural limitations akin to those of oxide structures in lithium-ion battery cathodes, enabling complete electrochemical utilization and reversible reactions during repeated charging and discharging.16,17
Although the fundamental composition of lithium–sulfur cells shares similarities with that of commercial lithium-ion cells, the conversion electrochemical reactions involved in lithium–sulfur cells, coupled with the physicochemical characteristics of their sulfur cathodes and unique redox reactions, distinguish them from conventional lithium-ion batteries (Fig. 1).14,18–20
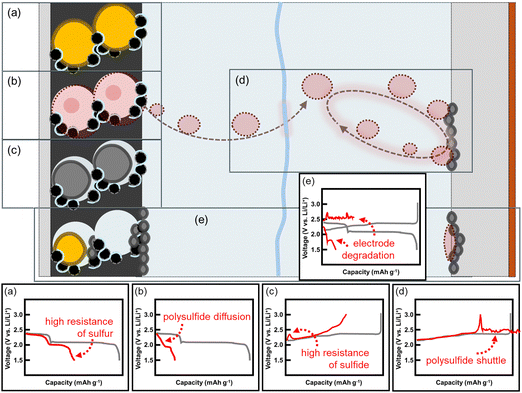 |
| Fig. 1 Challenges related to battery materials and cell electrochemistry of lithium–sulfur cells: (a) sulfur in the full-charge state, (b) polysulfides in intermediate charge/discharge states, (c) sulfide in the full-discharge state, (d) polysulfide diffusion, and (e) electrode instability. | |
The sulfur cathode, despite having the highest theoretical capacity among all solid-state active materials, also exhibits a high resistance of 10−30 S cm−1. This property results in low electrochemical utilization of the active material and low reversible capacity during cycling (Fig. 1(a)).17,21,22 When the solid-state sulfur in the cathode reacts with lithium ions in the electrolyte during discharging, it gradually forms liquid-state polysulfides (Li2S4–8).14,16,23 These liquid-state polysulfides, highly soluble in the electrolyte, can easily dissolve from the cathode. The dissolved polysulfides may irreversibly diffuse within the battery, further contaminating the lithium metal in the negative electrode and causing the deposition of lithium sulfide, which acts as an insulator. The generation, dissolution, diffusion, and electrode contamination by polysulfides result in an irreversible loss of the active material and electrode degradation, contributing to the rapid capacity loss and short cycle life in lithium–sulfur cells (Fig. 1(b)).20,24–26 During discharge, the liquid-state polysulfides retained in the cathode convert to solid-state lithium sulfide (Li2S2/Li2S), which exhibits a low conductivity of 10−14 S cm−1. This high internal resistance leads to severe polarization during charging, restricting rechargeability (Fig. 1(c)).8,20–22 In the charging reactions, solid-state lithium sulfide oxidizes into liquid-state polysulfides and solid-state sulfur. However, as the diffused polysulfides convert to higher-order polysulfides and sulfur during oxidation, they react with the lithium-metal electrode to form lower-order polysulfides and sulfides. This conflict between electrochemical and chemical reactions results in a low discharge/charge efficiency and even cell failure (Fig. 1(d)).24–26 Moreover, although the conversion battery reaction alleviates the constraints on the structure of the active materials to enhance their utilization and efficiency, they undergo reversible structural changes in the cathode. Given that the densities of sulfur and sulfide are 2.07 g cm−3 and 1.66 g cm−3, respectively, the sulfur in the cathode experiences a volumetric change approaching 80% and solid–liquid–solid conversion in each charge–discharge cycle. These volume and state changes soon deteriorate the electrode structure during repeated cycles, causing the rapid failure of electrode stability (Fig. 1(e)).10–12,27
Notable progress in lithium–sulfur cell technology was initiated in 2009 with the use of a mesoporous carbon matrix and a thermal-melt treatment method. This process facilitates the integration of low-viscosity molten sulfur into a conductive carbon matrix, enhancing the sulfur utilization. Throughout cycling, the generated polysulfides are accommodated within the porous carbon, mitigating the active-material loss and preserving the electron/ion conductivity.28–31 Subsequently, developments in carbon/sulfur composite cathodes, using advanced structural design and composition methods, led to enhanced lithium–sulfur battery performance.6–8,30–35
To facilitate use in composite cathodes, porous carbon was incorporated into the cell configurations after 2012.7–11,36–43 A porous current collector was developed to replace the conventional aluminum foil current collector, which improved the utilization and retention of the active material.38–40,44 An interlayer inserted between the cathode and the separator and a coated separator with a functional coating layer facing the cathode were designed after 2012 and 2015, respectively, to block the fast diffusion of the active material from the cathode, enhancing the stability and cycle life.36,37,45–47 Given the notable enhancements in discharge capacity approaching 90% utilization of sulfur and a cyclability approaching 1000–2000 cycles,48–51 lithium–sulfur cells have emerged as the forefront of next-generation battery research and development. This positioning is crucial for maintaining a competitive edge in the commercial lithium-ion battery market.7,52–56
1.3. Advanced lithium–sulfur batteries
Over nearly a decade of development, various additives and composite structures have been introduced in electrochemical lithium–sulfur cells. The functional materials used in these cells span a wide range, including polymers, ceramics, and metals.7–9,15–20 Nevertheless, many of these materials still heavily rely on integration with substantial amounts of carbon-based materials in the cell, with porous and conductive carbon being widely used.11,32,53–56 Notably, a large number of studies published between 2015 and 2020 have revealed that the conversion reactions within the battery and solid–liquid–solid state changes in sulfur are significantly affected by cell fabrication parameters.53–60 This influence extends beyond mere concerns about scaling up and the costs of mass production in the intersection of academic research and industrial applications.58–60 In fact, it penetrates the core aspects of electrochemical performance and battery chemistry.14,60 As a result, the intrinsic challenges of materials in electrochemical lithium–sulfur cells must be addressed in conjunction with the extrinsic challenges posed by the cell fabrication parameters.53–56,60–62
In terms of the electrochemical reactions of lithium–sulfur cells, solid-state sulfur and sulfide are highly insulating, leading to sluggish electrochemical utilization.14–17,60 To address the challenges posed by low electrochemical stability and efficiency attributable to the high resistance of insulating active materials and significant polarization in the reaction process, a considerable amount of conductive porous carbon and various functional materials are often integrated into the composite active materials with sulfur.7,11,57–59 These composite active materials typically contain 60–80 wt% sulfur content. Weighing between 70 and 80 wt%, these composites are then mixed with a significant amount of conductive carbon and binder to form a cathode slurry on a heavy aluminum foil current collector (∼5 mg cm−2). In the resulting electrode, the actual content of the active material participating in the electrochemical reaction falls below the critical threshold of the 60 wt% sulfur content, resulting in deteriorated specific gravimetric capacity and overestimated battery performance.57–62 The incorporation of a large amount of these inactive materials was initially aimed at utilizing the high specific surface area and large pore volume of porous materials and the surface adsorption capabilities of functional materials to impede the loss of liquid-state polysulfides.7,11,19–22 However, this kind of incorporation has faced growing criticism, as porous carbon and functional materials may adsorb and consume the electrolyte. In such scenarios, excessive electrolyte must be added during cell fabrication to ensure thorough wetting of the components.7,11,54–57 Furthermore, a surplus of electrolyte is required to compensate for the continuous consumption by adsorbent materials and electrodes during conversion reactions, leading to elevated electrolyte-to-sulfur ratios exceeding 20 μL mg−1.54–57 This surplus of electrolyte not only results in low energy density and an overestimation of polysulfide stability but also obscures polarization issues and complicates the calculation of reaction kinetics during the reduction and oxidation reactions of solid-state sulfur and sulfide.7,14,58–60 In terms of cathode preparation, most lithium–sulfur cells exhibit varied electrochemical and performance characteristics with low sulfur loading, typically ranging from 1 to 2 mg cm−2.7,54–56 However, cathodes with low sulfur loading cannot authentically reflect the insulation and polarization phenomena caused by solid-state sulfur and sulfide. Additionally, they fail to accurately deal with issues related to the loss of liquid-state polysulfides or demonstrate effective retention.7,14,54–56 Moreover, most lithium–sulfur cells utilize an excess of lithium metal electrodes with a high negative to positive electrode capacity ratio along with a high electrolyte volume. This approach, however, conceals the irreversible lithium consumption within the battery arising from the conversion battery reactions and solid–liquid–solid state transformations in the positive electrode.14,55,58
To address these challenges, the next generation of lithium–sulfur batteries is undergoing meticulous refinement to enhance both their performance and their application prospects. Current research efforts in advanced lithium–sulfur cells are concentrated on optimizing cell fabrication parameters to generate reliable electrochemical analysis data, while better understanding battery performance and its practical implications.10–12,14,26,52–60 The primary goal is to meet and surpass established standards for battery technology parameters by supplementing the amount of active material in the cathode, minimizing surplus electrolyte and anode material, and incorporating diverse battery process technology parameters within one cell. The ultimate aim is to demonstrate enhanced electrochemical performance and comprehensively assess the practicality of these advancements.7,14,52–60,63–65
Table S1 (ESI†) summarizes the recommended cell fabrication parameters and cell performance values outlined in recent review/perspective articles on lithium–sulfur batteries. In particular, the cathode should have a sulfur loading of 5 mg cm−2 and a sufficient sulfur content (60–80 wt% per electrode in the cell) while maintaining a low electrolyte-to-sulfur ratio of 4.0 μL mg−1. This configuration enables the development of a lithium–sulfur cell with an energy density approaching 400 W h kg−1. To achieve an energy density of 500 W h kg−1, the sulfur loading in the cathode should be over 6 mg cm−2 with 60–80 wt% sulfur per electrode, coupled with a low electrolyte-to-sulfur ratio of only 2.5 μL mg−1.63–65 In addition, the high-sulfur-loading cathode must be balanced with the lithium anode to achieve a negative to positive electrode ratio of less than 5.0 to 2.0. In such a cell, the electrochemical utilization is expected to remain as high as 1000 to 500 mA h g−1, accompanied by electrolyte-to-sulfur ratios of 5.0 to 2.5 μL mg−1. Overall, based on the values suggested in recent review articles and their averages, developing practical lithium–sulfur cells with high energy densities requires a sulfur loading exceeding 5 mg cm−2, a sulfur content of 60–80 wt% per electrode, electrolyte-to-sulfur ratios below 5.0 μL mg−1, negative to positive electrode ratios below 5.0, and electrolyte-to-capacity ratios below 5.0 μL mA h−1. Advanced lithium–sulfur cells meeting these criteria are expected to demonstrate cyclability and rate capability, indicative of a practical and reliable battery.9–12,52–65
Based on the suggested cell fabrication parameters mentioned above, Fig. 2 shows a comprehensive review of research articles on lithium–sulfur cells published between 2018 and 2024. We first investigate the critical cell fabrication parameters that dominate the overall electrochemistry of lithium–sulfur cells: sulfur loading, sulfur content, and the electrolyte-to-sulfur ratio. The amount of active material and its electrochemical utilization determine the specific capacity and the corresponding energy density. Ideally, the development of lithium–sulfur battery cathodes involves increasing both the sulfur loading and content. However, the insulating nature of sulfur and sulfides affects the utilization of high-loading sulfur cathodes. As shown in Fig. 2(a) and (b), most published research studies report sulfur loading and content lower than the required standards and face challenges in demonstrating cell performance and analysis using high-loading sulfur cathodes. Due to the conversion electrochemistry of sulfur, the formation, dissolution, and diffusion of polysulfides are influenced by the amount of electrolyte. Additionally, the amount of electrolyte affects ion transfer within the cell during cycling. Fig. 2(c) shows that the electrolyte-to-sulfur ratio is commonly high in research, implying a hidden risk in the discussions and proposed solutions addressing polysulfide issues and reaction kinetics related to the electrolyte amount in the cell.
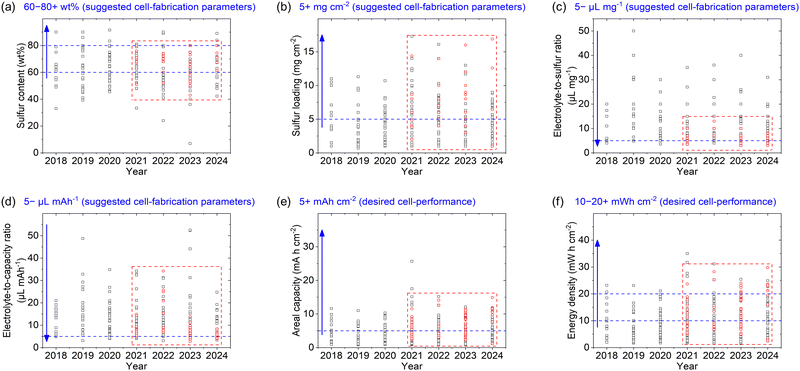 |
| Fig. 2 Cell fabrication parameters and cell performance comparisons: (a) sulfur contents, (b) sulfur loadings, (c) electrolyte-to-sulfur ratios, (d) electrolyte-to-capacity ratios, (e) areal capacities, and (f) energy densities. | |
Fig. 2(d)–(f) summarize cell performances, including the electrolyte-to-capacity ratio, areal capacity, and energy density. The commonly used high electrolyte-to-sulfur ratios result in the high electrolyte-to-capacity values, which is the main challenge in the progress of lithium–sulfur technology achieving a high energy density. The high electrolyte-to-sulfur and electrolyte-to-capacity ratios further imply that the current developments might either consume excessive electrolyte during trapping polysulfides or make limited improvements in the reaction catalysis. In addition, the use of low-loading sulfur cathodes in the cell inevitably leads to low areal capacity and energy density that need to be improved.
In contrast, Fig. 2 also illustrates increasing research efforts focused on enhancing cell fabrication parameters through appropriate materials and configuration designs, resulting in advancements in practical cell performance. Many of these studies successfully achieve at least one or two crucial parameters or performance values. The remaining challenge lies in achieving all necessary cell-fabrication parameters while maintaining high electrochemical performance under such critical conditions. Our group recently contributed to the design of several advanced lithium–sulfur batteries, including high-loading, lean-electrolytes, and solid-state-electrolyte configurations, highlighted in the red box in Fig. 2. These advanced lithium–sulfur batteries demonstrate how the design of the electrode substrate and the structure enhances the cell performance through customized configurations. To explore further developments in advanced lithium–sulfur batteries, this feature article brings together our key results and showcases the progressive cell designs and performances.7–12,52–65
2. Advanced lithium–sulfur batteries: high-loading lithium–sulfur cells
Sulfur cathodes offer a high capacity but are significantly more insulating than oxide electrodes. Thus, sulfur loading directly influences the reaction kinetics and active material retention of the cell. To promote commercialization and research, it is essential to increase the sulfur loading and content of the cells that dominate the performance metrics: areal capacity and energy density.63–65 In particular, low-loading sulfur cathodes lead to a low areal capacity and energy density, rendering commercialization challenging. However, high-loading sulfur cathodes face aggravated rapid polysulfide diffusion and insulative sulfide precipitation on the electrode surface, intensifying irreversible capacity decay within a shorter cycle life.52–60 To address these issues, recent studies on high-loading sulfur cathodes have focused on the use of porous current collectors and composite materials, as discussed in the following sections.
2.1. Porous current collectors
Porous current collectors with conductive networks and porous structures have been proposed to replace heavy aluminum-foil current collectors. Such current collectors accommodate a large amount of active material and host the dissolved polysulfides, thereby improving the electron transport and electrolyte distribution throughout the cathode. A three-dimensional (3D) conductive porous metal was initially proposed as the substrate material for current collectors in lithium–sulfur cells. Compared to traditional aluminum foils, which lead to severe self-discharge behavior, the cell with a porous current collector demonstrated better cycle stability, higher capacity retention, and lower self-discharge behavior. This indicates that porous current collectors are effective in improving the cycling performance of lithium–sulfur cells.66 Moreover, carbon nanofoams containing conductive networks can serve as effective current collectors to enhance the conductivity of the cathode. Graphene and molybdenum disulfide (MoS2) coatings have been applied over carbon nanofoam frameworks to improve the charge-transfer and polysulfide-trapping abilities, respectively. In particular, with a high sulfur loading of 4.8 mg cm−2 and a low electrolyte-to-sulfur ratio of 10 μL mg−1, cells utilizing carbon nanofoam, graphene-coated carbon nanofoam, and MoS2-coated carbon nanofoam have demonstrated high initial discharge capacities of 500 mA h g−1, 672 mA h g−1, and 633 mA h g−1, respectively. These cells exhibit a long cycle life over 100 cycles and a high capacity retention of 90% (Fig. 3(a)).67 Carbon nanofoam, when applied with an additional upper current collector, can serve as an interlayer in lithium–sulfur cells to improve their electrochemical utilization, efficiency, and stability. The porous and tortuous conducting carbon networks accelerate charge transfer and retard polysulfide diffusion. Thus, cells with a high sulfur loading of 8 mg cm−2 have been noted to exhibit a high electrochemical utilization of 65%, an areal capacity of 8.4 mA h cm−2, and an energy density of 18.2 mW h cm−2 with a long life of 200 cycles (Fig. 3(b)).68 Polyaniline is considered a promising material for the current collector in lithium–sulfur cells due to its high conductivity and strong affinity toward polysulfides. Chen et al. developed a covalent organic framework (COF)-like conjugated microporous polyaniline (CMPA) featuring an extended π-conjugated system and permanent 3D microporosity to alleviate the issues associated with unmodified polyaniline, i.e., an unstable frame structure, a low specific surface area, and few polar groups. The cell using the CMPA current collector incorporated a high-sulfur-loading cathode with 8.72 mg cm−2 sulfur to achieve a high areal capacity of 7.42 mA h cm−2, a high energy density of 20.3 W h g−1, and a long cycle life of over 500 cycles at 1C rate, with a low electrolyte-to-sulfur ratio of 6 μL mg−1 (Fig. 3(c)–(f)).69
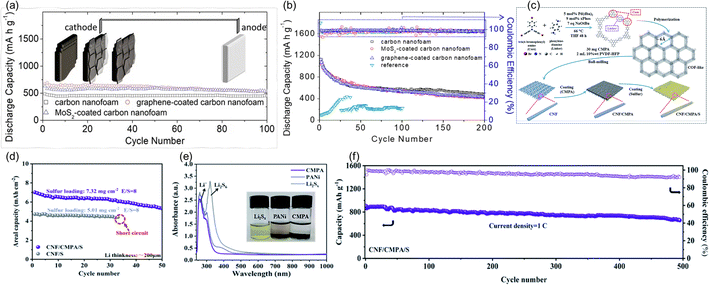 |
| Fig. 3 Porous current collectors. (a) Cyclability of carbon-nanofoam cathodes at C/10. Reproduced with permission.67 Copyright 2021, MDPI. (b) Cyclability of the cells with interlayers at C/10. Reproduced with permission.68 Copyright 2021, MDPI. (c) Schematic of the fabrication of a carbon nanofiber/conjugated microporous polyaniline/sulfur (CNF/CMPA/S) cathode. (d) Cyclability of CNF/CMPA/S and CNF/S cathodes at C/10. (e) Polysulfide adsorption based on polyaniline (PANi) and CMPA. (f) Cyclability of the CNF/CMPA/S cathode at 1C. Reproduced with permission.69 Copyright 2022, Royal Society of Chemistry. | |
In summary, this section introduces porous current collectors with conductive networks that are being developed to replace heavy aluminum foil collectors in lithium–sulfur cells. Various materials and structures are being adopted to enhance electron transport, electrolyte distribution, and polysulfide trapping. These advancements enable a high sulfur-loading capability along with high and stable electrochemical utilization of the large amount of sulfur within the porous current collector.
2.2. Composite materials
To address irreversible polysulfide diffusion, which leads to deteriorated cell performance in lithium–sulfur cells, and enhance the sulfur loading and sulfur content, one direct solution is to package the sulfur in porous conductive carbon substrates, forming a carbon–sulfur nanocomposite. In this manner, composite cathodes could contain a significant amount of insulating sulfur and capture the dissolved polysulfides in the liquid electrolyte. Commonly used conductive carbons (i.e., super P, black pearls, Ketjen black, and Vulcan black) have been incorporated in electrode preparation and cell-fabrication processes as carbon substrates to form carbon–sulfur nanocomposites with a high sulfur content of 80%. These carbon substrates comprehensively covered nonporous, microporous, micro/mesoporous, and macroporous carbons. Among them, the microporous carbon–sulfur cathode enabled a high sulfur loading of 4 mg cm−2 to achieve a high capacity approaching 1100 mA h g−1 along with a high capacity retention of 75% after 100 cycles, an areal capacity of 4.3 mA h cm−2, an energy density of 9.2 mW h cm−2, and reversibility of C/20–C/3 rates. This study recommended the selection of microporous carbon as the substrate for developing high-sulfur-loading carbon–sulfur composite cathodes (Fig. 4(a)).70 To improve the electrochemical utilization and stability of the active material, another method is to introduce an oxide–sulfur nanocomposite cathode, which features high electrocatalytic activity and forms strong covalent bonds with polysulfide molecules. A series of oxide-based polysulfide adsorbents, such as TiO2, ZrO2, SiO2, ZnO, and Al2O3, have been proposed to anchor polysulfides and promote electrocatalytic conversion. A cell with SiO2 has been noted to offer excellent polysulfide adsorption and electrocatalytic conversion in a high-loading sulfur cathode with 4 mg cm−2 of sulfur. This cell achieved a high capacity of 895 mA h g−1, a cycle life of 200 cycles, a rate performance from C/20 to C/2, an areal capacity of 3.7 mA h cm−2, and an energy density of 7.9 mW h cm−2. In addition, the undercoordinated sulfur atoms located at the edge sites of layered 2D transition metal sulfides are catalytically active, facilitating the anchoring–diffusion–conversion of polysulfides and inhibiting the shuttle effect in lithium–sulfur cells (Fig. 4(c)).71 A composite cathode consisting of conductive polymers could inhibit the diffusion of polysulfides and enhance the sulfur content and loading of lithium–sulfur cells. Anand et al. fabricated three different composite cathodes by mixing sulfur with polyaniline, poly(3,4-ethylenedioxythiophene) polystyrene sulfonate (PEDOT:PSS), and polypyrrole, respectively. Among them, the S-polypyrrole cathode achieved the highest discharge capacity of 1100 mA h g−1, while the S-polyaniline and S-PEDOT:PSS cathodes showed discharging capacities of 900 and 800 mA h g−1 at a high sulfur loading of 4 mg cm−2.72
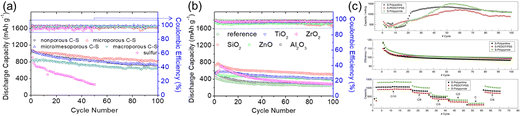 |
| Fig. 4 Composite materials. (a) Cyclability of carbon–sulfur nanocomposite cathodes at C/10. Reproduced with permission.70 Copyright 2022, MDPI. (b) Cyclability of oxide–sulfur composite cathodes at C/10. Reproduced with permission.71 Copyright 2022, American Chemical Society. (c) The cyclability, Coulombic efficiency, and rate performances of the cells with S-polyaniline, S-poly(3,4-ethylenedioxythiophene) polystyrene sulfonate (S-PEDOT:PSS), and S-polypyrrole, respectively.72 Copyright 2019, Elsevier. | |
In conclusion, to address polysulfide diffusion in lithium–sulfur cells, sulfur is packaged in porous conductive carbon substrates, forming carbon–sulfur nanocomposites. Composite cathodes designed with carbons, polymers, and oxides can utilize their unique material characteristics to inhibit polysulfide diffusion, thereby enhancing the lithium–sulfur cell performance. By utilizing the porous current collectors and the composite cathodes, the sulfur content and loading can be increased, advancing the development of lithium–sulfur cells closer to commercialization.
3. Advanced lithium–sulfur batteries: lean-electrolyte lithium–sulfur cells
The advanced lithium–sulfur concept introduced in 2021 aimed to use practical cell fabrication parameters to reflect actual electrochemical performance and progress. This approach has inspired numerous experimental and computational studies, highlighting key insights. Although the formation of liquid-state polysulfides at intermediate discharging and charging states is a major challenge in lithium–sulfur cell electrochemistry, most research papers attempt to mitigate or inhibit polysulfide formation using excessive amounts of electrolyte. To address this issue and investigate polysulfide diffusion, testing conditions should use low electrolyte-to-sulfur ratios of 5–10 μL mg−1. Under these conditions, lithium–sulfur cells operate in a semi-dry state, known as lean-electrolyte cells. These cells provide insights into realistic battery reactions and electrochemistry and have become a research hotspot for exploring practical lithium–sulfur battery chemistry.60–64 Although lean-electrolyte cells have advantages, the low electrolyte usage makes it challenging to collect easily analyzable data and good-looking data using traditional cell designs.73 To address the drawbacks, recent advancements in lean-electrolyte lithium–sulfur cells focus on designing cathode substrates and materials to reduce electrolyte consumption while addressing the challenges and potential solutions for maintaining high electrochemical performance under lean electrolyte conditions.
3.1. Cathode substrates
The integration of a high-loading sulfur cathode in a lean-electrolyte cell is crucial for realizing a low electrolyte-to-sulfur ratio. Recent studies have demonstrated that cathode substrates with low surface areas and high conductivity provide a conductive network that can activate and stabilize large amounts of sulfur while minimizing surplus electrolyte. Cathodes designed appropriately can withstand lean-electrolyte and high-sulfur-loading conditions and reduce the consumption of electrolyte during cycling, thus enhancing the cyclability of lean-electrolyte lithium–sulfur cells.60–69
Carbon substrates have been widely explored for lithium–sulfur battery cathodes. The structure and configuration of carbon substrates influence the cathode preparation and resulting electrochemical performance in lean-electrolyte cells. Studies on high-loading, lean-electrolyte cathodes with carbon nanotube/nanofiber composites have revealed the mechanisms underlying cell failure at low electrolyte-to-sulfur ratios, showing that electrolyte consumption degrades lithium-ion transport through the electrolyte. Cathode substrates that can mitigate the absorption and consumption of the electrolyte could extend the cycle stability of lean-electrolyte cells (Fig. 5(a)–(c)).60 Poor and sluggish cathode kinetics at reduced electrolyte-to-sulfur ratios were demonstrated by Chen et al.74 By introducing carbonized electrospun nanofibers with tunable nanoporosity, the adverse impact of the high nanoporosity of cathode substrates was revealed (Fig. 5(d)). With non-nanoporous carbonized electrospun nanofibers, a high-loading polysulfide cathode (i.e., 71 wt% and 14.4 mg cm−2 sulfur) exhibited a high reversible capacity of 802 mA h g−1 at a low electrolyte-to-sulfur ratio of 4 μL mg−1 (Fig. 5(e)).34 These cells also maintained low self-discharge rates over 90 days of storage (Fig. 5(f)).35 Yang et al. demonstrated a low nanoporous substrate incorporating nickel and carbon nanotubes. This framework exhibited a high capacity of 412 mA h g−1 per cathode with a high sulfur loading of 6.52 mg cm−2 (Fig. 5(g)).74 In addition to fibrous substrates, nanostructured carbon particles have been widely adopted in cathode design. Feng et al. demonstrated that nitrogen-doped carbon with low porosity offers high capacity under low electrolyte-to-sulfur ratios (Fig. 5(h) and (i)).75 Furthermore, through structural design, the performance of the lean-electrolyte cell could be further improved. Tu et al. used a chunky sulfur/graphene cathode (Fig. 5(j)) to enhance the utilization of the active material, achieving a high battery energy density of 520 W h kg−1 at electrolyte-to-sulfur ratios under 3 μL mg−1 (Fig. 5(k)).76
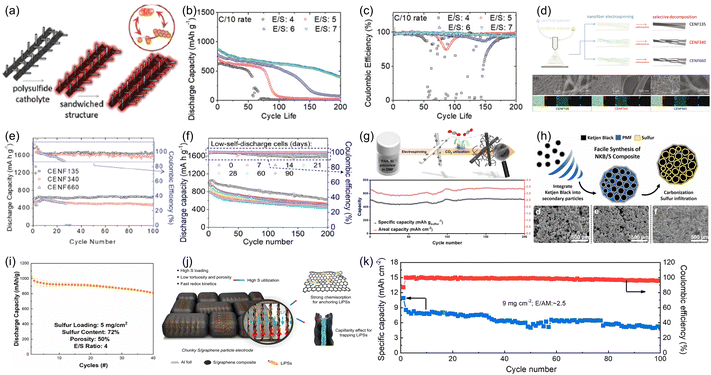 |
| Fig. 5 Cathode substrate. (a) Schematic and (b) and (c) cyclability of high-loading polysulfide cathodes with nanotube/nanofiber composites at C/10. Reproduced with permission.60 Copyright 2021, Royal Society of Chemistry. (d) Schematic and SEM images, (e) cyclability, and (f) self-discharge effect of polysulfide cathodes with carbonized electrospun nanofibers at C/10. Reproduced with permission.34 Copyright 2021, Elsevier. Reproduced with permission.35 Copyright 2023, Royal Society of Chemistry. (g) Schematic and cyclability of the low-nanoporosity substrate at C/5. Reproduced with permission.74 Copyright 2023, Elsevier. (h) Schematic and SEM images and (i) cyclability of cathodes with nitrogen-doped secondary carbon particles at C/16. Reproduced with permission.75 Copyright 2022, Elsevier. (j) Schematic and (k) cyclability of a high-loading and lean-electrolyte cathode and a chunky sulfur/graphene cathode at C/10. Reproduced with permission.76 Copyright 2023, American Chemical Society. | |
In addition to cathode substrate design, cathode preparation methods can be enhanced to exhibit the advantages of novel cathode substrates. Hot-pressed cathodes have been noted to encapsulate a large amount of sulfur within the substrate in a rapid fabrication time of 5 s (Fig. 6(a)). The high-loading hot-pressed cathode maintained stable cyclability under lean-electrolyte conditions (7–4 μL mg−1) (Fig. 6(b)).62 Moreover, a core–shell cathode design allowed a substantial amount of the polysulfide catholyte to be encapsulated within a conductive carbon shell with low nanoporosity (Fig. 6(c)). This unique cathode configuration attained a low electrolyte-to-sulfur ratio of 3 μL mg−1 with stable long cycle life (Fig. 6(d)).56 Notably, well-designed carbon substrates can help promote both cathode and anode applications. For example, a carbon structural material featuring carbon nanotubes entrapping conductive graphene was noted to facilitate the development of both high-sulfur-loading cathodes and stable lithium anodes (Fig. 6(e)). The lean-electrolyte lithium–sulfur full-cell exhibited a low negative to positive capacity ratio of 2.4, displaying stable cyclability with a high discharge capacity of 550 mA h g−1 and stability over 200 cycles (Fig. 6(f)).55 To improve the active material loading and energy density of lithium–sulfur batteries, Cheng et al. introduced a 3D free-standing aerogel composed of N-doped reduced graphene oxide modified by Co4N nanoparticles (Fig. 6(g)). This 3D cathode, pressed at 20 MPa, boasted a high sulfur loading of 24.0 mg cm−2 with a volumetric energy density of 2678.1 W h L−1 at an electrolyte-to-sulfur ratio of 3.3 μL mg−1 (Fig. 6(h)).77 Furthermore, Ma et al. introduced yolk–shell carbon flower@carbon nanospheres as sulfur hosts (Fig. 6(i)), which achieved a high sulfur loading of up to 12.5 mg cm−2 while lowering the electrolyte-to-sulfur rate to 5 μL mg−1 (Fig. 6(j)).78
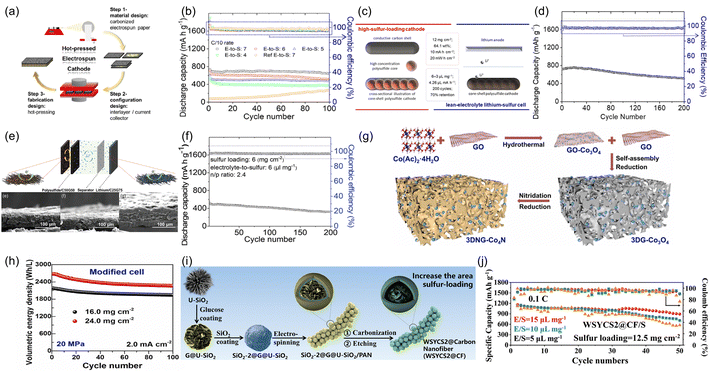 |
| Fig. 6 Cathode substrate. (a) Schematic and (b) cyclability of hot-pressed cathodes under lean-electrolyte conditions at C/10. Reproduced with permission.62 Copyright 2023, Elsevier. (c) Schematic and (d) cyclability of the core–shell polysulfide cathode with a low electrolyte-to-sulfur ratio of 3 μL mg−1 at C/10. Reproduced with permission.56 Copyright 2023, Royal Society of Chemistry. (e) Schematic and (f) cyclability of a full cell with a carbon-nanotube skeleton entrapping conductive graphene with a low negative to positive capacity ratio of 2.4 at C/10. Reproduced with permission.55 Copyright 2023, Wiley-VCH. (g) Schematic and (h) cyclability of a 3D free-standing cathode at C/13–C/20. Reproduced with permission.77 Copyright 2022, Elsevier. (i) Schematic and (j) cyclability of composite cathodes composed of a walnut-like yolk–shell carbon–sulfur host at C/10. Reproduced with permission.78 Copyright 2024, Wiley-VCH. | |
Overall, the development of lean-electrolyte lithium–sulfur cells benefits from the design of suitable conductive materials for the cathode substrate along with appropriate cathode configurations and fabrication processes. Using low nanoporous and conductive materials to construct stereoscopic cathode substrates allows a large amount of active material to be stored in the cathode and utilized, thereby offering exceptional battery energy density and stable long cycle life, while limiting the electrolyte consumption during cycling.
3.2. Adsorbers and catalysts
Functional adsorbent materials incorporated into cathode substrates offer surface chemical adsorption to capture polysulfides. In lean-electrolyte lithium–sulfur cells, this capability effectively reduces the loss of active materials, alleviates the necessity for excessive electrolyte, and reduces electrolyte consumption.79–84 Thus, low-surface area polysulfide absorption materials can help enhance the performance of lean-electrolyte lithium–sulfur cells.
Cement, a commonly used oxide mixture, has been introduced as a low-surface-area polysulfide adsorber (Fig. 7(a)). Using nonporous cement as a novel polysulfide adsorbent, a high-loading polysulfide and sulfur composite cathode with 6–9 mg cm−2 of sulfur achieved high areal capacities of 11.06 and 7.61 mA h cm−2 per cathode with stable cyclability under the lean-electrolyte condition (3 μL mg−1) (Fig. 7(b)).79,80 He et al. developed Nb-doped and oxygen-deficient TiO2 (Nb–TiO2−x) oxide materials to incorporate oxygen vacancies and Nb-doping to improve the electrical conductivity and facilitate the trapping and conversion of polysulfides (Fig. 7(c)). Wang et al. proposed a combination of transition metal oxide and transition metal phosphite, resulting in elevated polysulfide adsorption and catalysis capabilities (Fig. 7(e)). The introduction of both oxides into the lithium–sulfur cells enhanced the performance, achieving high sulfur loadings (>6 mg cm−2) and low electrolyte-to-sulfur ratios (<5 μL mg−1) (Fig. 7(d) and (f)).81,82 Furthermore, organic materials are potential polysulfide adsorbers for lean-electrolyte cells. Porous molecular crystals have been explored as polysulfide adsorbers in battery cathodes to improve the cycling performance. The high abundance of carbonyl groups and pores in cyclobenzoin ester resulted in the chemisorption of polysulfides while ensuring efficient electrolyte penetration, achieving a low electrolyte-to-sulfur ratio of 4 μL mg−1 and a low electrolyte-to-capacity ratio of 4.4 μL mA h−1 with a cycle life of 200 cycles (Fig. 7(g) and (h)).83 Zhang et al. introduced reactive polymer tubes as cathode materials. The carbonyl oxygen functional groups in the polymer tube exhibited a strong affinity to adsorb polysulfides while accelerating the conversion reaction during discharging. The resulting lean-electrolyte cell showed a high sulfur loading (9 mg cm−2) and a low electrolyte-to-sulfur ratio (6 μL mg−1) (Fig. 7(i)).84
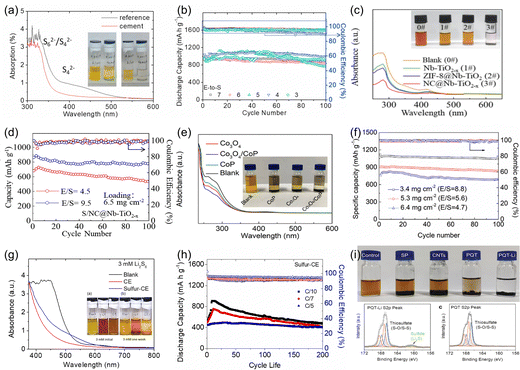 |
| Fig. 7 Adsorbers and catalysts. (a) Polysulfide adsorption measurements and (b) cyclability of polysulfide cathodes with cement at C/10. Reproduced with permission.79 Copyright 2023, Elsevier. (c) Polysulfide adsorption measurements and (d) cyclability of Nb-doped and oxygen-deficient TiO2 based sulfur cathodes. Reproduced with permission.81 Copyright 2022, Elsevier. (e) Polysulfide adsorption measurements and (f) cyclability of transition metal oxide/transition metal phosphate sulfur cathodes at C/10. Reproduced with permission.82 Copyright 2021, Elsevier. (g) Polysulfide adsorption measurements and (h) cyclability of porous molecular crystals in composite cathodes at C/10–C/5. Reproduced with permission.83 Copyright 2023, Elsevier. (i) Polysulfide adsorption and XPS spectrum of reactive-type polymer tubes. Reproduced with permission.84 Copyright 2023, Wiley-VCH. | |
Recently, high-entropy oxides and alloys have proven to be excellent adsorbers and catalysts for trapping and converting polysulfides. Specifically, (CrMnFeNiMg)3O4 high-entropy oxide was applied to high-loading sulfur cathodes (Fig. 8(a)).85–87 The strong polysulfide absorbability of (CrMnFeNiMg)3O4 with a high configuration entropy of 1.6R resulted in a long cycle life of 200 cycles and a high energy density of 14.12 mW h cm−2 for high-sulfur-loading cathodes (6–10 mg cm−2).86 This high-entropy oxide was further incorporated with a separator to hinder the polysulfide diffusion from the cathode to the lithium anode (Fig. 8(b)). With an improved polysulfide adsorption and conversion ability, the cells achieved a high capacity (990 mA h g−1), cycling rate (1C rate), and capacity retention rate (87%).87 Other high-entropy materials have also been introduced in lithium–sulfur cathodes. Raza et al. used the (Ni0.2Co0.2Cu0.2Mg0.2Zn0.2)O additive to facilitate chemical encapsulation and enhance the conversion reactions of polysulfides. The composite cathode (Ni0.2Co0.2Cu0.2Mg0.2Zn0.2)O with the support of additional Ketjen Black showed a high capacity (1044 mA h
g−1) at a high sulfur loading (4.4 mg
cm−2) (Fig. 8(c)).88 Wang et al. proposed the (Fe0.24Co0.26Ni0.10Cu0.15Mn0.25) high-entropy alloy with strong electrocatalytic activity toward polysulfides (Fig. 8(d)). With the accelerated conversion kinetics, the cell demonstrated a high sulfur loading (27.0
mg
cm−2) at a low electrolyte-to-sulfur ratio (3
μL
mg−1).89
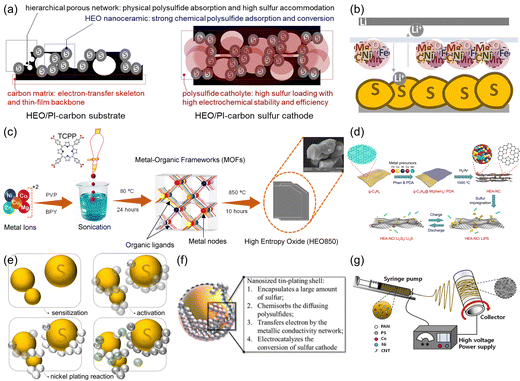 |
| Fig. 8 Adsorbers and catalysts. Schematic of the (a) (CrMnFeNiMg)3O4 composite sulfur cathode. Reproduced with permission.86 Copyright 2023, Elsevier. (b) The (CrMnFeNiMg)3O4 modified separator. Reproduced with permission.87 Copyright 2023, American Chemical Society. (c) Fabrication of the (Ni0.2Co0.2Cu0.2Mg0.2Zn0.2)O framework. Reproduced with permission.88 Copyright 2023, Wiley-VCH. (d) The (Fe0.24Co0.26Ni0.10Cu0.15Mn0.25) cathode. Reproduced with permission.89 Copyright 2022, Wiley-VCH. (e) Nickel-plated sulfur nanocomposites. Reproduced with permission.90 Copyright 2022, Wiley-VCH. (f) Tin-plated sulfur nanocomposites. Reproduced with permission.65 Copyright 2023, Royal Society of Chemistry. (g) Ni–Co metal alloy and NiCoO2-embedded electrospun cathode substrates. Reproduced with permission.91 Copyright 2024, Wiley-VCH. | |
In addition to carbon, polymer, and oxide materials, metals, although less commonly reported, also show strong affinity for polysulfide along with high metallic conductivity, crucial for designing high-performance lean-electrolyte cells with a high-loading sulfur cathode. Electroless metal plating was conducted to prepare a series of metal/sulfur nanocomposites.61,65,90 The nickel-plated sulfur energy-storage material was formed using tunable plating conditions and various sulfur contents (60–95 wt%) for active material synthesis (Fig. 8(e)).90 With the nickel nanoshell coated on the sulfur particle surface, high electrochemical utilization and fast polysulfide conversion kinetics were achieved. The nickel–sulfur nanocomposite simultaneously achieved a high sulfur loading (14 mg cm−2), a high sulfur content (74 wt%), and a low electrolyte-to sulfur ratio (7 μL mg−1) to attain excellent battery cycling and high energy density.61 A tin-plated sulfur nanocomposite was developed through chemical plating (Fig. 8(f)). By plating a nanoshell on the sulfur surface, large amounts of sulfur and polysulfides were encapsulated in the cathode region, providing excellent high active-material loadings and contents of 6–10 mg cm−2 and 65–85 wt% under lean-electrolyte conditions, respectively. Due to the strong polysulfide electrocatalytic activity and adsorption ability of the tin nanoshell, high energy densities were achieved (13–24 mW h cm−2) with a low electrolyte-to-capacity ratio (3.75 μL mA h−1).65 In addition to enhancing the performance by combining the metal with active materials, embedding the metal particles in the cathode conductive substrate is a viable strategy for improving battery performances. Park et al. introduced an electrospun substrate embedded with the Ni–Co metal alloy and NiCoO2. The metal nanoparticles improved the substrate conductivity, while anchoring and catalyzing polysulfides to improve the cycling performance (Fig. 8(g)).91
In summary, this section introduces different functional polysulfide-adsorbing materials. The strong electrochemical affinity of metal oxides, polymers, high-entropy materials, and metals with polysulfides enhances the active material retention. The catalysis capability and high conductivity further improve the reaction kinetics to enhance the electrochemical utilization and reversibility. Moreover, through the suitable design of the microstructure and morphology of the additive and resulting composite cathode, the developed lean-electrolyte lithium–sulfur cells demonstrate high and stable utilization of the active material, while reducing the electrolyte consumption.
4. Advanced lithium–sulfur batteries: solid-state-electrolyte lithium–sulfur cells
The use of solid-state electrolytes in lithium–sulfur cells can mitigate active material loss and enhance energy density through improved configurations and electrochemistry.9–11 However, the low electrochemical stability and interface compatibility of the anode result in lithium deterioration, and high interface impedance leads to the separation of the conductive agent and active material during cycling. Thus, cells with solid electrolytes typically exhibit a short cycle life and rapid capacity decay. According to pioneering studies,9–11,92–107 an ideal solid electrolyte for lithium–sulfur cells should have several characteristics: preventing electrode contact; incorporating channels for stable lithium-ion transfer; exhibiting strong insulation capabilities, high ionic conductivity, low interface impedance, and high electrochemical stability; and inhibiting polysulfide diffusion. The main types of solid-state electrolytes are discussed in the following sections.
4.1. Polymer-based solid-state electrolytes
Polymer solid-state electrolytes are known for their low weight, high flexibility, high chemical stability, and low cost. Among polymer electrolytes, poly(ethylene oxide) (PEO) is widely used due to its ability to conduct lithium ions through the alkoxy groups on its main chain and segmental motion of the chains. A composite polymer electrolyte was prepared by combining PEO/lithium bis(trifluoromethanesulfonyl)imide (LiTFSI) with a polypropylene membrane. This electrolyte inhibited rapid polysulfide diffusion on the cathode and stabilized the lithium-ion transfer, resulting in a high discharge capacity of 1212 mA h g−1 and a cycle life of 200 cycles with a discharge/charge efficiency of >99% (Fig. 9(a) and (b)).92 Polyacrylonitrile (PAN) and poly(methyl methacrylate) (PMMA) can also be utilized as gel electrolyte membranes, prepared through coating or electrospinning technologies. Considering these aspects, a PMMA/LiTFSI gel polymer electrolyte (GPE) was cast on a polypropylene membrane. The carbonyl groups on the PMMA matrix formed strong bonds with lithium sulfides, thereby inhibiting polysulfide diffusion in lithium–sulfur cells. This PMMA-based GPE achieved an ionic conductivity of 2.44 × 10−2 S cm−1, higher than that (1.66 × 10−4 S cm−1) of its precursor without liquid electrolyte. The sulfur loading of the cells with this GPE was 4–10 mg cm−2, resulting in a high areal capacity of 7.1 mA h cm−2 and an energy density of 15 mW h cm−2 (Fig. 9(c)).93 Non-woven and non-nanoporous PAN membranes were prepared using electrospinning technology. This PAN membrane, consisting of continuously interconnected lithium-ion transfer networks, exhibited a high mechanical strength and could tolerate lithium stripping and plating from the electrode surfaces over 1000 h at a current density of 1 mA cm−1 in a symmetric cell. In a lithium–sulfur cell, the PAN membranes allowed the cathode to have high sulfur loading and sulfur content of 16 mg cm−2 and 80 wt%, respectively. The high polysulfide retention and stable lithium-ion transportation resulted in a long cycle life of 200 cycles, high areal capacities of 7.0–8.6 mA h cm−2, and high energy densities of 14.7–18.1 mW h cm−2 (Fig. 9(d) and (e)).94 Yang et al. synthesized an asymmetric bi-functional GPE (IPN-GPE) by in situ cationic polymerization of 1,3-dioxolane (DOL) (PDXL) and cross-linking of cyanoethyl polyvinyl alcohol (PVA-CN). In this bi-functional GPE, the carbonyl groups attached to the cathode side could anchor the polysulfides and PDXL attached to the anode side could promote the uniform Li deposition. Thus, the cell with the IPN-GPE retain a high capacity of 807 mA h g−1 after 500 cycles at C/2 and a high Coulombic efficiency of 99.6% (Fig. 9(f)).95 Li et al. synthesized a polyether-based GPE by ring-opening polymerization of DOL and thioacetamide (TAA) was added in the cathode to form a liquid state electrolyte interface between the GPE and the cathode. This liquid electrolyte interface which is sulfide-soluble could reduce the accumulation of non-conductive solid sulfide and improve the conversion kinetics of polysulfides. The cell with the polyether-based GPE and the cathode additive of TAA demonstrated a high discharge capacity of 1047 mA h g−1, a long cycle life over 200 cycles, and a great rate performance from C/10 to 4C (Fig. 9(g) and (h)).96
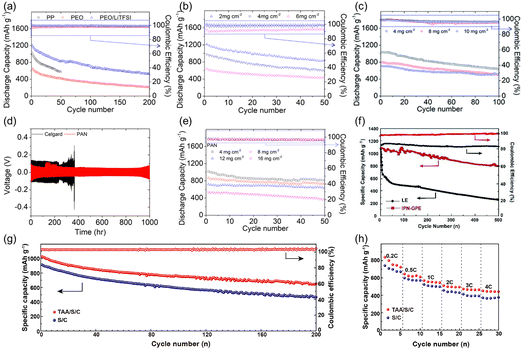 |
| Fig. 9 Polymer-based solid-state electrolytes. (a) Cyclability of cells with polypropylene (PP), poly(ethylene oxide) (PEO)-coated PP, and PEO/lithium bis(trifluoromethanesulfonyl)imide (LiTFSI)-coated PP membranes at C/10. (b) Cyclability of high-sulfur-loading cells with PEO/LiTFSI-coated PP membranes at C/10. Reproduced with permission.92 Copyright 2021, MDPI. (c) Cyclability of high-sulfur-loading cells with the poly(methyl methacrylate) (PMMA)-based GPE at C/10. Reproduced with permission.93 Copyright 2022, Royal Society of Chemistry. (d) Cyclability of the lithium//lithium symmetrical cells and (e) the high-sulfur-loading cells with electrospun polyacrylonitrile (PAN) nanofiber films at C/10. Reproduced with permission.94 Copyright 2023, MDPI. (f) The cycling performances of the cells with the liquid electrolyte and IPN-GPE.95 Copyright 2021, Royal Society of Chemistry. (g) Cycling performance at C/10 and (h) rate performance of the cells with and without the TAA additive.96 Copyright 2023, American Chemical Society. | |
4.2. Oxide-based solid-state electrolytes
Oxide-based electrolytes are characterized by stable crystal structures and lithium-ion transportation channels consisting of structural ceramics such as LiSICON, NASICON, perovskites, and garnets.97 Li1.5Al0.5Ge1.5(PO4)3 (LAGP), a NASICON type oxide electrolyte, features high chemical stability and a high ionic conductivity of 10−4 S cm−1 at room temperature. In addition, LAGP also acts as a physical barrier to prevent polysulfide diffusion and lithium dendrite growth in lithium–sulfur cells with its high relative density and high Young's modulus. Sun et al. optimized LAGP by adjusting its crystallinity through controlled sintering temperatures, resulting in a dense microstructure and effective inhibition of polysulfide diffusion (Fig. 10(a)). Their results showed the highest crystallinity of 99.9% and the best cell performance at a sintering temperature of 900 °C (Fig. 10(b)).98 The perovskite Li3xLa(2/3)−x□(1/3)−2xTiO3 (LLTO) featuring a high ionic conductivity of up to 10−3 S cm−1 was introduced as a replacement for liquid electrolytes in lithium–sulfur cells, in which a polysulfide cathode was applied to replace the solid sulfur cathode to achieve a high electrochemical performance and form a solid/liquid interface for rapid charge transfer. The LLTO solid electrolyte exhibited a high ionic conductivity of 3.9 × 10−4–1.2 × 10−3 S cm−1, the smooth interface between the surfaces of the solid electrolyte and the cathode ensured fast charge transfer, and the cell with the LLTO electrolyte achieved a high initial discharge capacity of 1429 mA h g−1 and promising rate performance from C/50 to C/3. Moreover, this LLTO solid-state electrolyte could inhibit rapid polysulfide diffusion and prevent the loss of active materials in the cathode, as indicated by an interface analysis (Fig. 10(c) and (d)).99 Garnets, Li7La3Zr2O12 and Li6.4La3Zr1.4Ta0.6O12, also exhibit high ionic conductivity ranging from 10−4 to 10−3 S cm−1. Shi et al. achieved a high initial capacity of 1308 mA h g−1 by using Li7La3Zr2O12 (LLZO) as the solid-state electrolyte in lithium–sulfur cells with a high sulfur loading of 4 mg cm−1. This LLZO electrolyte was doped with Ta and then coated with a thin layer of ZnO on the side facing the anode through atomic layer deposition. Poly(ethylene oxide) (PEO) was introduced between the LLZO electrolyte and the sulfur cathode to act as the buffer interlayer. The cell exhibited a high capacity of 1308 mA h g−1, an energy density of 257 mW h kg−1, and a rate performance of C/20–C/5 (Fig. 10(e)–(g)).100
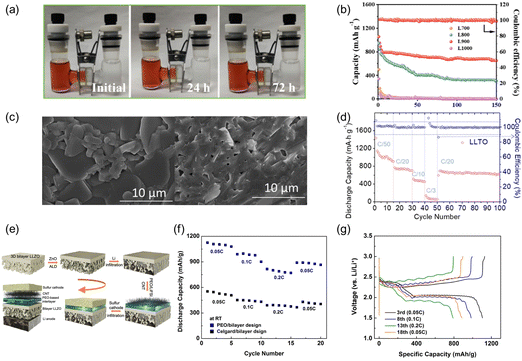 |
| Fig. 10 Oxide-based solid-state electrolytes. (a) Polysulfide permeability tests and (b) cyclability of the cells with the Li1.5Al0.5Ge1.5(PO4)3 (LAGP) solid electrolyte. Reproduced with permission.98 Copyright 2023, Elsevier. (c) SEM images of the LLTO solid–electrolyte surface and cross-sectional morphology. (d) Cyclability of a cell with Li3xLa(2/3)−x□(1/3)–2xTiO3 (LLTO) solid-state electrolyte. Reproduced with permission.99 Copyright 2024, Royal Society of Chemistry. (e) Schematic of the fabrication of a 3D asymmetric bilayer garnet-hybridized Li–S cell. (f) Rate performance and (g) voltage profile of PEO/bilayer and Celgard/bilayer cells. Reproduced with permission.100 Copyright 2023, American Chemical Society. | |
4.3. Sulfide-based solid-state electrolytes
Sulfide electrolytes are characterized by a crystalized Li10GeP2S12 structure and amorphous Li2S–SiS2 or Li2S–P2S5. These electrolytes can be integrated with a polysulfide cathode to lower the interface impedance of solid electrolytes.101 A Li2S-based catholyte/solid electrolyte composite was synthesized using P2S5 at low temperatures (∼50 °C). A typical Li2S cathode requires a high activation voltage of 4.0 V, leading to electrolyte and electrode degradation. However, the addition of P2S5 in the catholyte reduced the activation voltage to 3.0 V preventing electrolyte degradation and enhancing the electrochemical efficiency. With a high active material loading of 3.75 mg cm−2, the cell exhibited an initial discharge capacity of 700 mA h g−1, a high energy density of 5.6 mW h cm−2, and a long cycle life of 200 cycles (Fig. 11(a) and (b)).102 Additionally, a Li3PS4 sulfide solid-state electrolyte was coupled with a polysulfide cathode to optimize the performance of solid lithium–sulfur cells. The formation of a lithium phosphide and lithium phosphate protection layer, which is ionically conductive on the cathode side of this sulfide electrolyte, and the utilization of a polysulfide cathode lowered the interface impedance, improved charge transfer, and promoted lithium-ion diffusion. Therefore, the cell using the Li3PS4 sulfide electrolyte achieved a high sulfur loading of 5 mg cm−2, an initial discharge capacity of 1026 mA h g−1, an areal capacity of 5.1 mA h cm−1, and an energy density of 11.3 mW h cm−2 (Fig. 11(c)).103 In order to lower the high interfacial impedance between the sulfide electrolyte and the lithium sulfide cathode, Zhou et al. fabricated a modified sulfide electrolyte, Li7P2.9Ce0.2S10.9Cl0.3, which was formed by the dual-doping process. The Li7P2.9Ce0.2S10.9Cl0.3 electrolyte can achieve a high ionic conductivity of 3.2 mS cm−1. Therefore, the all-solid-state lithium–sulfur cell with the Li7P2.9Ce0.2S10.9Cl0.3 electrolyte showed a high capacity of 617 mA h g−1 and a long cycle life over 100 cycles. In addition, the cell demonstrated a high capacity retention of 89% after 100 cycles (Fig. 11(d)).104 Liu et al. utilized Li10GeP2S12, polyethylene oxide, (3-chloropropyl)trimethoxysilane, and nylon mesh to fabricate a sulfide-based electrolyte with a thickness reduced to 60 μm. The in situ polymerized fluorine-rich gel-state protective layer formed between this sulfide-based electrolyte and the anode surface improved the compatibility of the sulfide electrolyte with the lithium metal anode. Thus, the lithium//lithium symmetrical cell showed a long lifespan over 400 hours, while achieving a low overpotential. Moreover, the lithium–sulfur cell with this sulfide-based electrolyte showed a high initial discharge capacity of 1006.8 mA h g−1 and a high rate performance of C/10–1C (Fig. 11(e) and (f)).105
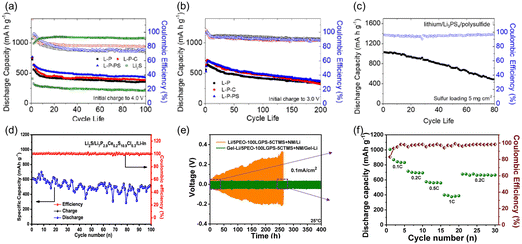 |
| Fig. 11 Sulfide-based solid-state electrolyte. Cyclability of cells with the sulfide solid-state electrolyte/Li2S cathode at activation voltages of (a) 4.0 V and (b) 3.0 V. Reproduced with permission.102 Copyright 2021, American Chemical Society. (c) Cyclability of a lithium/Li3PS4/polysulfide cell with a high sulfur loading of 5 mg cm−2 at a rate of C/20. Reproduced with permission.103 Copyright 2023, Royal Society of Chemistry. (d) Cyclability of the Li2S/Li7P2.9Ce0.2S10.9Cl0.3/Li–In battery at a current density of 0.06 mA cm−2.104 Copyright 2021, American Chemical Society. (e) Cyclability of the symmetric cells with sulfide-based electrolyte at a current density of 0.1 mA cm−2. (f) Rate performance of the MWCNT@S/5PEO-100LGPS-5CTMS + NM/gel-Li cell operated at 25 °C.105 Copyright 2022, Elsevier. | |
As a result, solid-state electrolytes that support stable lithium-ion transfer and inhibit polysulfide diffusion enhance the energy density and cycle stability in lithium–sulfur cells. Polymer electrolytes improve ion transfer and polysulfide retention, achieving high capacities and long cycle life. Oxide electrolytes provide a high ionic conductivity but face interface instability challenges. Sulfide electrolytes lower interface impedance and enhance electrochemical efficiency. Utilizing composite materials featuring the multi-functional properties of solid electrolytes in lithium–sulfur cells is one of the current methods to resolve the common problems of traditional electrolytes. Introducing inorganic fillers in polymer substrates to fabricate organic–inorganic composite solid electrolytes can provide more effective lithium-ion transportation. Moreover, inorganic fillers can also improve the mechanical strength and dimensional stability of the solid electrolyte. By combining the advantages of various materials, solid electrolytes could demonstrate promising capacities and cycle performance in lithium–sulfur cells.106
5. Prospective in advanced lithium–sulfur batteries: development and challenges
Enhancing battery chemistry and refining practical cell fabrication parameters are driving the development of advanced lithium–sulfur cells with high-loading sulfur cathodes. Whether as lean-electrolyte54–62,65 or solid-state-electrolyte cells,92–107 these advancements aim to boost the efficiency and viability of energy storage systems.7–12,52–65 Identifying challenges and implementing strategic solutions are crucial for achieving a targeted energy density of 400–500 W h kg−1.7,63,64 Efforts focus on enhancing cyclability, rate capability, and maintaining an 80% capacity retention.52–65 Incorporating lean-electrolyte and solid-state-electrolyte cells in semi-dry battery configurations is transformative, crucially combining stability and safety with high energy density in lithium–sulfur and solid-state battery systems.92–94,99–107
Exploring lithium–sulfur battery chemistry under optimal cell-fabrication conditions requires a thorough examination of key processes and potential failure mechanisms. Several critical challenges need addressing: (1) the high resistance of the solid-state active materials,17–22 (2) the low ionic conductivity of polysulfide catholytes,16,23–26 (3) impedance at the electrolyte/electrode interface,20–22,24–26 and (4) the development of thin lithium anodes.7,14,55,58,63 Progressing the understanding and efficacy of lithium–sulfur batteries under optimal fabrication settings necessitates exploring these factors.52–65,107 Future advancements in advanced lithium–sulfur cells are expected through focused efforts in these four key directions.
5.1. High resistance of solid-state active materials
Carbon/sulfur composites can help achieve reversible high capacity in lithium–sulfur cells.32,46,108–110 To develop a high-loading sulfur cathode with a sufficient sulfur content (i.e., 5+ mg cm−2 and 60–80 wt%), the carbon substrate can provide additional functions beyond the existing requirements.32,58–62 Although conductive carbon facilitates high electron transfer in the cathode, it encounters limitations in hosting a large amount of active material. The high porosity of the carbon substrate contributes to active material retention; however, increased porosity necessitates higher electrolyte-to-sulfur ratios to maintain the same electrochemical stability. The utilization of a large amount of conductive and porous carbon introduces challenges, requiring additional binders that lead to a reduced sulfur content.62–65 Thus, conductive carbon can be modified to achieve a porous network configuration without nanopores to host a large amount of active materials. Incorporating a polar material with chemical adsorption capability into the porous network made by non-nanoporous substrates is crucial for trapping polysulfides. Moreover, the composite carbon can enhance the mechanical strength of the substrate, tolerating the volume changes and state conversion of sulfur.
5.2. Low ionic conductivity of polysulfide catholytes
The lean-electrolyte condition, with an electrolyte-to-sulfur ratio of less than 5.0 μL mg−1, is crucial for enhancing the energy density of lithium–sulfur batteries.34,54–56 However, the electrolyte is continuously consumed by the electrodes during cycling, aggravating the sluggish polysulfide conversion kinetics. Moreover, the depletion of electrolyte can lead to complete failure of the battery. In lean-electrolyte cells with low-nanoporosity substrates, the introduction of polar surfaces and materials, such as heteroatom-doped carbon or metal compounds, enhances interactions with polysulfides,15,34,109 improving the rate and cycling performance.22,63,71,90,111–113 These kinetic promoters fall into two categories: heterogeneous electrocatalysts and homogeneous redox mediators. Both have been investigated for their mechanisms and performance in designing a carbon matrix with polarity and conductivity.
5.3. Impedance at the electrolyte/electrode interface
The semi-dry battery configuration has implications for the future development of solid-state lithium–sulfur cells with a high energy density and safety. However, these configurations also present challenges related to high interface impedance.54,99–107 In lean-electrolyte cells, the limited amount of electrolyte leads to the deposition of solid-state active material on the electrode, increasing interface impedance and compromising reversibility.59–62 Conversely, solid-state electrolytes can form a high-resistance interface with a solid-state electrode, hindering lithium-ion transfer.107 To address these challenges, an in situ interface resulting from sulfur chemistry may transform an inevitable problem into a potential solution. The use of a polysulfide catholyte in a high-loading sulfur cathode establishes a compatible liquid/liquid interface in lean-electrolyte cells and a smooth liquid/solid interface in solid-state-electrolyte cells. Additionally, polysulfides exhibit strong reaction activity, sustaining the conversion reaction of the sulfur cathode.99,102,103 These factors collectively ameliorate interface impedance in advanced lithium–sulfur cells.
5.4. Development of thin lithium anodes
Numerous strategies have been documented to protect lithium metal anodes. Although some studies have shed light on dendrite suppression mechanisms, their consideration of current density and areal capacity often lags behind advancements in cathode design.55,107,114,115 Thus, to evaluate the feasibility of a battery featuring a high-loading sulfur cathode with a specific areal capacity and operational current density, it is crucial to identify an anode protection method that aligns with these parameters. Moreover, many studies employ thick lithium anodes with excess capacity in designing lithium–sulfur cells. However, much of this excess lithium remains inactive in the electrochemical process, thereby reducing the battery's energy density. Thus, achieving a high energy density in advanced lithium–sulfur cells requires the use of a thin lithium anode.7,55–59 Accordingly, anode-protection strategies must therefore complement cathodes capable of high areal capacity at high current densities, ideally with a low negative-to-positive electrode capacity ratio below 5.0. In future progress, this alignment ensures seamless integration and synergistic operation of both electrode components, thereby optimizing the performance of advanced lithium–sulfur cells.
6. Conclusions
This feature article consolidates our key findings and emphasizes critical parameters essential for advancing lithium–sulfur cells with high-loading sulfur cathodes and innovative lean-electrolyte and solid-state-electrolyte configurations. This study highlights significant achievements in cell fabrication parameters and cell performance values, redefining the key scientific challenges encountered in practical advanced lithium–sulfur cells, extending beyond the previous considerations confined to ideal conditions. Forward-looking strategies are proposed as avenues for future research to overcome these challenges. Utilizing a composite carbon with a porous network, distinct from nanoporous conductive carbon, and modifying polar metallic surfaces play pivotal roles in advancing high-loading sulfur cathodes in lean-electrolyte and solid-state-electrolyte cells. Moreover, integrating polysulfide catholytes into well-designed cathode substrates is identified as a promising approach that is suitable for the unique battery chemistry of lithium–sulfur cells. This adaptation promises reduced internal impedance, ensuring high energy density and enhanced safety for solid-state batteries.
Author contributions
The manuscript was written through contributions of all authors. All authors have given approval to the final version of the manuscript.
Data availability
No primary research results, software or code have been included and no new data were generated or analyzed as part of this review.
Conflicts of interest
There are no conflicts to declare.
Acknowledgements
This work is supported by the National Science and Technology Council in Taiwan under grant 112-2636-E-006-006, 112-2923-E-006-004, and 113-2628-E-006-017-MY4, and by MA-tek under 2023-T-002 and in part by the Higher Education Sprout Project, Ministry of Education to the Headquarters of University Advancement at National Cheng Kung University.
Notes and references
- A. Manthiram, ACS Cent. Sci., 2017, 3, 1063–1069 CrossRef CAS PubMed.
- J. B. Goodenough and K. S. Park, J. Am. Chem. Soc., 2013, 135, 1167–1176 CrossRef CAS PubMed.
- A. Manthiram and J. B. Goodenough, Nat. Energy, 2021, 6, 323 CrossRef CAS.
- H. Ge, J. Qin, B. Zhang, L. Jiang, Y. Tang, B. Lu, S. Tian and J. Zhou, Nanoscale Horiz., 2024 10.1039/D4NH00243A.
- Z. Qin, T. Zhang, X. Gao, W. Luo, J. Han, B. Lu, J. Zhou and G. Chen, Adv. Mater., 2024, 36, 2307091 CrossRef CAS PubMed.
- A. Manthiram, Nat. Commun., 2020, 11, 1550 CrossRef CAS PubMed.
- H. Raza, S. Bai, J. Cheng, S. Majumder, H. Zhu, Q. Liu, G. Zheng, X. Li and G. Chen, Electrochem. Energy Rev., 2023, 6, 29 CrossRef CAS.
- Q. Wang, T. O’Carroll, F. Shi, Y. Huang, G. Chen, X. Yang, A. Nevar, N. Dudko, N. Tarasenko, J. Xie, L. Shi, G. Wu and D. Zhang, Electrochem. Energy Rev., 2024, 7, 15 CrossRef CAS.
- A. Manthiram, S.-H. Chung and C. Zu, Adv. Mater., 2015, 27, 1980–2006 CrossRef CAS PubMed.
- S.-H. Chung and A. Manthiram, Adv. Mater., 2019, 31, 1901125 CrossRef PubMed.
- S.-H. Chung, C.-H. Chang and A. Manthiram, Adv. Funct. Mater., 2018, 28, 1801188 CrossRef.
- S.-H. Chung and A. Manthiram, Joule, 2018, 2, 710–724 CrossRef CAS.
- A. Bhargav and A. Manthiram, Next Energy, 2023, 1, 100012 CrossRef.
- M. Wild, L. O’Neill, T. Zhang, R. Purkayastha, G. Minton, M. Marinescu and G. J. Offer, Energy Environ. Sci., 2015, 8, 3477–3494 RSC.
- L. Zhou, D. L. Danilov, F. Qiao, J. Wang, H. Li, R.-A. Eichel and P. J. L. Notten, Adv. Energy Mater., 2022, 12, 2202094 CrossRef CAS.
- T. Li, X. Bai, U. Gulzar, Y.-J. Bai, C. Capiglia, W. Deng, X. Zhou, Z. Liu, Z. Feng and R. P. Zaccaria, Adv. Funct. Mater., 2019, 29, 1901730 CrossRef.
- S. Lang, X. Feng, J. Seok, Y. Yang, M. R. Krumov, A. M. Villarino, M. A. Lower, S.-H. Yu and H. D. Abruña, Curr. Opin. Electrochem., 2021, 25, 100652 CrossRef CAS.
- R. Borah, F. R. Hughson, J. Johnston and T. Nann, Mater. Today Adv., 2020, 6, 100046 CrossRef.
- R. Deng, M. Wang, H. Yu, S. Luo, J. Li, F. Chu, B. Liu and F. Wu, Energy Environ. Mater., 2022, 5, 777–799 CrossRef CAS.
- J. Wang, H. Wang, S. Jia, Q. Zhao, Q. Zheng, Y. Ma, T. Ma and X. Li, J. Energy Storage, 2023, 72, 108372 CrossRef.
- G. Cao, R. Duan and X. Li, EnergyChem, 2023, 5, 100096 CrossRef CAS.
- M. Zhao, H.-J. Peng, B.-Q. Li and J.-Q. Huang, Acc. Chem. Res., 2024, 57, 545–557 CAS.
- G. D. Donato, T. Ates, H. Adenusi, A. Varzi, M. A. Navarra and S. Passerini, Batteries Supercaps, 2022, 5, e202200097 CrossRef.
- T. Maihom, J. Sittiwong, M. Probst and J. Limtrakul, Phys. Chem. Chem. Phys., 2022, 24, 8604–8623 RSC.
- J. Li, Z. Niu, C. Guo, M. Li and W. Bao, J. Energy Chem., 2021, 54, 434–451 CrossRef CAS.
- Y. V. Mikhaylik and J. R. Akridge, J. Electrochem. Soc., 2004, 151, A1969 CrossRef CAS.
- N. Nakamura, S. Ahn, T. Momma and T. Osaka, J. Power Sources, 2023, 558, 232566 CrossRef CAS.
- X. Ji, K. T. Lee and L. F. Nazar, Nat. Mater., 2009, 8, 500–506 CrossRef CAS PubMed.
- X. Ji, S. Evers, R. Black and L. F. Nazar, Nat. Commun., 2011, 2, 325 CrossRef PubMed.
- X. Ji and L. F. Nazar, J. Mater. Chem., 2010, 20, 9821–9826 RSC.
- S. Evers and L. F. Nazar, Acc. Chem. Res., 2013, 46, 1135–1143 CrossRef CAS PubMed.
- L. Zhang, Y. Wang, Z. Niu and J. Chen, Carbon, 2019, 141, 400–416 CrossRef CAS.
- A. Eftekhari and D.-W. Kim, J. Mater. Chem. A, 2017, 5, 17734–17776 RSC.
- Y.-C. Ho and S.-H. Chung, Chem. Eng. J., 2021, 422, 130363 CrossRef CAS.
- C.-C. Wu, Y.-C. Ho and S.-H. Chung, J. Mater. Chem. A, 2023, 11, 24651–24660 RSC.
- Y.-S. Su and A. Manthiram, Nat. Commun., 2012, 3, 1166 CrossRef PubMed.
- Y.-S. Su and A. Manthiram, Chem. Commun., 2012, 48, 8817–8819 RSC.
- S.-H. Chung and A. Manthiram, Electrochim. Acta, 2013, 107, 569–576 CrossRef CAS.
- Y. Fu, Y.-S. Su and A. Manthiram, Angew. Chem., Int. Ed., 2013, 52, 6930–6935 CrossRef CAS PubMed.
- S.-H. Chung and A. Manthiram, Adv. Mater., 2014, 26, 1360–1365 CrossRef CAS PubMed.
- S.-H. Chung and A. Manthiram, Adv. Funct. Mater., 2014, 24, 5299–5306 CrossRef CAS.
- S.-H. Chung and A. Manthiram, J. Phys. Chem. Lett., 2014, 5, 1978–1983 CrossRef CAS PubMed.
- S.-H. Chung and A. Manthiram, Adv. Mater., 2014, 26, 7352–7357 CrossRef CAS PubMed.
- Y. Yue and H. Liang, Small Methods, 2018, 2, 1800056 CrossRef.
- S.-H. Chung and A. Manthiram, Chem. Commun., 2014, 50, 4184–4187 RSC.
- L. Chen, H. Yu, W. Li, M. Dirican, Y. Liu and X. Zhang, J. Mater. Chem. A, 2020, 8, 10709–10735 RSC.
- J.-Q. Huang, Q. Zhang and F. Wei, Energy Storage Mater., 2015, 1, 127–145 CrossRef.
- A. A. Razzaq, G. Chen, X. Zhao, X. Yuan, J. Hu, Z. Li, Y. Chen, J. Xu, R. Shah, J. Zhong, Y. Peng and Z. Deng, J. Energy Chem., 2021, 61, 170–178 CrossRef.
- Z. Wang, H. Ji, L. Zhou, X. Shen, L. Gao, J. Liu, T. Yang, T. Qian and C. Yan, ACS Nano, 2021, 15, 13847–13856 CrossRef CAS PubMed.
- C. Zhao, Y. Yang, Y. Liu, Z. Sun, T. Zhang, Z. Gao, F. Huo and Y. Zhang, Appl. Surf. Sci., 2024, 644, 158736 CrossRef CAS.
- R. Razaq, M. M. U. Din, D. R. Småbråten, V. Eyupoglu, S. Janakiram, T. O. Sunde, N. Allahgoli, D. Rettenwander and L. Deng, Adv. Energy Mater., 2024, 14, 2302897 CrossRef CAS.
- C. Zhao, K. Amine and G.-L. Xu, Acc. Chem. Res., 2023, 56, 2700–2712 CrossRef CAS PubMed.
- F. Zhao, J. Xue, W. Shao, H. Yu, W. Huang and J. Xiao, J. Energy Chem., 2023, 80, 625–657 CrossRef CAS.
- L. Wang, M. Zhen and Z. Hu, Chem. Eng. J., 2023, 452, 139344 CrossRef CAS.
- G.-T. Yu and S.-H. Chung, Small, 2023, 19, 2303490 CrossRef CAS PubMed.
- Y.-C. Wu and S.-H. Chung, J. Mater. Chem. A, 2023, 11, 9455–9463 RSC.
- J. Lochala, D. Liu, B. Wu, C. Robinson and J. Xiao, ACS Appl. Mater. Interfaces, 2017, 9, 24407–24421 CrossRef CAS PubMed.
- R. Fang, S. Zhao, Z. Sun, D.-W. Wang, H.-M. Cheng and F. Li, Adv. Mater., 2017, 29, 1606823 CrossRef PubMed.
- Z. Han, S. Li, Y. Wu, C. Yu, S. Cheng and J. Xie, J. Mater. Chem. A, 2021, 9, 24215–24240 RSC.
- Y.-J. Yen and S.-H. Chung, Chem. Commun., 2021, 57, 2009–2012 RSC.
- C.-S. Cheng and S.-H. Chung, Chem. Eng. J., 2022, 429, 132257 CrossRef CAS.
- C.-C. Wu and S.-H. Chung, J. Power Sources, 2023, 566, 232944 CrossRef CAS.
- M. Zhao, B.-Q. Li, X.-Q. Zhang, J.-Q. Huang and Q. Zhang, ACS Cent. Sci., 2020, 6, 1095–1104 CrossRef CAS PubMed.
- Y. Fei and G. Li, Adv. Funct. Mater., 2024, 34, 2312550 CrossRef CAS.
- C.-K. Kung and S.-H. Chung, Mater. Horiz., 2023, 10, 4857–4867 RSC.
- S.-H. Chung and A. Manthiram, Electrochim. Acta, 2013, 107, 569–576 CrossRef CAS.
- S.-Y. Chen and S.-H. Chung, Nanomaterials, 2021, 11, 2083 CrossRef CAS PubMed.
- Y.-J. Quay and S.-H. Chung, Nanomaterials, 2021, 11, 3342 CrossRef CAS PubMed.
- X. Chen, Y. Wang, J. Wang, J. Liu, S. Sun, L. Zhu, Q. Ma, N. Zhu, X. Wang, J. Chen and W. Yan, J. Mater. Chem. A, 2022, 10, 1359–1368 RSC.
- C.-H. Yu, Y.-J. Yen and S.-H. Chung, Nanomaterials, 2021, 11, 1518 CrossRef CAS PubMed.
- Y.-C. Huang, H.-I. Hsiang and S.-H. Chung, ACS Sustainable Chem. Eng., 2022, 10, 9254–9264 CrossRef CAS.
- A. B. Puthirath, A. Baburaj, K. Kato, D. Salpekar, N. Chakingal, Y. Cao, G. Babu and P. M. Ajayan, Electrochim. Acta, 2019, 306, 489–497 CrossRef CAS.
- Z.-X. Chen, Q. Cheng, X.-Y. Li, Z. Li, Y.-W. Song, F. Sun, M. Zhao, X.-Q. Zhang, B.-Q. Li and J.-Q. Huang, J. Am. Chem. Soc., 2023, 145, 16449–16457 CrossRef CAS PubMed.
- J. Yang, D. Lee, W. C. Yun, D. W. Kang, Y. Kim and J. W. Lee, Chem. Eng. J., 2023, 470, 144337 CrossRef CAS.
- S. Feng, J. Liu, X. Zhang, L. Shi, C. Anderson, Y. Lin, M.-K. Song, J. Liu, J. Xiao and D. Lu, Nano Energy, 2022, 103, 107794 CrossRef CAS.
- S. Tu, Z. Chen, B. Zhang, X. Wang, R. Zhan, C. Li and Y. Sun, Nano Lett., 2022, 22, 5982–5989 CrossRef CAS PubMed.
- Q. Cheng, Z. Pan, H. Rao and X. Zhong, J. Alloys Compd., 2022, 901, 163625 CrossRef CAS.
- H. Ma, Z. Yu, H. Li, D. Guo, Z. Zhou, H. Jin, L. Wu, X. Chen and S. Wang, Adv. Funct. Mater., 2024, 34, 2310301 CrossRef CAS.
- Y.-J. Wang, C.-C. Hung and S.-H. Chung, Ceram. Int., 2023, 49, 11846–11853 CrossRef CAS.
- T.-M. Hung, C.-C. Wu, C.-C. Hung and S.-H. Chung, Nanomaterials, 2024, 14, 384 CrossRef CAS PubMed.
- Z. He, T. Wan, Y. Luo, G. Liu, L. Wu, F. Li, Z. Zhang, G. Li and Y. Zhang, Chem. Eng. J., 2022, 448, 137656 CrossRef CAS.
- D. Wang, D. Luo, Y. Zhang, Y. Zhao, G. Zhou, L. Shui, Z. Chen and X. Wang, Nano Energy, 2021, 81, 105602 CrossRef CAS.
- Y.-J. Yen, T.-H. Chen, Y.-T. Wang, A. Robles, M. Đerić, O. Š. Miljanić, W. Kaveevivitchai and S.-H. Chung, J. Power Sources, 2023, 565, 232891 CrossRef CAS.
- K. Zhang, X. Li, Y. Yang, Z. Chen, L. Ma, Y. Zhao, Y. Yuan, F. Chen, X. Wang, K. Xie and K. P. Loh, Adv. Funct. Mater., 2023, 33, 2212759 CrossRef CAS.
- S.-H. Chung, Y.-H. Wu, Y.-H. Tseng, T. X. Nguyen and J.-M. Ting, ChemSusChem, 2023, 16, e202300135 CrossRef CAS PubMed.
- Y.-H. Tseng, Y.-C. Lin, Y.-H. Wu, J.-M. Ting and S.-H. Chung, J. Energy Storage, 2023, 68, 107767 CrossRef.
- Y.-C. Lin, Y.-H. Wu, J.-M. Ting and S.-H. Chung, Energy Fuels, 2023, 37, 15162–15169 CrossRef CAS.
- H. Raza, J. Cheng, C. Lin, S. Majumder, G. Zheng and G. Chen, EcoMat, 2023, 5, e12324 CrossRef CAS.
- Z. Wang, H. Ge, S. Liu, G. Li and X. Gao, Energy Environ. Mater., 2023, 6, e12358 CrossRef CAS.
- C.-S. Cheng and S.-H. Chung, Batter. Supercaps, 2022, 5, e202100323 CrossRef CAS.
- H. Park, S. Lee, H. Kim, H. Park, H. Kim, J. Kim, M. Agostini, Y.-K. Sun and J.-Y. Hwang, Carbon Energy, 2024, 6, e472 CrossRef CAS.
- L.-L. Chiu and S.-H. Chung, Polymers, 2021, 13, 535 CrossRef CAS PubMed.
- L.-L. Chiu and S.-H. Chung, J. Mater. Chem. A, 2022, 10, 13719–13726 RSC.
- L.-L. Chiu and S.-H. Chung, Polymers, 2023, 15, 1460 CrossRef CAS PubMed.
- Y.-J. Yang, R. Wang, J.-X. Xue, F.-Q. Liu, J. Yan, S.-X. Jia, T.-Q. Xiang, H. Huo, J.-J. Zhou and L. Li, J. Mater. Chem. A, 2021, 9, 27390–27397 RSC.
- C.-C. Li, W.-P. Wang, X.-X. Feng, Y.-H. Wang, Y. Zhang, J. Zhang, L. Zhang, J.-C. Zheng, Y. Luo, Z. Chen, S. Xin and Y.-G. Guo, ACS Appl. Mater. Interfaces, 2023, 15, 19066–19074 CrossRef CAS PubMed.
- H. Pan, Z. Cheng, P. He and H. Zhou, Energy Fuels, 2020, 34, 11942–11961 CrossRef CAS.
- S. Sun, X. Cui, Q. Ma, J. Wang, M. Ma, X. Yao, Q. Cai, J. Li, X. Chen, Z. Wang, R. Zhuang, P. Mu, L. Zhu, J. Liu and W. Yan, J. Colloid Interface Sci., 2023, 650, 659–668 CrossRef CAS PubMed.
- Y.-C. Huang, B.-X. Ye and S.-H. Chung, RSC Adv., 2024, 14, 4025–4033 RSC.
- C. Shi, T. Hamann, S. Takeuchi, G. V. Alexander, A. M. Nolan, M. Limpert, Z. Fu, J. O’Neill, G. Godbey, J. A. Dura and E. D. Wachsman, ACS Appl. Mater. Interfaces, 2023, 15, 751–760 CrossRef CAS PubMed.
- H. Wan, S. Liu, T. Deng, J. Xu, J. Zhang, X. He, X. Ji, X. Yao and C. Wang, ACS Energy Lett., 2021, 6, 862–868 CrossRef CAS.
- Y.-J. Yen and S.-H. Chung, ACS Appl. Mater. Interfaces, 2021, 13, 58712–58722 CrossRef CAS PubMed.
- Y.-J. Yen and S.-H. Chung, J. Mater. Chem. A, 2023, 11, 4519–4526 RSC.
- L. Zhou, M. K. Tufail, N. Ahmad, T. Song, R. Chen and W. Yang, ACS Appl. Mater. Interfaces, 2021, 13, 28270–28280 CrossRef CAS PubMed.
- H. Liu, P. He, G. Wang, Y. Liang, C. Wang and L.-Z. Fan, Chem. Eng. J., 2022, 430, 132991 CrossRef CAS.
- Y. Li, N. Deng, H. Wang, Q. Zeng, S. Luo, Y. Jin, Q. Li, W. Kang and B. Cheng, J. Energy Chem., 2023, 82, 170–197 CrossRef CAS.
- S. Bandyopadhyay and B. Nandan, Mater. Today Energy, 2023, 31, 101201 CrossRef CAS.
- N. Jayaprakash, J. Shen, S. S. Moganty, A. Corona and L. A. Archer, Angew. Chem., Int. Ed., 2011, 50, 5904–5908 CrossRef CAS PubMed.
- S.-H. Chung and A. Manthiram, Adv. Energy Mater., 2019, 9, 1901397 CrossRef.
- R. Guo, Y. Yang, X. L. Huang, C. Zhao, B. Hu, F. Huo, H. K. Liu, B. Sun, Z. Sun and S. X. Dou, Adv. Funct. Mater., 2024, 34, 2307108 CrossRef CAS.
- Y. Guo, Q. Niu, F. Pei, Q. Wang, Y. Zhang, L. Du, Y. Zhang, Y. Zhang, Y. Zhang, L. Fan, Q. Zhang, L. Yuan and Y. Huang, Energy Environ. Sci., 2024, 17, 1330–1367 RSC.
- X. Liu, J.-Q. Huang, Q. Zhang and L. Mai, Adv. Mater., 2017, 29, 1601759 CrossRef PubMed.
- B. Yan, X. Li, W. Xiao, J. Hu, L. Zhang and X. Yang, J. Mater. Chem. A, 2020, 8, 17848–17882 RSC.
- Q. Zhao, Z. Tu, S. Wei, K. Zhang, S. Choudhury, X. Liu and L. A. Archer, Angew. Chem., Int. Ed., 2018, 57, 992–996 CrossRef CAS PubMed.
- C.-Z. Zhao and Q. Zhang, Trends Chem., 2019, 1, 709–710 CrossRef CAS.
Footnote |
† Electronic supplementary information (ESI) available: Cell-fabrication parameters and the resulting electrochemical-performance values. See DOI: https://doi.org/10.1039/d4cc03085k |
|
This journal is © The Royal Society of Chemistry 2024 |