DOI:
10.1039/D3BM01170D
(Review Article)
Biomater. Sci., 2024,
12, 57-91
Progress of cell membrane-derived biomimetic nanovesicles for cancer phototherapy
Received
13th July 2023
, Accepted 12th September 2023
First published on 13th September 2023
Abstract
In recent years, considerable attention has been given to phototherapy, including photothermal and photodynamic therapy to kill tumor cells by producing heat or reactive oxygen species (ROS). It has the high merits of noninvasiveness and limited drug resistance. To fully utilize this therapy, an extraordinary nanovehicle is required to target phototherapeutic agents in the tumor cells. Nanovesicles embody an ideal strategy for drug delivery applications. Cell membrane-derived biomimetic nanovesicles represent a developing type of nanocarrier. Combining this technique with cancer phototherapy could enable a novel strategy. Herein, efforts are made to describe a comprehensive overview of cell membrane-derived biomimetic nanovesicles for cancer phototherapy. The description in this review is mainly based on representative examples of exosome-derived biomimetic nanomedicine research, ranging from their comparison with traditional nanocarriers to extensive applications in cancer phototherapy. Additionally, the challenges and future prospectives for translating these for clinical application are discussed.
1. Introduction
Since ancient times, cancer has been one of the most serious diseases that endanger human life.1–5 Chemotherapy, radiotherapy, and surgery are some of the traditional treatment options.3 High recurrence rates are caused by the inability of surgeries to remove all tumor tissues entirely. Similarly, most chemotherapies cannot target only cancer cells but severely damage healthy organs, while radiotherapy has a propensity for damaging healthy tissues and cells close to the cancerous cells.6 Because of the advanced methods that have been created over the past decades at the nanoscale, nanotechnology has recently changed the world.7–15 There has been a lot of interest in these new therapeutic approaches with increased efficacy and fewer undesirable side effects.16–22 Along with the fantastic advances achieved in the developing fields of nanotechnology, a wide range of multimodal nanomaterials have been found to improve the quality of our life. To fulfill the increasing requirements for practical translation, some of these nanomaterials were further investigated for broad biomedical applications, and were referred to as “nanomedicine”. The scientific world has paid close attention to these precise nanoplatforms because they have sparked the development of innovative therapeutic strategies for cancer and other critical disorders.23 The three primary groups of currently accessible nanosystems for nanomedicine are organic, inorganic, and organic–inorganic hybridized nanosystems.22,24 The class of nanomedicines has been substantially expanded for various nanomedical applications by the incredible range of their designs, contents, shapes, physical and chemical properties, and bioactivities.25–27
Due to its distinct benefits of convenience, high efficacy, and minimal drug resistance, phototherapy has been extensively investigated in cancer treatment.28–30 To kill the targeted cells in vivo, phototherapy uses phototherapeutic agents (PAs, which include light absorbers and photosensitizers) to transform light energy into chemical or thermal energy.31,32 In phototherapy, the light exposure can be precisely adjusted to irradiate only the target area and provide some tumor selectivity.33 Thus, phototherapies can considerably reduce the damaging consequences to healthy tissue compared with chemotherapy and radiotherapy. Recent research has revealed that phototherapy can effectively stimulate the immune system by causing the immunostimulatory death of tumor cells, which has garnered considerable attention and expanded the use of phototherapy.34–36
Cell membrane-derived nanovesicles are essentially distinct by their size (100–1000 nm in diameter) and production method.37,38 They are phospholipid nanocarriers produced from cells that serve as signalosomes and deliver quantities of bioactive chemicals to particular target cells for signal transduction.39 Nanovesicles derived from cell membrane are now widely acknowledged as key nanovehicles that regulate the biological activity of multicellular animals in an intercellular-transmission way.40,41 They are crucial for regulating many physiological functions, including the survival of stem cells,42 tissue regeneration41,43 and immunological control,44 as well as the pathogenesis that underlies the development of many diseases.45 We now have a much better grasp of cell membrane-derived biomimetic nanovesicle nanotechnology.46 These essential intercellular crosstalk messengers can act as reliable and practical nanocarriers to encapsulate and transfer a functional molecular cargo to cure pathological abnormalities.23 Additionally, they possess inherent biophysical and biochemical features for activating a particular single/chain physiological behavior for the therapy of various diseases through their fascinating endogenous capabilities, such as precise target-homing selectivity and immunosurveillance.41,47 Nanovesicles are thus determined to be a natural nanocarrier with much better biocompatibility and bioavailability than traditional synthetic nanomedicine from a materials perspective, as the cell-derived hierarchical nanoarchitectures of nanovesicle-based biomimetic nanomedicine represent an evolving type of nanocarrier, mounted with a responsive molecule-decorated phospholipid bilayer and an enclosed parental matrix in the same topological configuration.7,48,49 Therefore, it is proposed here that the main concepts for creating cell membrane-derived biomimetic nanovesicles can be derived from conventional synthetic nanomedicines.50 If successful, this technique could combine the innate benefits of the cell membrane-based nanovesicle as a natural nanoparticle with cancer phototherapy enabled by sophisticated nanotechnology. Expediting the advancement of cell membrane-derived biomimetic nanovesicles, numerous evaluations have examined the development of the nanovesicle sector; the majority of them simply pay attention to a few particulars, such as enrichment techniques and biological advancement.44,51–54 We currently have only a partial and incomplete understanding of the precise capabilities of cell membrane-derived biomimetic nanovesicles of unique biostructures and biochemical properties for cancer phototherapy activities.
This review summarizes recent advances in the development of cell membrane-derived biomimetic nanovesicles for cancer phototherapy. The sources of natural cell membranes, the type of nanomedicine core, and their isolation techniques are summarized. The advantages and comparison of cell membrane-based biomimetic nanovesicles with traditional nanocarriers are sequentially discussed, as shown in Fig. 2. The immune escape ability, in vivo prolonged blood circulation time, homologous tumor-targeting ability, and the phototherapy efficacy of nanovesicle-based biomimetic nanomedicine are discussed in detail and compared with the uncoated and traditional nanomedicine approaches (Fig. 1). Finally, potential challenges and perspectives in developing cell membrane-derived nanovesicle-based biomimetic nanomedicine for cancer phototherapy are covered. Table 1 summarizes the recent applications of cell membrane-derived nanovesicle-based biomimetic nanomedicine for cancer PTT. Table 2 summarizes information about the recent applications of cell membrane-derived nanovesicle-based biomimetic nanomedicine for cancer PDT.
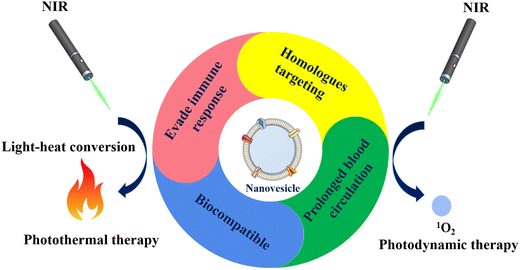 |
| Fig. 1 Diagrammatic representation of nanovesicles derived from cell membrane-built biomimetic nanomedicine for cancer phototherapy. | |
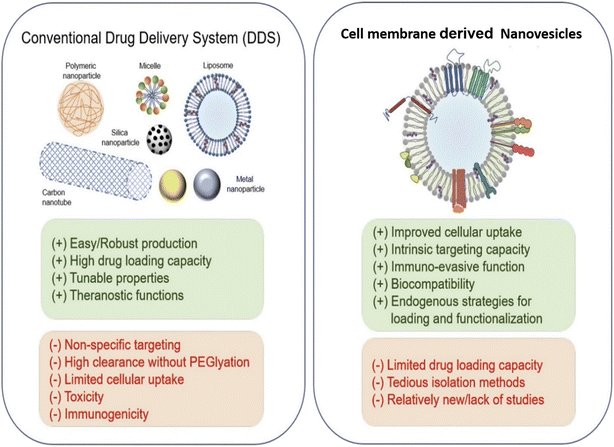 |
| Fig. 2 Comparison between cell membrane-derived nanovesicles and traditional formulations as drug delivery systems (DDS). Reproduced with permission.60 Copyright 2021, Elsevier. | |
Table 1 Summary of the recent applications of cell membrane derived biomimetic nanovesicles for cancer PTT
Different cell membrane sources |
Type of nanomedicine core/nanoplatform |
Drug/imaging agent encapsulated |
Phototherapy type |
Tumor cells |
Ref. |
RBCM |
Black phosphorus quantum dots (BPQDs) |
Programmed cell death protein 1 (PD-1) antibody (aPD-1) |
PTT |
4T1 cells |
125
|
Iron oxide magnetic nanoclusters (MNCs) |
Fe3O4 magnetic nanoclusters |
PTT |
MCF-7 cells |
206
|
Polypyrrole NPs |
BQ123 |
PTT |
HCT 116 cells |
126
|
ICG-HCPT NPs |
10-Hydroxycamptothecin (10-HCPT) and indocyanine green (ICG) |
PTT |
HeLa cells |
207
|
Melanin NPs |
Melanin |
PTT |
A549 cells |
129
|
Hollow mesoporous Prussian blue NPs |
Doxorubicin (DOX) |
PTT |
4T1 cells |
130
|
Prussian blue NPs |
Gamabufotalin (CS-6) |
PTT |
NIH-3T3, -MB-231, HeLa, HCT-116, and BGC-823 cells |
131
|
Macrophage membrane |
Gold NPs |
Doxorubicin |
PTT |
B16F10 and DC2.4 cells |
208
|
AuNR (gold nanorods) @RGD-Exosomes |
Doxorubicin |
PTT |
HeLa cells |
209
|
Magnetic iron oxide NPs (Fe3O4 NPs) |
Iron oxide (Fe3O4) |
PTT |
MCF-7 cells |
210
|
Hollow bismuth selenide NPs |
Quercetin |
PTT |
4T1 cells |
139
|
Nanodots |
NIR-II Aggregation-induced emission (AIE) photothermal agents |
PTT |
4T1 cells |
211
|
Platelet membrane |
Photothermal-sensitive liposomes |
Glucose oxidase and ferric ammonium |
PTT |
HepG2 and H22 cells |
149
|
PLGA NPs |
Doxorubicin and Indocyanine green (ICG) |
PTT |
MCF-7, MDA-MB-231, and CAMA-1 and HeLa cells |
212
|
Polypyrrole NPs |
Polypyrrole and doxorubicin |
PTT |
Huh7 cells |
213
|
Fe3O4 magnetic NPs |
Fe3O4 |
PTT |
MCF-7 cells |
214
|
Cancer cell membrane |
Aggregation-induced emission (AIE) NPs |
AIE fluorophores |
PTT |
CT26, Hep1–6, and NIH3T3 cells |
175
|
Superparamagnetic iron oxide and PLGA Nps |
Hematoporphyrin monomethyl ether (HMME) |
PTT |
4T1 cells |
173
|
Porous silicon nanoparticles, i.e., MSNs |
ICG and DOX |
PTT |
4T1 cells |
170
|
Vanadium carbide quantum dots |
TAT peptides |
PTT |
NHDF, MCF-7, and A549 cells |
215
|
Black phosphorus quantum dots (BPQDs) |
BPQDs |
PTT |
U251, Hepa1–6, LLC and 4T1 cells |
180
|
PLGA NPs |
ICG |
PTT |
HepG2, A549, MCF-7 and MB231 cells |
172
|
Black-titanium NPs |
Iridium complexes |
PTT |
HeLa cells |
171
|
Stem cell membrane |
Exosomes |
ICG and curcumin (CUR) |
PTT |
U87MG cells |
193
|
Iron oxide (Fe3O4) NPs |
Polydopamine (PDA) and siRNA |
PTT |
DU145 cells |
216
|
Hybrid membrane |
Hollow polydopamine NPs |
PDA |
PTT |
B16-F10 |
201
|
Iron oxide magnetic NPs |
Iron oxide |
PTT |
Head and neck squamous cell carcinoma |
202
|
Polypyrrole NPs |
Polypyrrole NPs |
PTT |
HCT116 cells |
203
|
Melanin NPs |
Melanin |
PTT |
MCF-7 cells |
204
|
Hollow copper sulfide NPs |
Copper sulfide and sorafenib |
PTT |
HCC cells |
205
|
Others |
Gold nanocage (AuNC) |
DOX and ICG |
PTT |
MDA-MB-231 and 4T1 cells |
167
|
EVs |
Paclitaxel and ICG |
PTT and PDT |
MCF-7 cells |
217
|
Giant membrane vesicles (GMVs) |
ICG, AS1411 and TMPyP4 |
PTT and PDT |
CCRF-CEM cells and HeLa cells |
218
|
Table 2 Summary of the recent applications of cell membrane-derived biomimetic nanovesicles for cancer PDT
Different cell membrane sources |
Type of nanomedicine core |
Drug/imaging agent encapsulated |
Phototherapy type |
Tumor cells |
Ref. |
RBCM |
Exosomes |
Chimeric peptide (ChiP) |
PDT |
4T1 and CT26 cells |
240
|
PLGA NPs |
DOX and methylene blue |
PDT |
HeLa, MCF-7, and HEK293 cells |
221
|
Copolymer methoxypoly(ethylene glycol)2 Kblock-poly(D,L-lactide)2 K (PEG-b-PDLLA NPs) or simply Dimeric Prodrug NPs |
5,10,15,20 Tetraphenylchlorin (TPC) and Paclitaxel |
PDT |
HeLa cells |
241
|
Cancer cell membrane |
Tumor exocytosed exosome/AIE luminogen (AIEgen) hybrid nanovesicles (DES) |
Dexamethasone (DEX) |
PDT |
4T1 cells |
258
|
Exosome |
Chlorin e6 (Ce6) |
PDT |
MIA-PaCa-2 and B16F10 cells |
259
|
EVs |
DOX |
PDT |
PC-3, ATCC ® CRL-1435™ |
111
|
Porphyrin metal–organic framework (MOF) |
Glucose oxidase (GOx) and catalase |
PDT |
T1 cells, B16F10 cells, and HepG2 cells |
231
|
Porphyrinic (MOF) (PCN-224) |
Tirapazamine (TPZ) |
PDT |
4T1 cell |
230
|
Stem cell membrane |
EVs |
meta (Tetrahydroxyphenyl)-chlorin (mTHPC) |
PDT |
CT26 cells and ID8 cells |
263
|
Upconversion NPs (UCNPs) |
ZnPC and MC540 |
PDT |
HeLa cells |
261
|
Gelatin nanogels |
Ce6 |
PDT |
A549 cells |
262
|
Macrophage cell membrane |
AIE dots |
AIE photosensitizers i.e., MeTIND-0, MeTIND-2, and MeTIND-4 |
PDT |
4T1 cells |
277
|
Platelet cell membrane |
PLGA NPs |
Verteporfin |
PDT |
4T1 cells |
278
|
|
W18O49 NPs |
W18O49 and metformin |
PDT and PTT |
Raji cells, a Burkitt's lymphoma cell line |
279
|
Hybrid membrane |
LSCMR NPs |
Ce6 and chikusetsusaponin IVa methyl ester |
PDT |
4T1 cells |
275
|
|
LPHM@DDI NPs |
IR780 and DOX |
PDT and PTT |
4T1 cells |
276
|
Other |
Au NPs |
Ce6 and BSA |
PDT |
MGC-803 cells |
280
|
2. Comparison of cell membrane-derived nanovesicles with traditional nanocarriers
Researchers can evaluate the benefits and drawbacks of nanovesicles derived from cell membrane and conventional nanocarriers by closely contrasting their biological characteristics. FDA-approved conventional nanocarriers for the clinical administration of chemotherapeutics have been explored in cancer treatment.55,56 Traditional nanoparticles are simple to produce, resulting in a high yield and beneficial flexibility in separation and purification. However, their widespread use has been hampered by their toxicological properties and lack of targeted selectivity. If exosomes are created by normal cells, their biocompatibility and low toxicity are the most desirable features. Nanovesicles as a therapeutic method of delivery are still in their infancy, even though the area of synthetic nanoparticles remains relatively new. There are not many investigations that compare the two in parallel, even though a recent study found that nanovesicles are more effective in targeting tumors than liposomes.57 The only lingering query is whether the liposomes utilized in the study had received clinical approval. An extensive comparison of all the numerous forms of liposomes, including anionic, cationic, PEGylated, ligand-modified, pH-sensitive, etc., with exosomes may be required to demonstrate their effectiveness. Another factor in comparison between nanovesicles and artificial nanoparticles is drug encapsulation efficiency. Compared with unloaded synthetic nanoparticles, nanovesicles are naturally packed and loaded with various compounds, making it challenging to obtain high drug-loading efficiency.58 However, external and internal loading techniques, like electroporation with intrinsic loading and a biogenesis process, may increase the drug-loading efficiency of exosomes.55 Nanovesicles could be tailored to carry specific payloads of importance using biological methods, such as selective packing in the nanovesicle-producing cells. Both artificial nanoparticles and nanovesicles can be improved in terms of biostability and tumor targeting. Nanovesicles’ propensity for bioengineering and naturally occurring surface membranes depend on each other. For instance, a recent study showed that nanovesicles with the signal regulatory protein (SIRP) on their surface can avoid immune recognition,56 and display biostability and a prolonged circulation period. We hypothesize that bioengineered surface molecules represented on the lipid bilayer membranes of nanovesicles would be more biologically compatible and active, as shown in Fig. 2. In contrast, peptides or targeting molecules loaded in synthetic nanoparticles frequently lose their conformation and binding activity. Since RGD is the peptide most frequently used to alter liposomes, it might be abundantly engineered on nanovesicles with a better affinity and binding accuracy to target tumor cells than liposomes.59
3. Cell membrane-derived nanovesicles for cancer phototherapy
Photodynamic therapy (PDT) and photothermal therapy (PTT) are the two broad categories of phototherapy, and can be generally classified based on their mechanism of action. PDT is the process of using a photosensitizer to produce reactive oxygen species (ROS) in the presence of light, which causes oxidative harm to cells.61 PTT does not require the use of oxygen, in contrast to PDT. The elevated local temperature immediately destroys the tumor by converting light into heat with the aid of a photothermal agent (PA). As a result, hypoxic tumors that are resistant to PDT can be treated with PTT.62,63 Different PAs with intriguing optical characteristics have been created during recent years to treat tumors, and have shown a lot of promising findings.64,65 To maximize the effects of phototherapy, selection of the PA is crucial. Strong light stability, precise absorption wavelength, and high energy conversion efficiency are all characteristics of the perfect PA.66 For PDT and PTT, conventional nanomedicines, for example dendrimers,25 micelles,67 liposomes,47 mesoporous silica nanoparticles (MSNs),68 silver nanoparticles,69,70 carbon nanotubes,71,72 magnetic nanoparticles,73 2D materials,74 quantum dots,75 metal–organic frameworks (MOFs),76 and inorganic 2D nanosheets,45 have been recently reported with efficient drug loading ability. Compared with conventional chemo and radiotherapy, nanomedicine-based PTT and PDT have increased therapeutic specificity and decreased toxic side effects due to their inherent light-induced toxicities.28,77 However, several drawbacks restrict the use of PTT or PDT in medical settings. For instance, nanoparticles are frequently perceived as invaders after being introduced into the body, which triggers an immune reaction against them.78,79 The mononuclear phagocyte system (MPS) is expected to recognize and remove nanoparticles during blood circulation, shortening circulation duration and limiting therapeutic effects.80 Also, they accumulate in healthy organs.81,82 In order to facilitate immune evasion, extend blood circulation, and improve tumor targeting, surface modification of nanoparticles is a typical method.83 The current industry standard for coating materials is poly(ethylene glycol) (PEG) coating of nanoparticles.84 However, it has been discovered that anti-PEG antibodies are produced, which causes rapid clearance from the blood circulation.85 Surface modification using a cellular membrane is an evolving technique to give nanomedicine excellent biointeraction properties. This technique has received a lot of interest in the nanomedicine field, particularly in the areas of delivery of drugs,86,87 imaging,88,89 detoxification,90 vaccination91 and phototherapy.92 The interior of the biomimetic nanomedicine is made of synthetic materials, while the outer layer is made of a cell membrane.93 The layer of the natural cell membrane may inherently resemble the antigenic variety of the source cells, resulting in a range of source cell functions like immune escape and long circulation.94,95
PTT is a unique hyperthermia technique for cancer therapy that uses Nano mediators to transform light into heat to produce the best therapeutic results.96 PTT can destroy the malignant area locally while causing the least harm to the untargeted tissue when combined with near-infrared (NIR) light.97,98 PTT has gained attention in biomedical applications due to its non-invasive nature, excellent spatial and temporal control, and lack of observed resistance. A variety of nanomediators have been used for this objective, including gold-based nanomaterials (such as nanoshells, nanorods, and hollow nanospheres),99 NIR dyes (such as indocyanine green, IR780),100,101 metal sulfides102,103 and carbon-based nanomaterials.104,105 The possibility of imaging mode-integrated photothermal conversion (PTC) agents for diagnosis and concurrent therapy outcome tracking has also drawn great interest.106
Dependent on the photochemical reaction caused by photosensitizers (PS) under light irradiation, PDT, a light-based cytotoxic therapy, has now been demonstrated as viable alternative treatment for cancer.107,108 The excited PS shifts from a baseline singlet electronic state to a lesser or higher electronically singlet state upon exposure to light of a particular wavelength and then passes through the intersystem crossover to the excited triplet state. This is the basis of the PDT theory. When the ground-state triplet molecular oxygen collides with the triplet PS, the triplet molecular oxygen is excited to the singlet electronically excited state, where it produces ROS, such as superoxide anion (O2−), hydroxyl radicals (OH), and hydrogen peroxide (H2O2).109 By causing several harmful processes, such as denaturation of proteins inside cellular membranes and organelles, lipid peroxidation, and mitochondrial membrane potential malfunction, ROS plays significant roles in PDT in cancer treatment.110 The primary factors affecting PDT efficacy are PS, light, and oxygen content in tissues.107 Following PDT, cancer cells can quickly create exosomes in large quantities, according to recent research.111 Specifically, after PDT at sub-lethal dosages, the data indicate that cytotoxic effects could induce cell membrane-derived nanovesicle secretion.
4. Recent applications of biomimetic exosomes for cancer phototherapy
Because of its proven effectiveness and comparatively low invasiveness, cancer phototherapy has gained more and more attention. Many different nano-delivery technologies have been developed over the past few years to improve phototherapy techniques. To boost therapeutic efficacy from the perspectives of direct tumour destruction and better delivery processes assisted by nano delivery designs, this section presents cancer phototherapy techniques, including PTT and PDT, using cell membrane-generated biomimetic nanovesicles.
4.1 Photothermal therapy (PTT)
In this part (as summarized in Table 1), we will cover the recent applications of cell membrane-derived biomimetic nanovesicles in cancer PTT.
4.1.1 Red blood cell-derived nanovesicles.
To achieve extended blood retention and reduce macrophage absorption, red blood cell (RBC) manipulation has been suggested as a biomimetic technique.112,113 RBCs can efficiently provide their dependent or inner components with prolonged blood survival and immune evasion skills because of their inherent surface, including CD47, different membrane proteins, acidic sialyl moieties, and glycans.114–117 To attain overall improved in vivo stability for imaging applications, some research groups effectively loaded RBCs with magnetic particles.118,119 To enhance blood retention, Wang et al. have also shown a multimodal platform embellishing RBCs with various moieties.99 However, only structures with sizes that remained at the micrometer level could incorporate RBCs’ cloaking capabilities.120 Zhang et al. recently created a revolutionary strategy to fit this “RBCs getup” within the nanoscale range.99 It has been shown that various nanomaterials, including polymeric nanoparticles and gold nanoparticles, can be encapsulated into nano-sized RBC membrane (RBCM)-derived vesicles to obtain prolonged blood retention and the improved permeability and retention (EPR) effect, which is a special benefit of nanoparticles in cancer research.121–123 Additionally, this cell membrane encapsulation method has been shown to result in slower blood clearance than the PEGylated equivalents124 and can be combined with active targeting ligands for targeted therapy.121
Even with conventional chemical and anti-human epidermal growth factor receptor (HER) therapy, basal-like breast cancer has a depressing prognosis and a triple-negative character. But this stubborn cancer type's high mutation rate makes it amenable for immunotherapy. A highly effective technique for causing tumor neoantigen release in situ is photothermal therapy, which has a lot of potential in cancer immunotherapy. In order to achieve this goal, the Liang et al. study team created a biomimetic black phosphorus quantum dot (BPQD) formulation that uses near-infrared (NIR) laser irradiation to elicit breast cancer cell apoptosis in situ and activate the immune system to destroy metastatic and residual cancer cells. The BPQDs were coated with RBCM, creating a biomimetic BPQD-RM nanovesicle (BPQD-RMNV) with a long circulatory period and accumulation in tumor in vivo, as illustrated in Fig. 3. The basal-like 4T1 breast tumor suffered necrosis and apoptosis with radiation treatment, and dendritic cells (DCs) were mobilized to collect tumor antigens in vivo. Additionally, CD8+ T lymphocytes were preserved from exhaustion using an antibody against programmed cell death protein 1 (aPD-1).125 Notably, BPQD-RMNV-mediated PTT and aPD-1 therapy markedly inhibited in vivo growth of residual and metastatic tumors. Therefore, immune checkpoint inhibition antibody and biomimetic BPQD-RMNV-mediated PTT enhanced CD8+ T-cell recruitment and engagement in cancer, which directly inhibited basal-like breast tumor development in vivo.125
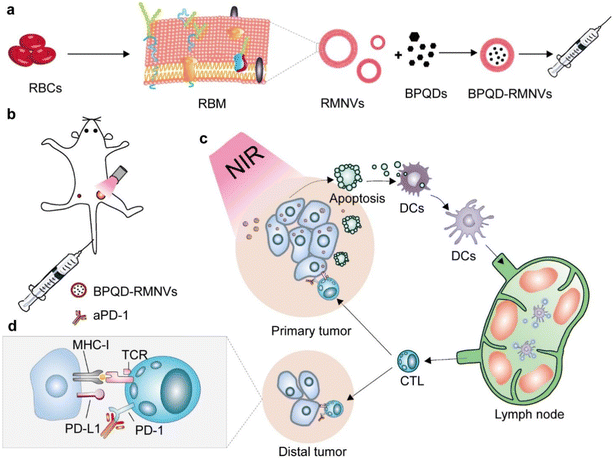 |
| Fig. 3 Diagrammatic representation of photothermal cancer immunotherapy facilitated by BPQD-RMNVs and aPD-1. (a) Preparation of BPQD-RMNVs. (b) In vivo treatment of 4T1 tumor-bearing mice with BPQD-RMNV-mediated PTT and aPD-1 therapy. (c) In situ induction of tumor cell apoptosis. (d) Tumor ligand PD-L1 involvement. Reproduced with permission.125 Copyright 2019, Elsevier. | |
Recently iron oxide nanoparticles have been found effective for photothermal conversions, and have attracted growing attention among researchers, in addition to their inherent benefits for magnetic resonance imaging (MRI). However, similar to other inorganic nanomaterials, their utilization in vivo has been severely limited by rapid macrophage absorption, brief blood retention times, and undesirable biodistributions. The research team led by Ren and colleagues developed a reasonable design for RBCM-cloaked iron oxide magnetic clusters (MNC@RBCs) to address these issues. The results demonstrated that by simply adding a “ultra-stealth” biomimetic coating to iron oxide magnetic nanoclusters (MNCs), MNC@RBCs maintained the image analysis and photothermal capabilities inherited from MNC cores while attaining significantly decreased nonspecific macrophage absorption (Fig. 4). When administered intravenously to mice, MNC@RBCs show improved blood retention time, high tumor accumulation, and reduced liver biodistribution. This study demonstrates a carefully designed combination of MNCs and RBCs, utilizing the benefits of both capabilities in a single entity, and indicates a good prospect for the use of iron-based nanomaterials in vivo.99
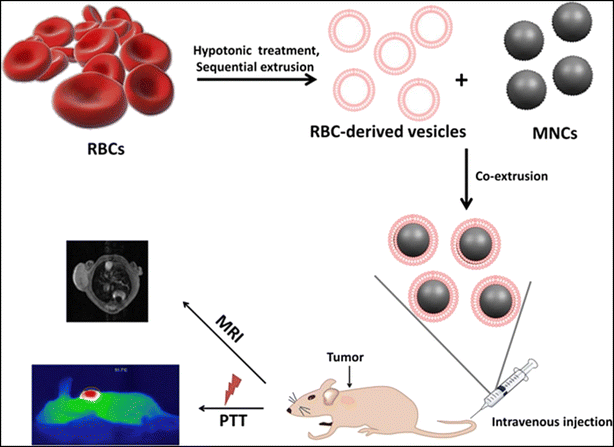 |
| Fig. 4 Illustration of the preparation method of MNC@RBCs and its uses for MRI and PTT in mice. Reproduced with permission.99 Copyright 2016, Elsevier. | |
Through the targeted dilation of tumor vasculature, Wang et al. proposed a method to increase the delivery effectiveness of biomimetic photothermal nanoagents for better photothermal therapy. A biomimetic layer of true RBCM was coated onto the exterior of photothermal polypyrrole nanoparticles (PPy@RBCs NPs) utilizing a simple nanocoating method. This enhanced blood retention time, as the nanoparticles inherited the immune escape ability from natural RBCs. Additionally, the PPy NPs cores are responsible for the superb photothermal and photoacoustic imaging capabilities that were all preserved. The endothelin A (ETA) receptor antagonist BQ123 was also used to control the tumor microenvironment, further enhancing the photothermal result. By altering an ET-1/ETA transduction pathway and inhibiting the ETA receptor, the BQ123 could promote tumor vascular relaxation and improve blood flow perfusion, but it did not affect the vessel perfusion of normal tissues. When used consistently, this smart strategy significantly increased the amount of biomimetic PPy NPs in the tumor location. The research demonstrated that biomimetic PPy NPs coupled with the specific antagonist BQ123 had a potent antitumor effect and outperformed biomimetic PPy NPs and PEGylated PPy NPs with BQ123, exhibiting a significantly improved photothermal treatment which could be attained with low-dose photothermal agent administration. By inhibiting the ETA receptor, these results offer a promising method for future boosted photothermal treatments of a similar nature.126 Because surface proteins and membrane structures are reserved in nanoparticles concealed by RBCM, they confer significant cell-specific functions such as biocompatibility, extended circulation lifetime, and reduced reticular endothelial system (RES) absorption ability. Considering the negative aspects of carrier-free nanomedicine, such as its low stability, lack of immune evasion function, and harmful drug burst release, Ye et al. developed and fabricated a novel RBCM biomimetic combinational therapeutic system by entrapping the low molecular drug co-assemblies of 10-hydroxycamptothecin (10-HCPT) and indocyanine green (ICG) in the RBC vesicles for prolonged circulation, controlled release, and synergistic chemoPTT. The self-reorganized RBCs@ICG-HCPT nanoparticles (NPs) had a diameter of about 150 nm, a core–shell morphology, a high drug payload of around 92 percent by weight, and a diminished ability to be absorbed by the reticuloendothelial system (RES). RBCs@ICG-HCPT NPs significantly increased the concentration at the tumor locations by passive targeting accompanied by cellular endocytosis, using the stealth capabilities of RBCM. RBCs@ICG-HCPT NPs showed great instability by heat-mediated membrane breakdown and pH change in response to near-infrared laser stimulation coupled with acidic stimulus.127 This prompted fast disintegration and hastened drug release. RBCs@ICG-HCPT NPs under dual-stimuli achieved highly effective apoptosis in cancer cells and outstanding tumor ablation by chemoPTT. This carrier-free, tiny molecule drug co-assembly-based biomimetic nanoplatform, which integrates imaging capability as a promising theranostic system, may be used for cancer detection and combination therapy.128 PTT has recently emerged as an intriguing noninvasive strategy for cancer treatment. The development of non-toxic, biodegradable biomaterials with significant in vivo photothermal performance still faces difficulties. RBCM-camouflaged melanin (Melanin@RBCs) nanoparticles (Fig. 5) were created as a platform for in vivo antitumor PTT by Jiang et al. as they investigated natural melanin nanoparticles isolated from living cuttlefish as efficient photothermal agents. The naturally produced melanin nanoparticles showed substantial NIR absorbance, a greater photothermal conversion efficacy (about 40%) than synthetic melanin-like polydopamine nanoparticles (about 29%), and excellent biodegradability and biocompatibility. It has been demonstrated that melanin nanoparticles with RBCM wrapping preserved their superior photothermal properties, had improved blood circulation, and significantly increased their accumulation at tumor sites. Approximately 4 h after intravenous injection, the ideal concentration of Melanin@RBCs at tumors was reached under the supervision of their inherent photoacoustic imaging ability. The developed Melanin@RBCs nanoparticles demonstrated noticeably more PTT efficiency than plain melanin nanoparticles in A549 tumor-bearing mice after exposure to an 808 nm laser.129
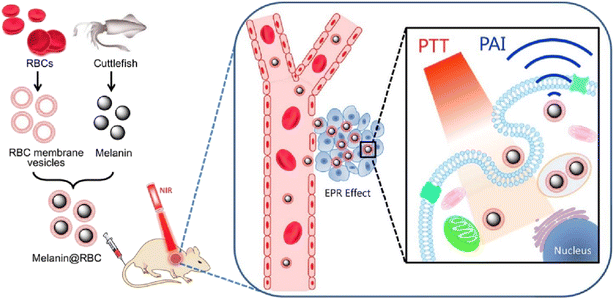 |
| Fig. 5 Schematic representation of RBCM cloaked melanin NPs for better PTT. Reproduced with permission.129 Copyright 2017, Elsevier. | |
Over the last few decades, cancer therapy primarily based on nanodrugs has been developed. Poor drug-loading capacity, speedy blood clearance, and low antitumor effectiveness with a significant risk of recurrence are the main problems that nanodrugs face. For this reason, Chen et al.'s study team created hollow mesoporous Prussian blue nanoparticles (HMPB@RBCs NPs) that mimic RBCM for cancer combination treatment. Compared with plain HMPB NPs, HMPB@RBCs NPs have dramatically improved stability, immune evasion, and blood residence time. Model drug doxorubicin (DOX), with a loading capability of up to 130% weight, was encapsulated within HMPB@RBCs NPs. Furthermore, the pH-/photoresponsive release was seen in HMPB@RBCs NPs loaded with DOX. The DOX@HMPB@RBCs NPs exhibited exceptional effectiveness in synergistic photothermal/chemotherapy of cancer, as shown by the in vivo experiments.130 Gamabufotalin (CS-6), an anti-cancer agent, has been released non-targeted, which makes it challenge to treat patients with standard chemotherapy because it can have significant adverse effects. Due to their substantial advantages of high loading efficiency, adjustable release behavior, and targeted accumulation at tumor areas, the creation of innovative nanodrug systems has recently garnered more and more interest. Liu et al. created the nanodrug system known as HA@RBCs@PB@CS-6 NPs (HRPC). Prussian blue nanoparticles (PB NPs) having a hollow porous structure were utilized in this approach as a dual-purpose carrier for CS-6 and a photothermal sensitizer. The outcome showed that the RBCM implementation on the PB NPs increased the immune escape ability by more than 60% while also extending the circulation life to 10 h. Additionally, they revealed that the loading drug of CS-6 carried out part of its highly potent anticancer activity by significantly lowering the expression of HSP70, which increased the effectiveness of the photothermal therapy. The in vivo investigation showed that HRPC performed exceptionally well in simultaneous photothermal/chemotherapy of cancer with no adverse effects on normal tissues.131
4.1.2 Macrophage-derived nanovesicles.
Due to their role as circulating guardians for the phagocytosis of foreign substances and immunological function, macrophages, a kind of white blood cell (WBC), have stimulated the construction of numerous functional nanocarriers.132,133 It becomes conceivable to use this cell–cell adhesion for efficient cancer targeting if macrophage membranes can conceal nanoparticles.134,135 Using cell membranes for nanoparticle surface modification is a top-down strategy that takes advantage of the rapid growth of nanotechnology and nanoscience and completely replicates the membrane antigens from actual cells for fabricated nanoparticles.112,136 According to Li and coworkers, the connections between the macrophage's 4 integrins and the cancer cells’ vascular cell adhesion molecule-1 (VCAM-1) enable native macrophages to attack lung metastases of breast cancer directly.132 To develop personalized, precise cancer treatment in the future, the adaptable design of exosomes could offer a functional basis for their manufacture with more varied characteristics from synthetic materials.137
The detection and management of cancers could be revolutionized by nanotechnology. Prolonged blood circulation and active cancer targeting are desirable characteristics for nanoparticles utilized for in vivo cancer therapy. They should also be undetectable to the immune system and harmless. To treat cancer, Meng et al. created a biomimetic nanoplatform with the features above. Vesicles made from rebuilt macrophage membranes were then coated over magnetic iron oxide nanoparticles (Fe3O4 NPs). The resulting Fe3O4@MM NPs had excellent biocompatibility, immune evasion, cancer selectivity, and light-to-heat conversion properties, since they were derived from the Fe3O4 core and the macrophage outer shell. Biomimetic Fe3O4@MM NPs were additionally used for highly efficient photothermal therapy of breast cancer in naked mice due to their good in vitro and in vivo features. Surface treatment of synthetic nanomaterials with biomimetic cell membranes demonstrates an innovative method for creating the optimal nanoplatform for translational medicine.138 The main obstacles to traditional nanodrug-based tumor therapy include poor tumor accumulation, fast removal from the systemic circulation, and a significant risk of metastasis and invasion. Fig. 6 illustrates the hollowed bismuth selenide nanoparticles (abbreviated as M@BS-QE NPs) that Zhao et al. research team created for combination treatment of breast cancer. Compared with bare BS NPs, the resultant M@BS-QE NPs exhibited prolonged circulation life, accelerated and improved tumor accumulation, immune evasion properties, C–C chemokine ligand 2 (CCL2)-mediated recruiting properties, and active targeting potential. By lowering heat shock protein 70 (HSP70), one malignancy-specific, overexpressed thermal resistance-related chaperone, the consequent QE liberation under near-infrared (NIR) laser irradiation could sensitize cancer cells to photothermal therapy. To reduce breast cancer lung metastasis, M@BS-QE NPs downregulated the signaling pathways for p-Akt and matrix metalloproteinase-9 (MMP-9), which breaks down the extracellular matrix to promote tumor invasion and metastasis. Consequently, these findings offer a biomimetic approach that holds great promise for enhancing therapeutic efficacy and improving the validity of treatment with minimal adverse effects on both primary and lung metastasis of breast cancer.139 This approach uses the traits of breast cancer and the biological characteristics of macrophages.
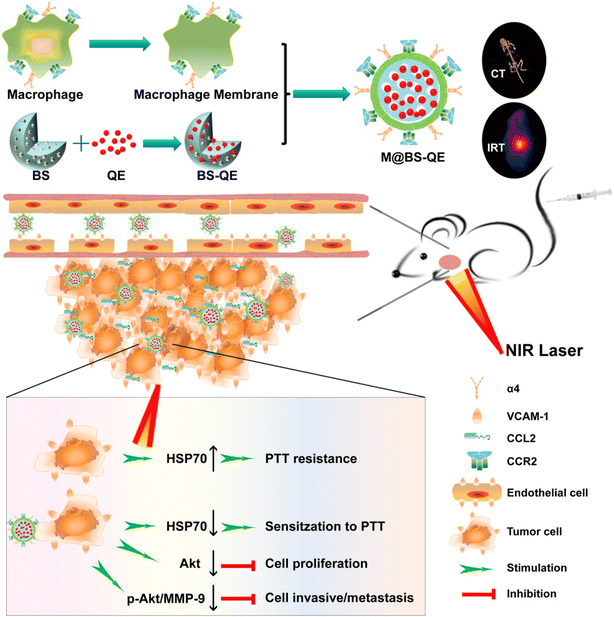 |
| Fig. 6 Schematic diagram of Biomimetic Synergistic M@BS-QE preparation. Tumor proactive recruitment/targeting ability and combinatory anti-cancer effect for simultaneous PTT and metastatic breast cancer therapy. Reproduced with permission.139 Copyright 2018, American chemical Society. | |
4.1.3 Platelet-derived nanovesicles.
Drug delivery technologies have reduced side effects while enhancing biocompatibility, pharmacokinetics, and therapeutic efficacy.140,141 Due to their comparable homing characteristics or self-expressed signal molecules, biomimetic nanoparticles are very effective at targeting tumors.142,143 Similarly, because of their inherent existence in vivo, good biocompatibility and lengthy circulation times are simultaneously achieved. Platelets can target areas of vascular damage in the body to prevent thrombogenesis and preserve the integrity of blood circulation.144 Platelets could communicate “don't eat me” signals for self-recognition to the CD47 membrane protein in vivo, preventing macrophage phagocytic uptake.145 Additionally, platelets overexpress P-selectin, enabling platelets to clump together on tumor cells by binding directly to the elevated CD44 receptors on the surface of cancer cells.136,146 Platelets, which have a long lifespan in the circulation, the ability to adhere to cancer cells, and respond to vascular damage, produce platelet exosomes.147 Regarding internalizing tumor cells as nanoparticles, platelet exosomes outperform metrocytes. Additionally, blood contains many platelet exosomes, easily accessible as drug delivery vehicles.148 Platelet exosomes are efficient at carrying drugs, but their limited drug-carrying capacity prevents them from being used in more settings.149 Because of spreading of the tumor, breast cancer is linked to a significant death rate. The ineffectiveness of photochemotherapy in targeting circulating tumor cells (CTCs) in the lymphatic system and blood restricts its potential to prevent metastasis. To overcome this, the study team of Ye et al. embellished platelet membrane (PM) on the surface of nanoparticles, known as nanoplatelet vesicles, as depicted in Fig. 7. The biomimetic nanoplatelets are co-encapsulated with the chemotherapeutic agent doxorubicin and the FDA-approved photothermal agent indocyanine green (ICG). Through high-affinity associations between the P-Selectin of PM and the CD44 receptors of tumor cells, nanoplatelet vesicles have the potential to escape immune surveillance and specifically trap and eliminate CTCs in both blood and lymphatic circulations. Compared with uncoated NPs, PM-coated NPs display better cellular absorption by MDA-MB-231 breast cancer cells and cause higher cytotoxicity levels in tumor cells. It was found that in three proven xenograft or orthotopic breast tumor-bearing mouse models, the multipurpose nanoplatelet vesicles eradicated the primary tumor and greatly suppressed breast cancer spread. This example indicates that biomimetic nanoplatelets offer a promising method of actively capturing and destroying CTCs in blood and lymph in breast cancer therapy.150
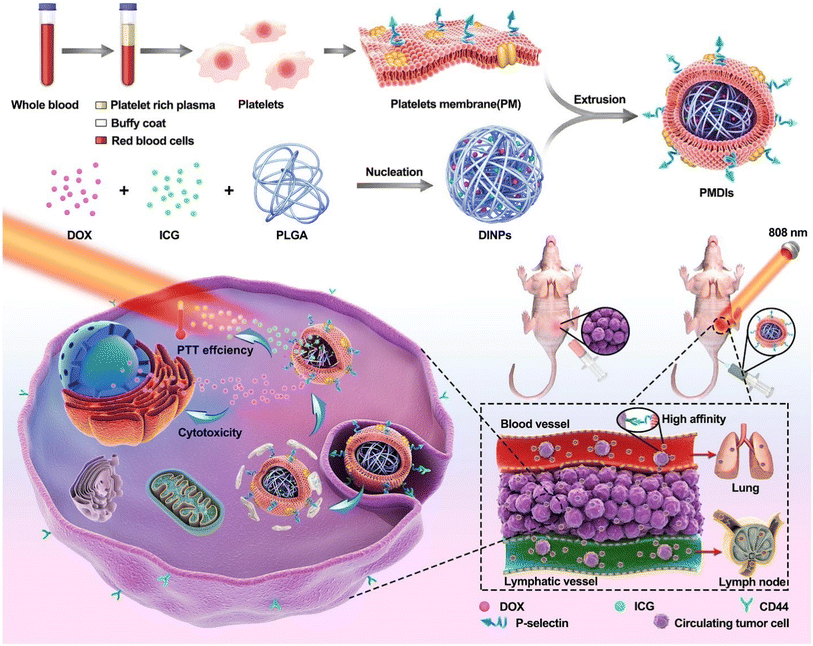 |
| Fig. 7 Preparation method of nanoplatelet vesicles and their synergistic effects against metastatic breast cancer cells with additive chemo and PTT. Reproduced with permission.150 Copyright 2019, Elsevier. | |
Two of the biggest obstacles to hepatocellular carcinoma (HCC) therapy, particularly after metastasis, are targeted drug delivery and the controlled release of therapeutics at tumor locations. In a platelet membrane-facilitated localized chemophotothermal therapeutic method, Wu et al. incorporated doxorubicin (DOX) and polypyrrole (PPy) nanoparticles that served as photothermal agents into platelet membrane vesicles (PLT-PPy-DOX). The particles were intravenously administered into an orthotopic animal model of HCC and given immune evasion and tumor-targeting properties from platelet membrane vesicles. PLT-PPy-DOX nanoplatforms were widely distributed in the tumor tissues, as predicted. To boost the prompted release of DOX as well as directly destroy tumor cells, hyperthermia was produced under laser irradiation (808 nm). This combination of regional chemotherapy and photothermal therapy showed outstanding antitumor effectiveness in reducing the development of the primary tumor and preventing metastases. This localized therapy, which uses organic photothermal agents that are well biodegradable and biocompatible natural cell membranes, may offer innovative perspectives for creating biomimetic nano-vehicles to treat HCC on an individual basis.151 Nanoparticles could revolutionize cancer treatment and diagnosis. Theranostic nanoplatforms must have active cancer targeting and extended systemic circulation. They must also be invisible to the immune system, and harmless. An all-in-one nanoplatform with these qualities was created by the research team of Rao et al. for individualized cancer theranostics. Platelet-derived vesicles (PLT-vesicles) were collected from mouse blood, and were subsequently coated, with their membrane proteins, onto Fe3O4 magnetic nanoparticles (MNs). The resulting core–shell PLT-MNs were then infused into the donor mice for improved tumor magnetic resonance imaging (MRI) and photothermal therapy. They acquired the prolonged blood flow and cancer-targeting abilities from the PLT membrane, and the MN core's magnetic and optical absorption characteristics (PTT). Meanwhile, it was discovered that PLT-MN targeting to PTT locations (i.e., tumor sites) is induced by PTT treatment, and this improved targeting of PLT-MNs to tumor sites can augment PTT effects. Furthermore, PLT-MNs exhibited outstanding immunological compatibility. This research offers a fresh perspective on creating biomimetic nanoparticles for targeted disease diagnosis and treatment.152 Using a biomimetic drug delivery system (DDS) opens up new possibilities for simulating biological elements to improve the accuracy of tumor targeting and advance treatment efficacy. In order to create a laser-controlled nanosystem (FG@PEL), Ma et al.'s research team combined platelet exosomes and photothermally sensitive liposomes with ferric ammonium (FAC) and glucose oxidase (GOx), as illustrated in Fig. 8. The outcomes demonstrated the existence of two cascade treatment techniques in FG@PEL. Because of the biological ability and response to the vascular damage brought on by photothermal action, FG@PEL, the first cascade therapy method, was capable of achieving a cascade targeting effect. In order to improve chemodynamic therapy (CDT), hydrogen peroxide was produced when glucose was oxidized utilizing GOx. FAC then catalyzed this hydrogen peroxide to accomplish a cascade reaction. Meanwhile, the photothermal effect may expedite the process and increase the amount of hydroxyl radicals produced, enhancing the therapeutic impact. Through the simultaneous modality of FG@PEL, a significant therapeutic effect could be obtained, and the principle of cooperative enhancement among various treatments was also clarified. Consequently, this study may offer a promising method for curative tumor therapy.149
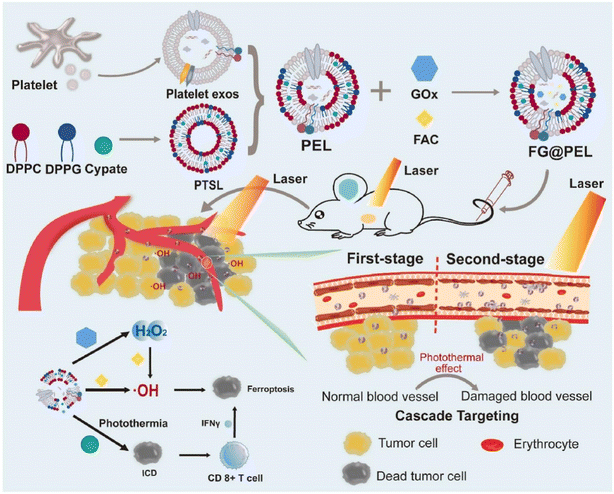 |
| Fig. 8 Schematic diagram of the platelet exosome and its application in PTT. Reproduced with permission.149 Copyright 2022, Elsevier. | |
4.1.4 Cancer cell-derived nanovesicles.
A useful technique for delivering anti-tumor therapeutics is the assembly of biomimetic nanoparticles using natural biomaterials, like cancer cell membranes, and other synthetic nanoparticles.114,153,154 Depending on the characteristics of cell membranes, the biomimetic nanoparticles demonstrated target-homing ability, sustained blood flow, and good biocompatibility.155,156 Exosomes are endogenous nanovesicles released by various cells, and have recently been developed as an innovative drug delivery mechanism.157 Additionally, many studies have shown that the membrane protein in exosomes, which have a diameter of 30–100 nm, can achieve target homing and avoid phagocytosis.57,158 Porous silicon nanoparticles (MSNs) have been frequently utilized for drug administration because of their excellent drug-loading capacity,176 in contrast to the exosomes, which have a low drug-loading ability.159,160 Further research is essential to create exosome-biomimetic nanoparticles for cancer therapy with significant drug loading and strong biocompatibility. Combining chemotherapy and photothermal therapy with exosome-biomimetic nanoparticles could result in a unique method for treating malignancies.161–164 To effectively cause tumor cell apoptosis when exposed to an 808 nm laser, ICG, one of the most widely used photosensitizers, was created.165,166 Doxorubicin (DOX), a broad-spectrum chemotherapeutic medication, and ICG administration can enhance anticancer effects.167,168 However, the clinical applications of ICG are restricted by its hydrophobic property and DOX's toxicity in vivo. In order to accomplish the anti-tumor treatment, ICG and DOX can be co-loaded into exosome-biomimetic nanoparticles, enhancing drug stability, employment, and efficacy.169 To achieve the synergistic effects of chemotherapy and photothermal therapy against breast cancer, Tian et al.'s research team created tumor-cell-derived exosome-camouflaged porous silicon nanoparticles (E-MSNs) as a drug delivery method for co-loading ICG and DOX (ID@E-MSNs). This is depicted in Fig. 9. In contrast to ID@MSNs, biomimetic nanoparticles ID@E-MSNs can be efficiently taken up by the tumor cell. The photothermal impact of ICG and the cytotoxicity of DOX are still present in ID@E-MSNs. ICG can induce hyperthermia under 808 nm near-infrared radiation to burst E-MSN nanovehicles, speed up drug release, and cause tumor eradication, resulting in efficient chemophotothermal therapy. The ability of ID@E-MSNs to accumulate in tumor tissue and limit the invasion and spread of tumours was demonstrated in vivo using 4T1 tumor-bearing BALB/c mice. The use of tumor exosome-biomimetic nanoparticles as a viable medication delivery technology for effective cancer photothermal therapy provides proof-of-concept.170
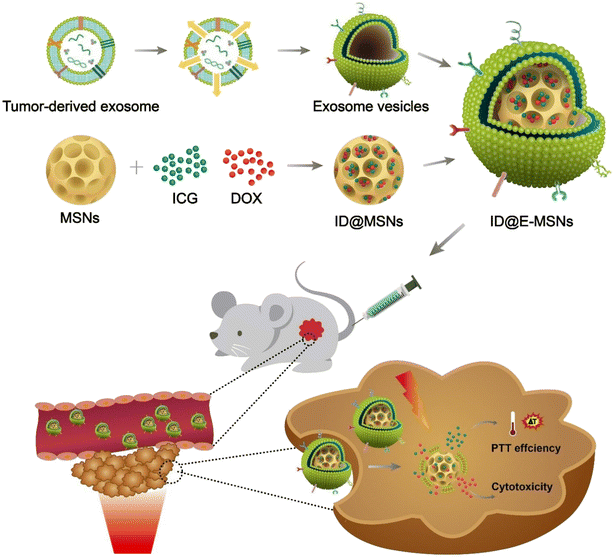 |
| Fig. 9 Diagrammatic representation of ID@E-MSNs nanosystem for targeted chemophotothermal therapy against cancer. Reproduced with permission.170 Copyright 2020, Frontiers. | |
One of the most challenging medical problems of the century is the detection and treatment of cancer. Novel anticancer methods are still desperately needed despite tremendous advancements. There has been growing interest in photothermal and sonodynamic therapy, two of the most promising methods, in which sensitizers are triggered by light or ultrasonic radiation to produce a cytotoxic impact. While these treatments have unquestionably demonstrated a high therapeutic success rate, they are inherently constrained. According to Shen et al.'s proposal as depicted in Fig. 10, a nanoplatform functionalized with black titanium nanoparticles, iridium complexes, and tumor cells membrane vesicles could be used for hierarchically targeted, synergistic photothermal and sonodynamic cancer imaging therapy. When exposed to ultrasonic waves, the particles produced heat effectively and catalytically formed reactive oxygen species. The nanoparticle formulation displayed a hierarchical targeting strategy by selectively localizing in the mitochondria and preferentially accumulating in cancerous cells. The nanoparticles were capable of acting as a treatment modality and ultimately destroying a tumor inside a mouse model when exposed to synergistic 1064 nm and ultrasonic radiation. They could also serve as an imaging element and locate the tumor site with superior spatial resolution.171
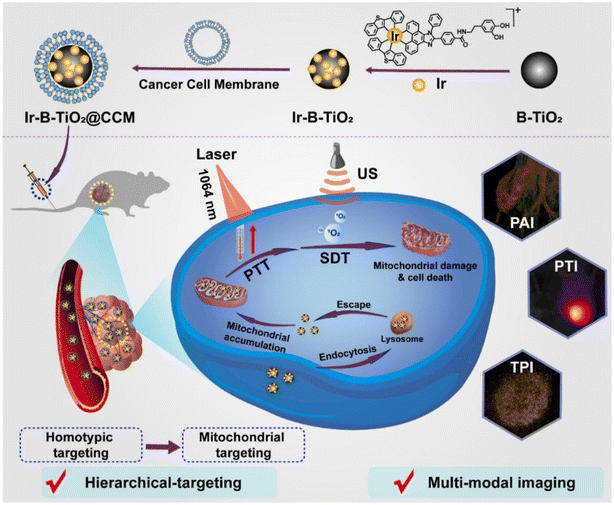 |
| Fig. 10 The preparation and mechanism of Ir-B-TiO2@CCM for multifunctional theranostic PTT and sonodynamic cancer therapy. Reproduced with permission.171 Copyright 2021, Elsevier. | |
A platform of nanoparticles coated with cancer cell membrane vesicles was revealed by Chen et al. as a theranostic nanoplatform. The biomimetic nanoparticles (ICNPs), coated with the cancer cell membrane and loaded with indocyanine green (ICG), exhibited a core–shell nanostructure composed of an ICG polymeric core and a cancer cell membrane coating. With good monodispersity, favorable photothermal responsiveness, and exceptional fluorescence/photoacoustic (FL/PA) imaging properties, ICNPs showed targeted homologous targeting to cancer cells. ICNPs dramatically increased cell endocytosis and homologous-targeting tumor accumulation in vivo by taking advantage of the functionalization of cancer cell membranes. Additionally, ICNPs were efficient at imitating cells to minimize detection by the kidneys and liver. ICNPs can achieve real-time regulated in vivo dynamic distribution with high spatial resolution and penetration using NIR-FL/PA dual-modal imaging. ICNPs demonstrated extremely effective photothermal therapy to eliminate xenografted tumors when exposed to NIR laser radiation. Potent ICNPs with characteristics similar to those of tumor cell membranes can act as a bionic nanoplatform for phototherapy and imaging of specific cancers.172 Because of its exceptional effectiveness and ability to control metastases, comprehensive anticancer therapy using integrated multimodal methods is receiving more and more attention. Using the 4T1 mouse model, Lin et al.'s research team described a synergistic triple protocol comprising immune checkpoint blockade, photothermal and sonodynamic therapy (PTT and SDT). An innovative advanced imaging nanoprobe merged with cancer cell membrane-biomimetic nanoparticles (CHINPs) preloaded with superparamagnetic iron oxide (SPIO) and hematoporphyrin monomethyl ether (HMME) enhances PTT and SDT in a synergistic manner. In situ tumor removal and systemic anti-tumor immune activation are achieved by CHINPs, which have excellent homologous tumor targeting ability and are sequentially induced by ultrasonic and NIR light with the aid of magnetic resonance, photoacoustic, and photothermal imaging. It has been demonstrated that combining this strategy with immune checkpoint inhibitor therapy inhibits tumor spread. This study offers proof-of-concept for triple therapy employing immunotherapy and multimodal imaging-guided PTT/SDT relying on biomimetic nanoprobes to eradicate malignancies.173
The accuracy and safety of cancer therapy have been enhanced by the targeted delivery of medicines employing antibodies or nanoparticles. But the scarcity and diversity of molecular targets within tumors have led to poor and inconsistent distribution of targeted medicines, impairing the effectiveness of treatment. In order to achieve this goal, Kim et al. created a collaborative targeting system in which synthetic and biological nanocomponents collaborate to localize synthetic receptor–lipid conjugates (SR–lipids) selectively to the membrane of tumor cells, amplifying the successive targeting of therapeutics. Using fusogenic liposomes, the SR–lipids were first explicitly delivered to the membranes of tumor cells in the perivascular area. The SR–lipids were distributed throughout the tumor tissues, where there were few molecular targets, by hitchhiking with extracellular vesicles released by the tumor cells. They demonstrated that the targeted photosensitizer has a uniform distribution due to the targeted delivery of SR–lipids to the cancer cell membrane.174 The effectiveness of photothermal therapy is severely constrained by the low penetration depth of photothermal agents (PTAs) active in the NIR-I biowindow and the thermal susceptibility brought on by heat shock protein (HSP). By integrating the vanadium carbide quantum dots (V2C QDs) PTA with a designed exosome (Ex) vector produced from cancer MCF-7 cells, Cao et al. proposed a technique of low-temperature nucleus-targeted PTT in the NIR-II region to effectively destroy tumors. The TAT peptides were used to modify the tiny fluorescent V2C QDs and incorporate them into Ex with RGD modification (V2CTAT@ Ex-RGD), which has good photothermal activity in the NIR-II range. The resultant nanoparticles had excellent biocompatibility, a long circulation period, the capacity to escape from endosomes, and the power to target cells and reach their nuclei to achieve low-temperature PTT with high tumor-destructive effectiveness. The NIR-II region's low-temperature nucleus-targeted PTT offers more opportunities for PTT's successful clinical application.191
The scientific world has taken notice of the promising cancer treatment method known as nanoparticles-assisted photothermal therapy. Creating bio-excretable NPs with both precise tumor-targeting and effective optical characteristics is challenging. By electroporating tumor cell-derived “exosome caps” (TT3-oCB NP@EXOs) with aggregation-induced emission (AIE) NPs, Li et al. created hybrid nanovesicles with improved PTT efficiency and second near-infrared (NIR-II, 900–1700 nm) fluorescence properties. When compared with TT3-oCB NPs, TT3-oCB NP@EXOs had superior biocompatibility, good targeting capability in vitro, the capacity to target homologous tumors in vivo, and extended circulation time. Due to their strong and consistent photothermal conversion capacity under 808 nm irradiation, TT3-oCB NP@EXOs were also used as biomimetic NPs for NIR-II fluorescence imaging-guided PTT of malignancies. As a result, the tumor cell-derived EXO/AIE NP hybrid nanovesicles could offer a potential artificial targeting technique, enhancing tumor diagnostics and PTT after assessing existing AIE NPs with poor targeting properties.175Recent research has demonstrated that PTT encourages tumor lymphocyte recruitment and the anticancer effects of CAR-T cells, indicating that PTT and immunotherapy may be an effective way to treat malignancies.176 Due to its excellent effectiveness and low invasiveness, PTT is one of the most intriguing alternatives to conventional therapy approaches for cancer.177–179 Exosomes (hEX), produced from cancer-bearing mice after PTT therapy, were successfully employed by Liu et al. as a vehicle for delivering BPQDs and cancer-specific antigens. Using C57BL/6 mice as lung cancer models, the anti-tumor capacity and anti-tumor immunological pathway of hEX@BP were examined. The outcomes demonstrated that under NIR laser exposure, hEX@BP exhibited good PTT efficiency and tumor ablation, produced local tumor necrosis due to photothermal death, stimulated host immunity, and raised the number of tumor-infiltrating T-cells. For therapeutic tumor photonanovaccines, tumor-derived exosomes may be a promising potential vector for nanomaterials and medications.180
4.1.5 Stem cell-derived nanovesicles.
As a natural delivery method, the greatly extended circulation and malignant cells-specific features of stem cells have generated intense interest for producing several biomimetic delivery systems.181,182 To increase tumor targeting and extend circulation time, it is possible to encapsulate nanoparticles using natural cell membranes to meet the objective of cell imitation.88,183 These nanoparticles acquire the overall biofunctions of actual cell membranes. The stem-cell membrane-covered nanoparticles have demonstrated significant characteristics, such as extended circulation spans and tumor targeting, in comparison with RBCM-covered nanoparticles.184 Liposomes, polymer, and peptide-conjugated systems have all been widely employed for the transport of siRNA up to the present time.185 Liposome-based carriers have been used extensively for siRNA transport because they have the benefits of strong stability and excellent transfection efficiency. However, the main difficulties for cationic liposome in vivo applications are toxicity and siRNA degradation.186 Cell membrane-derived vesicles, a natural equivalent of liposomes, have just started to be employed in medication delivery. According to research, siRNA and other drugs could be delivered through exosomes produced from plasma. Due to their great biocompatibility, nontoxicity, and direct MR imaging capacity, iron oxide (Fe3O4) NPs have undergone extensive research in medical applications.187 Polydopamine (PDA)-coated hydrophobic Fe3O4 NPs have only recently been used as a photothermal treatment modality in vivo.188,189 Additionally, PDA has a variety of surface functional groups (such as amine and catechol) that can bind a variety of biomolecules, including single-stranded DNA.190 For effective siRNA loading and transport, Mu et al.'s research team generated Fe3O4@PDA NPs. Mesenchymal stem cells (MSCs) were subsequently used to coat the Fe3O4@PDA NPs as illustrated in Fig. 11. According to the data, MSC membrane-coated Fe3O4@PDAsiRNA NPs (Fe3O4@PDAsiRNA@MSCs) retained the magnetic resonance imaging and photothermal capabilities inherited from Fe3O4@PDA. The created nanocomplexes demonstrated good siRNA delivery performance in DU145 cells. Moreover, Fe3O4@PDAsiRNA@MSCs NPs may block the activity of the endogenous Plk1 gene and result in overt death in DU145 cells by supplying siRNA against Plk1 gene. In a DU145 xenograft mouse model, a combination of photothermal therapy and gene silencing demonstrated blatant antitumor effectiveness. Fe3O4@PDAsiRNA@MSCs NPs show significant potential as a vehicle for gene and photothermal treatment based on early in vitro and in vivo research.191
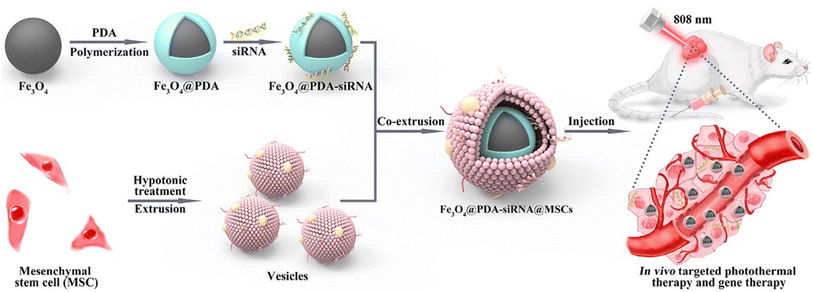 |
| Fig. 11 Illustration of the formulation of MSC membrane-cloaked Fe3O4@PDA-siRNA NPs and its in vivo targeted PTT and gene therapy. Reproduced with permission.191 Copyright 2018, American Chemical Society. | |
Due to its low invasiveness, bottom-up effect, and complete spatiotemporal control, photothermal therapy is developing as an advanced noninvasive technique for effective cancer treatment. Because of the uneven distribution of heat within the tumor, hypoxic circumstances, local relapse, and distant metastasis, most chemotherapies become less efficient when used alone.192 As a result, exosome-based chemophototherapy has been seen as a promising method for treating tumors. Li et al. thereby showed that combined therapy leads to a better chemophototherapy outcome when compared with chemotherapy or phototherapy used alone. The encapsulated medicines can circulate for a longer time in vivo and efficiently concentrate at the tumor site with the help of an exosome, as illustrated in Fig. 12. The permeability of the exosome membrane is affected by the local hyperthermia brought on by NIR radiation, which also causes drug release for sustained chemotherapeutic efficacy. More significantly, it was discovered that specific pathways, such as apoptosis, self-renewal suppression, and EMT programmed reversion, are engaged in the potential molecular processes of exosome-based synergistic chemophototherapy. According to these findings, the modified exosome can successfully integrate chemotherapy and phototherapy to cure glioma. It may also offer a novel therapeutic approach and possible molecular target for clinical treatment.193
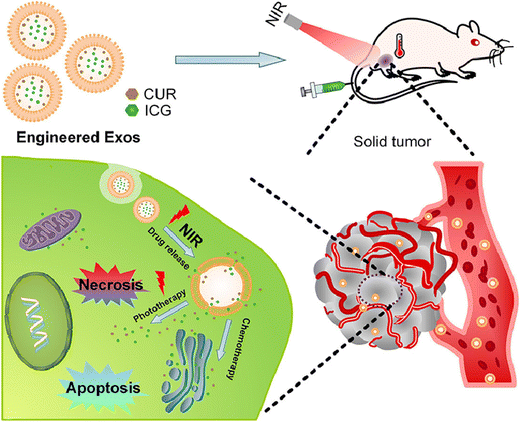 |
| Fig. 12 Diagram of engineered exosome for NIR-triggered drug delivery and robust synergistic chemophototherapy against glioma model. Reproduced with permission.193 Copyright 2020, Elsevier. | |
4.1.6 Hybrid membrane-derived nanovesicles.
To date, various nanovesicles with hybrid biomimetic membranes have been created and engineered. These nanovesicles serve purposes like delivering drugs, detoxifying, detecting cancer, and producing cancer vaccines.194 Hybrid nanovesicles typically utilize membranes sourced from various cells like RBCs, platelets, cancer cells, immune cells such as macrophages, and even bacteria.195,196 Nanovesicles using different cell membranes can be compared with those with only one membrane type. They can do the same things as the original membranes, like making the nanovesicles last longer, getting past the immune system, directly targeting tumors, and sticking to tumor cells.197,198 Furthermore, these mixed-membrane nanovesicles can also perform new functions that the regular membranes cannot do. For instance, they can show tumor signals to immune system cells (antigen-presenting cells) by finding their way to lymph nodes.195 Moreover, nanovesicles with hybrid membranes have the potential to enhance how nanoparticles spread in tissues by lessening the attachment of unrelated normal cells (like WBCs).199 Alternatively, they can specifically enhance the gathering of nanoparticles in organs like the liver and spleen.200 Because of these clear benefits, using a mix of cell membranes in hybrid membrane nanovesicles has demonstrated significant potential for cancer PTT applications.
Wang et al. discussed a hybrid membrane called OMV-CC, which combines a bacterial outer membrane vesicle (OMV) with a B16-F10 cancer cell (CC) membrane. They successfully coated this hybrid membrane onto hollow polydopamine (HPDA) nanoparticles. By merging OMV immunotherapy with HPDA-based photothermal therapy (PTT), they enhanced the effectiveness against melanoma. Injecting HPDA@[OMV-CC] nanoparticles into the tail vein led to even distribution within melanoma and triggered an immune response. This response rapidly matured dendritic cells (DC) in the lymph nodes of treated mice. The results suggest that combining anti-tumor immune response and PTT reinforces treatment, effectively eliminating melanoma without notable side effects.201 Bu et al. developed a new approach by creating a hybrid membrane-coated iron oxide magnetic nanoparticle (MN) called {[CSC-P]MN}. This method was used for the first time to improve photothermal therapy (PTT) for head and neck squamous cell carcinoma (HNSCC). The platelet membrane inherited from its source cells can avoid the immune response because of its surface markers with “don't eat me” signals.
On the other hand, the CSC membrane can target the cancer cells due to specific surface molecules. The [CSC-P]MNs possess great qualities for immune evasion, accurate cancer targeting, magnetic resonance imaging, and photothermal therapy. Compared with MNs coated with a single cell membrane, [CSC-P]MNs stay in circulation longer and target better. Moreover, [CSC-P]MNs have a stronger PTT ability, significantly inhibiting HNSCC tumor growth. Overall, this biomimetic multimembrane-coated nanoplatform has the potential to enhance the effectiveness of therapy against tumors in the complicated tumor environment.202 Researchers have extensively investigated polypyrrole nanoparticles (PPy NPs) for their ability to generate heat when exposed to NIR light, which makes them promising for PTT of tumors. However, creating safe, self-targeting, and long-lasting PPy materials to maximize PTT effects is still challenging. Liu et al. demonstrated that these nanoparticles could effectively destroy tumor cells under direct NIR light exposure by covering PPy NPs with a combination of RBCM and platelet membranes. These newly developed PPy@[R–P] nanoparticles combine the features of both RBCM and platelets, resulting in extended circulation times and the ability to target themselves to the tumor site. When injected through the tail vein, these nanoparticles caused photothermal stimulation under NIR laser exposure, damaging tumor blood vessels and causing microthrombosis. With the presence of platelet membranes, a significant amount of PPy@[R–P] nanoparticles accumulated at the sites of microthrombosis. This improved the distribution of nanoparticles in tumor tissues and led to successful photothermal treatment. The outcomes from using PPy@[R–P] nanoparticles hold promise for effective anti-tumor photothermal therapy.203 Jiang et al. combined RBCM with membranes from MCF-7 cells to create a hybrid membrane, forming an erythrocyte-cancer (RBC-M) hybrid membrane. This hybrid membrane camouflaged melanin nanoparticles, resulting in a platform named Melanin@RBC-M. The purpose was to improve the effectiveness of photothermal therapy (PTT). The combined RBC-M hybrid membrane vesicles retained the proteins from RBCM and MCF-7 cell membranes. The resulting Melanin@RBC-M platform showed extended circulation in the bloodstream and could target MCF-7 cells specifically. Interestingly, when the MCF-7 cell membrane components in RBC-M were increased, the homotypic targeting ability of Melanin@RBC-M also increased. On the other hand, increasing the RBCM components in RBC-M decreased the uptake of Melanin@RBC-M by macrophages and extended their circulation time in the bloodstream. When injected into mice with MCF-7 tumors, Melanin@RBC-M nanoparticles containing a 1
:
1 ratio of RBC and MCF-7 membrane proteins showed notably higher accumulation in tumors and better PTT effects. This outperformed other Melanin@RBC-M nanoparticles with different protein ratios and regular melanin nanoparticles. This success was due to finding the right balance between prolonged circulation in the bloodstream and targeted tumor binding. Applying hybrid membranes to nanoparticles could provide flexibility and control in enhancing their functions, opening up new possibilities for medical use.204
Hepatocellular carcinoma (HCC) ranks as the third major cause of cancer-related deaths across the globe. Nanoparticles coated with cell membranes, mimicking biological structures, exhibit better compatibility and specificity for similar cells. They have gained significant attention for targeted therapies against tumors. In their study, Ji and his team created a hybrid membrane-coated nanoparticle by combining cancer cell and macrophage membranes. These nanoparticles, known as CuS-SF@CMV NPs, were responsive to near-infrared light, enclosing sorafenib and modified with anti-VEGFR. These CuS-SF@CMV NPs featured distinctive membrane proteins from both cancer cells and macrophages. They could selectively accumulate in cancer cells in lab tests and tumors in live subjects, as opposed to standard CuS NPs. Furthermore, when exposed to near-infrared light, the CuS-SF@CMV nanoparticles accomplished combined photothermal and chemotherapy effects in cancer cells. This led to a 94.3% reduction in tumor growth in a mouse hepatoma model. The initial temperature rise rapidly eliminated tumor cells, while the presence of sorafenib and the anti-VEGFR antibody extended the tumor-killing action. Sorafenib hindered tumor cell growth, and the anti-VEGFR antibody obstructed angiogenesis through specific pathways. Overall, the CuS-SF@CMV nanoparticles were able to evade the immune system, target tumor cells, load drugs, and convert light into heat. This combination makes them highly suitable for synergistic photothermal and chemotherapy treatment against hepatocellular carcinoma.205
4.2 Photodynamic therapy (PDT)
It is unknown how exosomes function in PDT. Our considerable effort has recently been focused on learning more about the function of cell membrane-derived nanovesicles in photodynamic cancer therapy. The role of nanovesicles in photodynamic therapy for cancer treatment will be the main topic of discussion in this section, as summarized in Table 2.
4.2.1 RBCs-derived nanovesicles.
In addition to naturally occurring carriers like RBCs, the human body can also be endowed with synthetic and human-made nanocarriers. RBCs are biconcave-shaped adaptable cells can move through the capillary network smaller than their diameters.219 A significant portion of RBCs in the body transport oxygen and carbon dioxide and act as a buffer system for maintaining the body's pH. To carry therapeutic payloads, scientists have taken advantage of RBCs’ key characteristics, such as nonimmunogenicity, biocompatibility, longer circulation time, and higher volume.220 By culturing them with the whole or lyzed RBCs, cargo or biomolecules can be loaded.221 These RBCs can then be utilized for controlled delivery of the cargo in blood after being encapsulated.222 The RBCM can be employed to create nanosized vesicles for the delivery of cancer drugs in addition to serving as a carrier for entire RBCs.223 Unlike chemotherapy and radiation therapy, photodynamic therapy offers several advantages, such as noninvasiveness, lack of systemic toxicity, and a lower risk of developing drug resistance. To treat bladder, cervical, and lung cancer, photodynamic medicines such as aminolevulinic acid (ALA), protoporphyrin, and hematoporphyrin derivatives have been authorized.224 Recent research has demonstrated that combination therapies are more efficient in treating cancer than either chemotherapy or photodynamic treatment alone.225 In order to achieve the desired therapeutic outcome, nanocarriers should also remain in the blood for a longer time. The prolonged circulation of the nanocarrier can be achieved by coating its surface with appropriate materials, thereby avoiding clearance by the reticuloendothelial system (RES).224 Although RBCs are biocompatible and employed for prolonged drug release, tumor-specific drug delivery may not be possible without altering the RBC surface. The RBCM can now be chemically altered to target tumors. In order to produce chemotherapeutic and photodynamic effects, Bidkar et al. showed the preparation of transferrin (TF) receptor-targeted RBCM-coated NPs. To create the TF-DoxMB NPs depicted in Fig. 13, the TF-conjugated RBCM were designed to include doxorubicin (Dox) and methylene blue (MB)-loaded PLGA NPs. The NPs were 106 nm in size. The TF-conjugated membrane was coated on the NP surface, which allowed the medications to be delivered specifically to cancer cells that express the TF receptor. Dox and MB were explicitly delivered to the cancer cells, followed by laser irradiation to eradicate them. The combination of chemo- and photodynamic treatment exhibited synergistic activity, according to viability assays and combination index (CI) studies. Interestingly, Dox's antiproliferative action and the ROS that photosensitization created caused DNA damage-mediated death in HeLa and MCF-7 cells. Because of the synergistic activity of Dox and MB, the targeting effectiveness of the TF-DoxMB NPs was extremely remarkable in tumor spheroids.226
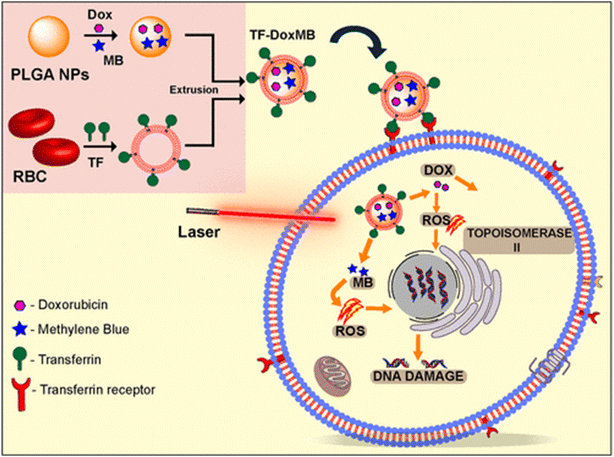 |
| Fig. 13 Presentation of transferring-conjugated RBCM-coated PLGA NPs preparation for chemo- and PDT against cancer. Reproduced with permission.226 Copyright 2020, American Chemical Society. | |
Biocompatible exosomes are readily available and could be less immunogenic than artificial delivery methods.227 Due to their ideal size, exosomes can diffuse passively into tumors and bypass the mononuclear phagocyte system by taking advantage of the increased permeability and retention (EPR) effect.228 Additionally, exosomes containing phospholipid bilayers could merge with the plasma membrane directly to improve cellular absorption.229 Besides that, the ineffective drug entrapment efficiency and challenging multifunctional modification processes severely limit the biological uses of exosome-based delivery systems.137 Additionally, delivering the medicine in exosomes to its therapeutically active region is extremely difficult and crucial in achieving the intended efficacy. Most nanodrug delivery systems are required to pass through several physiological obstacles before reaching their specialized subcellular targets.230,231 According to some reports, a photodynamic treatment that targets the plasma membrane can bypass intracellular barriers to damage the membrane's integrity and kill cells.232–234 While this happens, the plasma membrane rupture may alter its permeability, leading to cell photochemical internalization (PCI) and increased photosensitizer uptake.235 However, the photosensitizer-generated lethal ROS have a short half-life (40 ns) and a small diffusion range (20 nm), making them simple to deactivate during cytoplasmic diffusion.236 The single membrane-targeted PDT impact is frequently inadequate. Because the DNA helix is the focus molecule of ROS, nucleus-targeted PDT is seen as an excellent alternative as a supplementary therapy for tumors.237 Notably, the efficacy of the photosensitizer's nuclear penetration could be improved by conjugating it to a nuclear localization signals (NLS) peptide, but it is severely constrained by endo/lysosomal trapping and enzymatic degradation.238 Exosomes can directly fuse with plasma membranes to enter cells, although there is still a non-negligible amount of endocytosis-induced endo/lysosomal trafficking. To enhance the cytosolic and nuclear distribution of photosensitizer in exosome-based nanosystems, a strong strategy is urgently required.239 Cheng et al. created exosomes using chimeric peptides to target photosensitizer distribution to the plasma membrane and nucleus and simultaneous photodynamic therapy. Notably, the plasma membrane and nucleus of tumor cells are preferentially and progressively destroyed in precision PDT using a dual-stage light method. In a nutshell, plasma membrane-targeted PDT of chimeric peptide-modified exosomes (ChiP-Exo) could directly impair the structure of the membrane and partially induce cell death. More intriguingly, the first-stage light's photochemical internalization and lysosomal escape dramatically increase the cytosolic distribution of ChiP-Exo, which may boost its nuclear transport because the peptide contains nuclear localization signals (NLS). Intranuclear ChiP-Exo would generate ROS in situ to destabilize nuclei for strong and synergistic PDT upon the application of second-stage light irradiation. This double-light directed subcellular dual-targeted PDT strategy, which is based on exosomes, exhibits a significantly improved therapeutic benefit on the inhibition of tumor growth with minimal system toxicity, and it also offers novel insights for the development of individualized biomedicine for accurate tumor therapy.240
The biomimetic approach has presented many prospects for building therapeutic systems with improved anticancer activity and biocompatibility. RBCM-camouflaged nanoparticles (RBCs (M(TPC-PTX))) have been described by Pei et al. for use in combination with photodynamic treatment. According to Fig. 14, the 5,10,15,20-tetraphenylchlorin (TPC) photosensitizer and the ROS-responsive PTX dimer (PTX2-TK) make up the majority of the inner core's construction. The produced RBCs(M(TPC-PTX)) is quickly taken up by endosomes according to in vitro tests. The TPC can produce ROS under the right lighting conditions, activating PTX2-TK cleavage and on-demand PTX discharge for chemotherapy in addition to PDT. According to in vivo research, RBCM coating increases accumulation in tumor and prolongs blood circulation. Combining chemotherapy with photodynamic therapy improves the therapeutic effect against cancer, and light-triggered medication release lowers systemic toxicity. These qualities make the proposed technology for treating cancer very promising.241
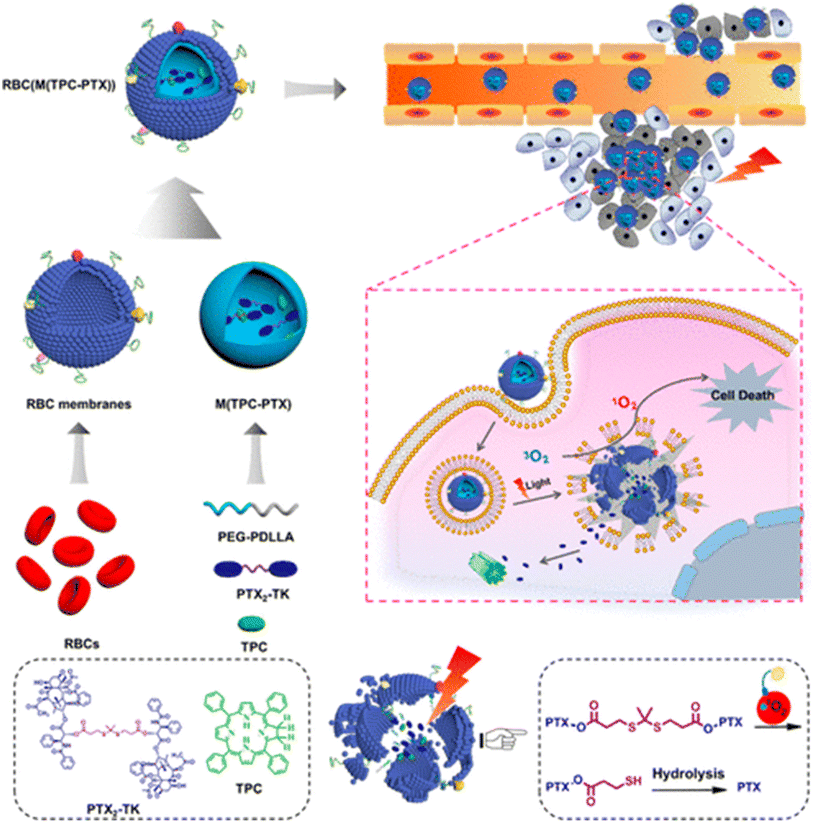 |
| Fig. 14 Representation of RBCM-cloaked dimeric prodrug NPs (RBCs(M(TPC-PTX))) in combination chemo/PDT for extended blood circulation and light-mediated drug release. Reproduced with permission.241 Copyright 2018, American Chemical Society. | |
4.2.2 Cancer cell-derived nanovesicles.
In photodynamic therapy, harmful free radicals and ROS, including singlet oxygen, are produced when photosensitizer (PS) chemicals are exposed to specific light wavelengths in the presence of oxygen (O2). PDT is a useful treatment for a variety of illnesses, including superficial or localized malignancies.242–244 The adoption of novel PDT techniques has been made possible by introducing PS substances with aggregation-induced emission (AIE) features. When AIE-based fluorophores are present in solution as aggregate molecules, they emit substantially more powerful emissions.245,246 Due to their strong photostability, biocompatibility, and capacity for high-contrast imaging, AIE luminogens (also known as AIEgens) make excellent agents for bioimaging purposes.247 AIEgens can be used for PDT-based treatment modalities because several of them easily produce ROS.248 Since the bulk of AIE PS substances are extremely hydrophobic, they are consequently difficult to use in in vivo biological situations. The direct intratumoral administration of AIE PS substances following their entrapment within the hydrophobic core of novel AIEgen-based hybrid systems, such as lipid nanoparticles, may be attained. However, these AIEgen-based methods are constrained by their poor target tissue permeability, low encapsulation efficiency, limited capacity to target tumors precisely, and poor drug loading content (DLC).249,250 As a result, new AIEgen-based platforms must be created to penetrate tumors more successfully. Exosomes produced by tumors may be the most effective way to increase AIE PS tumor invasion. Exosomes are microscopic (50–200 nm) cellular secretions originating from multivesicular structures.251 With the aid of commercially available reagents, such exosomes may be easily extracted from biological samples and the supernatants of cell cultures.252 Due to their endogenous origin, exosomes have a number of characteristics that render them potential options for drug delivery methods, including a lack of immunogenicity, great biocompatibility, and the capability to stay in the bloodstream for long periods of time.253 These exosomes can also traverse the blood–brain barrier and penetrate deep into different tissues that are thick or structural.254,255 It is significant to note that exosomes can be swallowed by cells and can adhere to particular tissues or cell types in a particular way, depending on the protein profiles of the exosome membrane and cell surface.256 Exosomes are perfect for clinical applications in drug delivery because they improve the solubility of substances typically insoluble in aqueous solutions.257 Thus, the efficient production of exosome/AIEgen hybrid nanovesicles could enhance the efficacy of PDT for cancer therapy. To create tumor exocytosed exosome/AIE luminogen (AIEgen) hybrid nanovesicles (DES) that might allow effective tumor penetration, Zhu et al. used an electroporation approach. In order to alleviate local hypoxia and restore normal vascular function inside the tumor microenvironment (TME), dexamethasone was used. This dramatically improved the PDT effectiveness of DES nanovesicles and allowed them to successfully limit tumor growth. For the first time, hybridization of AIEgen and biological tumor-exocytosed exosomes was accomplished, and it was coupled with PDT methods by restoring intratumoral vascular regularity to lessen local tissue hypoxia. This study emphasizes a novel method for developing PDT systems based on AIEgens and the potential clinical utility of AIEgens.258
A possible strategy to increase the effectiveness of cancer treatment is to specifically shut off the nutrition supply and metabolism pathways of cancer cells. By embedding glucose oxidase (GOx) and catalase in the porphyrin metal–organic framework (MOF) of PCN-224 (PCN stands for porous coordination network), Li et al. research team created a cancer-targeted cascade bioreactor (designated as mCGP) for synergistic starving and PDT. It is shown in Fig. 15. The immune evasion and homotypic targeting characteristics of mCGP would significantly improve its cancer targeting and retention capacities as a result of biomimetic surface functionalization. By catalyzing endogenous hydrogen peroxide (H2O2) to produce oxygen (O2) after being ingested by cancerous cells, mCGP has been discovered to enhance microenvironmental oxygenation. This would then hasten the breakdown of intracellular glucose and boost the output of cytotoxic singlet oxygen (1O2) when exposed to light. Because of this, mCGP demonstrated enhanced combinatorial therapeutic benefits of long-term cancer deprivation therapy and powerful PDT, which effectively inhibited the tumor growth after just one injection. The development of supplementary approaches for spatiotemporally regulated cancer treatment might be easily achieved by this cascade bioreactor.231
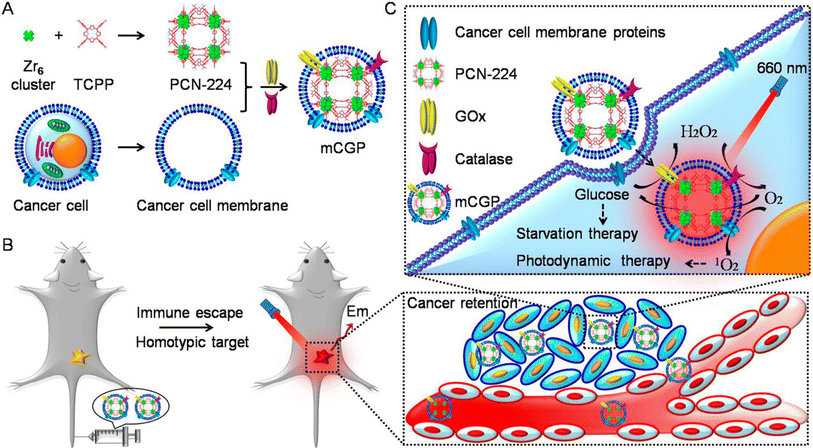 |
| Fig. 15 Figure showing cancer cell membrane-cloaked cascade bioreactor for targeted cancer starvation and PDT. (A) Preparation method of mCGP. (B) Homotypic targeting and immune escape mechanism after iv administration of mCGP. (C) Starvation therapy via cut of glucose supply cascade reaction and 1O2 generation for PDT. Reproduced with permission.231 Copyright 2017, American Chemical Society. | |
An innovative approach for successful cancer treatment would involve altering the tumor microenvironment. By fabricating TPZ-loaded porphyrinic metal–organic framework PCN-224, a cancer cell membrane-coated nanoplatform (TPZ@PCN@Mem), with tumor-targeted PDT and the ensuing hypoxia-amplified bioreductive therapy, Zhang et al. have made significant advances in the field of medicine. Due to the immune escape and homologous targeting capabilities provided by the cancer membrane coating, TPZ@PCN@Mem showed preferential buildup and long-term survival at tumor tissue after treatment. The stimulation of TPZ for improved chemotherapy in 4T1 orthotopic tumor was further accelerated by the local hypoxic microenvironment that resulted from the production of harmful ROS by PCN-224 during light irradiation. With few adverse effects, the simultaneous cascade therapeutic benefits of TPZ@PCN@Mem could dramatically slow the primary tumor's growth and block its distant metastasis. The discovery gave a new understanding of focused and efficient tumor treatment by demonstrating an overwhelming advantage of using this bioinspired technique for hypoxia-activated bioreductive treatment and tumor-targeted PDT.230
Exosomes, produced by all cells and involved in cell-to-cell interaction, have been used in numerous recent studies as DDS. A developing medical technique called immunotherapy uses the patients’ immune systems. Immune cells are triggered in immunotherapy by antibodies, other immune cells, and genetic alterations for the objectives of cancer therapy. Jang et al. used tumor-derived reassembled exosomes (R-Exo) as both a medication delivery vehicle and an immunostimulatory agent. A chlorin e6 photosensitizer was added to exosomes produced from tumors during exosomal re-assembly. Following this alteration, R-Exo generally contains the same membrane proteins and their original average size, enabling the targeting of tumor cells. As seen in Fig. 16, chlorin e6-loaded R-Exo (Ce6-R-Exo) can effectively produce ROS inside tumor cells when exposed to laser irradiation. Ce6-RExo also promoted immune cell cytokine production, suggesting that these transformed exosomes have potential as an immunotherapeutic drug. This innovative method uses tumor-derived Ce6-R-Exo to deliver immune-combination therapy and photodynamic therapy for cancer treatment.259
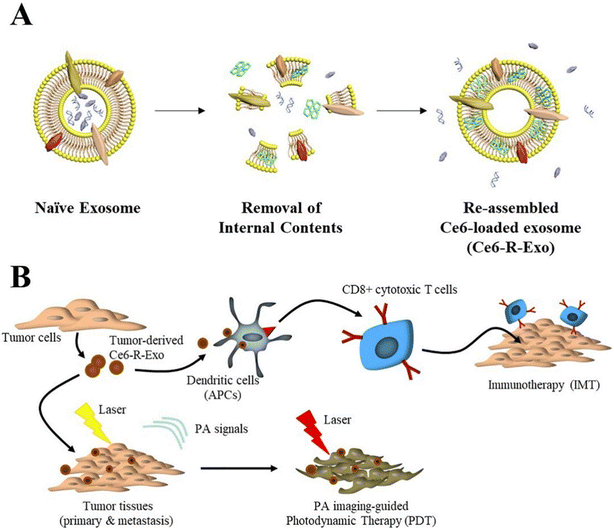 |
| Fig. 16 Illustration of Ce6-R-exosome. (A) Preparation method of Ce6-R-exosome (B) Demonstration of a combination of PDT and immunotherapy with PA imaging guidance. Reproduced with permission.259 Copyright 2021, Elsevier. | |
A new cancer treatment named photodynamic therapy is especially well-suited for treating confined malignant tumors. As a chemotherapeutic agent, the phototherapeutic drug is typically injected into the bloodstream and travels throughout the body. However, to provide localized therapeutic effects, light stimulation is required. EVs are produced in large quantities by cancer cells in vitro and in vivo in response to photodynamic therapy. Aubertin et al. discovered that following an hour of photoactivation, millions of EVs per cell were released into the extracellular media. Doxorubicin treatment (chemotherapy) had a comparable impact, although fewer EVs were generated 24 h after the therapy. They also discovered that the produced EVs were capable of transferring big intracellular items, medicines, and even extracellular membrane parts to naive target cells. The massive stimulation of cancer cell vesiculation by anti-cancer therapies was confirmed in vivo by the many fold increase in circulating EV levels caused by photodynamic therapy and chemotherapy.260
4.2.3 Stem cell-derived nanovesicles.
The great extended circulation and tumor-specific features of stem cells as a natural carrier system have sparked intense interest in producing several biomimetic delivery systems. To increase tumor targeting and extend blood circulation time, it is possible to encapsulate nanoparticles with natural cellular membrane to fulfill the cell mimicry criterion.136,183 These nanoparticles that mimic cell membranes inherit the collective biofunctions of natural cell membranes. Stem-cell membrane-covered nanoparticles have demonstrated significant characteristics, such as prolonged circulation durations and tumor targeting, compared with RBCM-covered nanoparticles. The photodynamic therapeutic agents based on upconversion nanoparticles (UCNPs) hold promise for the treatment of deep-tissue cancer because they may be able to get over the present restrictions brought on by the shallow depth of penetration of visible light. However, the therapeutic effects of UCNP-based PDT in vivo are hampered by short blood circulation times and inadequate tumor targeting. Gao et al. revealed intravenously injectable stem-cell-membrane-camouflaged upconversion nanoarchitecture as a biomimetic tumor PDT platform for this reason. As seen in Fig. 17, the biomimetic PDT system is created by fusing mesoporous silicon-encapsulated -NaYF4:Yb3+, Er3+ UCNPs with stem-cell membranes. Multiple membrane components were translated as a consequence of the translocation of the stem-cell membranes to the UCNPs, giving the platform the long circulation and tumor-targeting capabilities of the membranes. A single 980 nm laser was used concurrently, activating multiple photosensitizers due to the UCNP cores’ capacity to emit various colors. Experiments conducted in the lab and on animals showed that this unique platform inherited stem cells’ capacity to target tumors and demonstrated significant accumulation. The new photosensitizer-loaded system has greater tumor suppression effectiveness by iv tail injection, according to in vivo tumor PDT studies. This stem-cell-membrane-camouflaged upconversion nanoarchitecture equips synthetic UCNPs with biological cell membranes and shows potential for systemic administration of deep-tissue PDT cancer therapy.261
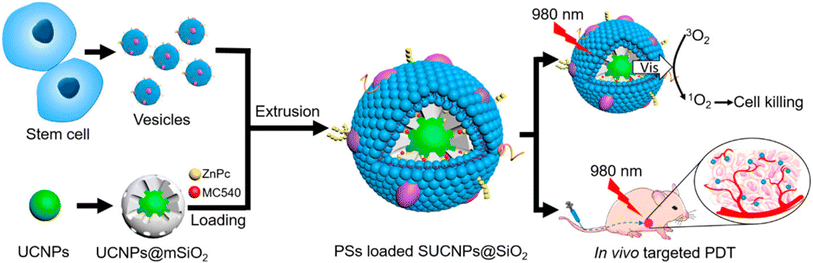 |
| Fig. 17 The preparation process of SUCNPs@mSiO2 and its PDT mechanism. Reproduced with permission.261 Copyright 2016, American Chemical Society. | |
ROS are produced during photodynamic therapy employing photosensitizers, which cause tumor cells to undergo apoptosis or necrosis. However, the photosensitizer's ineffective targeting, poor water solubility, and quick removal from the blood remain significant obstacles to its good anticancer activity. A stem cell membrane-camouflaged gelatin nanogel (NG) was created by the research team of Feng et al. It combines the stem cell membrane's targeting ability with Ng's drug-loading capacity, providing a variety of unique benefits for directed drug administration. This bioinspired DDS consists of wrapped stem cell membrane vesicles (SCV), the outer shells, and hydrophobic photosensitizer-loaded gelatin nanogels (Ng) (Ng/Ce6), the inner cores. Ng/Ce6@SCV had an average hydrodynamic diameter of 202.7 nm, with a polydispersity index (PDI) of 0.113. After receiving near-infrared (NIR) laser irradiation, Ng/Ce6@SCV could effectively encourage the cellular internalization of Ce6 and produce sufficient ROS in the tumor cells to effectively inhibit the growth of A549 tumor cells in vitro. Following delivery, Ng/Ce6@SCV displayed targeting buildup and long-term persistence at tumor tissues, which was associated with the stem cell membrane's capacity for immune evasion and tumor targeting. The in vivo anti-tumor performance results showed that, following NIR radiation, Ng/Ce6@SCV had an increased anti-tumor effect by considerably reducing primary tumor development with few adverse effects. All findings suggested that this polyphosphoester-based, bioinspired nanodrug delivery system would work well for accurate and efficient PDT of malignancies.262
Although cytoreductive surgery is successful, the spread of tumor metastasis in the peritoneal cavity, also known as peritoneal metastasis (PM) or carcinomatosis, marks an advanced stage of gastrointestinal and gynecological cancer with a very bad prognosis due to microscopic residual disease. Due to the low tumor specificity of photosensitizers and significant side effects, PDT has been therapeutically limited in managing peritoneal metastases. EVs obtained from mesenchymal stem/stromal cells (MSCs) have been proposed by Gazeau et al. as the 4th generation of immune-active PS vectors capable of targeting peritoneal metastasis with outstanding selectivity, potentiating PDT cytotoxicity at the tumor site without affecting healthy tissues. A ground-breaking method for the high yield, mass manufacturing of MSC-EVs with the therapeutic meta (tetra hydroxyphenyl)-chlorin (EVs-mTHPC) suitable for clinical translation needs has been created. Compared with the free medication and the liposomal formulation Foslip, intraperitoneal injection of EVs-mTHPC demonstrated a substantial improvement in tumoral selectivity. A significant amount of tumoral necrosis was enabled by PDT mediated by EVs-mTHPC (55% of necrotic tumoral nodules versus 18% for Foslip, p 0.0001), and antitumor immune cell infiltration, primarily proinflammatory M1-like CD80+ and CD8+ T cell effector, was also encouraged. Following PDT using EVs-mTHPC, intra-tumor growth was dramatically reduced. Overall, the tumoral selectivity that was made possible by EV vectorization of mTHPC allowed it to circumvent the PDT toxicity of the free medication and lengthen mouse lifespan in the colorectal carcinomatosis model. The therapeutically applicable fourth-generation PDT platform for peritoneal metastasis treatment that uses MSC-EVs appears to overcome current PDT limitations and foster a hot tumor immune milieu in PM.263
4.2.4 Platelet cell-derived nanovesicles.
The significant difficulties that make tumor photodynamic therapy less effective as treatment include the following. ROS (PDT's cytotoxic factor) have a minimal lifetime, and additional study is required for functionalizing photodynamic nanostructures with active targeting moieties. Deep tissue invasion requires the creation of photodynamic systems that are excited by longer near-infrared light because the existing ones are typically activated by light at the short end of the NIR band. The effect is compromised when the irradiation dose is reduced, which is important to prevent skin injury. Finding a solution to this problem is still difficult. For this reason, other research group instead of demonstrated that platelet membrane-coating over a nanoparticle preloaded with a photodynamic sensitizer combined with exposure to solar light might help to address all of the aforementioned difficulties. As seen in Fig. 18, the platelet membrane-coating gives the resultant nanoparticle extended systemic circulation and active tumor cell-targeting.264,265 When the platelet membrane is fused over a synthetic nanoparticle, the resulting particle inherits the platelet's active targeting ability, which results in markedly elevated deposition within the tumor.136,152,266,267 P-selectin, a membrane protein found naturally on the platelet surface, modulates its active targeting potential by primarily binding with CD44 receptors up-regulated on the cancer cell surface. It was also demonstrated that preloading verteporfin into PLGA nanoparticle (NP-Ver) changes its absorption peak from 682 nm to 712 nm, which favors deep tissue penetration.268 Verteporfin is a photodynamic agent approved by the FDA for eradicating abnormal blood vessels in eyes.269,270 Despite having a similar capacity for increasing a nanoparticle's systemic circulation, the platelet membrane-coated nanoparticle (NP-Ver@P) exhibits even better tumor absorption when injected iv than its RBCM-coated counterpart (NP-Ver@R).271 NP-Ver@P outperforms NP-Ver@R when exposed to sunlight in the 680 nm–730 nm range at an output energy density of just 0.05 W cm−2, enabling useful therapeutic results without harming skin tissue at the tumor location.272
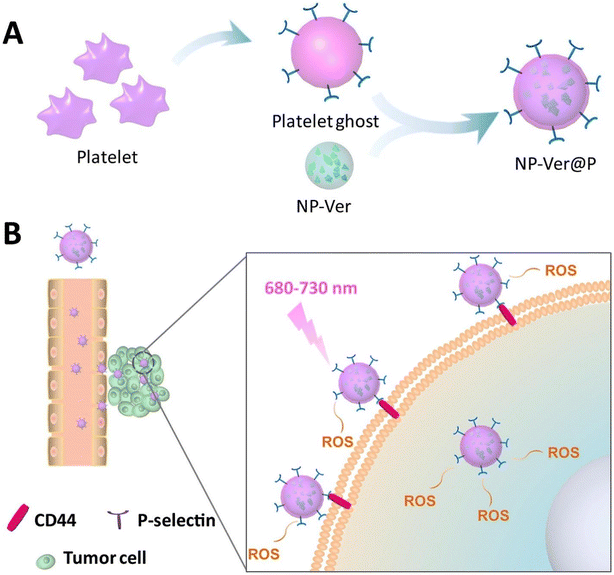 |
| Fig. 18 Schematic representation showing (A) Fabrication of NP-Ver@P by fusing platelet ghost-derived vesicles over verteporfin PLGA NPs and (B) Active targeting mechanism through specific binding of P-selectin with CD44 receptor on the cancer cell and ROS generation via NIR irradiation. Reproduced with permission.272 Copyright 2018, Elsevier. | |
W18O49-mediated photodynamic treatment and photothermal therapy are constrained by tumor hypoxia and readily oxidized characteristics. To co-load W18O49 NPs with metformin, Zou et al. reported the production of platelet membranes as nanocarriers (PM- W18O49-Met NPs). Through the passive EPR effect and active adherence between platelets and cancer cells, platelet membranes can promote the buildup of W18O49 at tumor areas and shield it from oxidation and immunological escape. Metformin (Met), a common anti-diabetic medication, can be used to treat tumor hypoxia by lowering oxygen uptake. Consequently, ROS/hypoxia imaging in vitro, IR thermal imaging in vivo, and PET imaging in vivo, ROS and heat production are significantly increased. Improved therapeutic benefits are demonstrated by PM-W18O49-Met NPs, which also significantly limit tumor development and promote tumor cell apoptosis. As a result, our work provides a unique approach for concurrently increased PDT and PTT, which has promise for use in bio applications.
4.2.5 Hybrid membrane-derived nanovesicles.
Mixing various cell membranes with unique proteins and traits can empower biomimetic nanosystems to carry out diverse functions within changing biological conditions.273,274 When we look at nanovesicles made from mixed membranes, they can show better performance and safety compared with those derived from a single cell membrane.
This section highlights the recent uses of nanovesicles derived from hybrid membranes for breast cancer PDT. Breast cancer is common in women and often has poor outcomes. Xie with his colleagues combined a plant-based component called chikusetsusaponin IVa methyl ester (CSME) from traditional Chinese medicine, Panax japonicus, with a photodynamic agent called chlorin e6 (Ce6). They improved the therapeutic effects against breast cancer by using liposome nanoparticles coated with a mix of cell membranes (red blood cell and cancer cell membranes). The liposomes were also modified with RGD for better solubility, targeting, and treatment results with CSME. These findings show the successful development of nanocomplexes that last longer in the body, avoid the immune response, target the tumor site, and effectively fight cancer without harming normal tissue. The nanocomplexes work by controlling cell growth and causing cell death. Overall, this liposome-based drug-delivery approach offers a promising option for treating breast cancer with chemotherapy and photodynamic therapy.275
Triple-negative breast cancer (TNBC) is a very aggressive form of breast cancer that lacks effective treatment targets. PTT and PDT are two important ways to treat TNBC, and when combined with chemotherapy, they can work even better together. Researchers from the Zhang group developed a special nanoplatform using a mix of leukocyte and platelet cell membranes (LPHM) and large-pore mesoporous silicon nanoparticles (DLMSNs). They loaded a near-infrared fluorescent dye called IR780 and a chemotherapy drug called doxorubicin (DOX) into the big pores of DLMSNs, making DLMSN@DOX/IR780 (DDI) nanoparticles. These nanoparticles were then covered with LPHM to create LPHM@DDI nanoparticles. With the help of LPHM, LPHM@DDI nanoparticles effectively targeted TNBC cells and showed strong performance in PTT and PDT, both in lab tests and live subjects. When exposed to near-infrared laser light, LPHM@DDI nanoparticles showed a combined effect that both harmed TNBC cells and prompted them to undergo apoptosis. In TNBC mice, these nanoparticles effectively stopped tumor growth and prevented its return by destroying the tumor and stopping blood vessel growth (anti-angiogenesis). This positive outcome came from the synergy of combining PTT, PDT, and chemotherapy. Overall, this research presents a hopeful nanoplatform that can efficiently carry and deliver both photo and chemotherapy drugs to target TNBC cells, offering a potential treatment approach for TNBC.276
5. Challenges
Using biomimetic nanovesicles as delivery systems for cancer phototherapy has several benefits but also some drawbacks. The main drawback is understanding cell membrane-derived nanovesicles’ function and structure. Inadequate nanovesicle separation and isolation methods, leading to a low yield, are the first significant disadvantages associated with their pharmaceutical use.281 Through the transfer of biomacromolecules from the host to the receiving cell, these nanovesicles play a role in cellular communication. The specific molecules conveyed, their functions and the resulting heterogeneity are all unknown.282 To avoid inducing an immunological reaction, it is also important to select appropriate donor cells.283 Even if the donor cells are recognized, a further approaching issue is a scale-up procedure to create enough vesicles for effective therapy.284 Nanovesicles contain a variety of biomolecules, and techniques for loading drugs into them play a significant part in the production of these DDS. Consequently, another difficult task is adding foreign chemical or biological components to nanovesicles. It is also essential to address the problems with releasing foreign hydrophilic macromolecules and the potential release of undesirable cargo elements found naturally in the exosomes.285 Because nanovesicles contain a portion of the original cell from which they are formed and have limited room for housing foreign agents, inadequate loading of medical payloads is another obstacle for nanovesicles.286 In order to accommodate the entrapment of foreign hydrophilic macromolecules, nanovesicles’ functionalization is therefore required.287 Another issue with nanovesicle composition is that it can convey unfavorable material from the producing cells being immunogenic and cancer-causing.229,288 Additionally, technological, financial, and legal difficulties have been identified as obstacles to the exploitation of nanovesicles in research.289 In order to overcome the challenges and utilize cell membrane-derived nanovesicles as drug-delivery vehicles in clinical settings, researchers have conducted numerous studies in this area. Nanovesicles must undergo a thorough in vivo examination to determine their strength and toxicity before using them as a nano-platform for the delivery of drug.
6. Conclusion and future perspective
The most recent developments in cell membrane-derived biomimetic nanovesicles for cancer phototherapy are summarized in this review. To produce enough heat or lethal ROS to destroy cancer cells, phototherapy calls for photoabsorbing substances or PSs that are successfully deposited in the tumor location. Traditional phototherapeutic nanoparticles, in contrast, frequently encounter problems such as early immune system identification and rapid clearance from blood circulation, which results in poor concentration in the targeted region. The cell-membrane coating method offers a particular biological contact to help overcome these constraints. This cell-membrane coating method is unaffected by the original, uncoated phototherapeutic nanoparticles’ biological activity and photophysical properties. Additionally, it permits the light-stimulated release of enclosed pharmaceuticals for synergistic and combination therapies, since the covered cell membrane can be ablated from phototherapeutic nanoparticles under laser irradiation. RBCM, cancer cell membrane, platelet membrane, macrophage membrane, and stem cell membrane produced nanovesicles from which nanovesicles were herein discussed. For the cell-membrane coating technique to be further improved, a few critical difficulties must be resolved. To maintain functionality during blood circulation, the coherence of the cell membrane and the core of the nanovesicle should firstly be preserved to the maximum extent. However, it has not yet been discovered how to correctly assess the integrity of nanovesicles produced from cell membranes in vivo. Secondly, the dense ECM and high interstitial fluid pressure (IFP) in the tumor microenvironment prevent cell membrane-derived nanovesicles from penetrating inside the tumor area, despite exhibiting unique advantages.290,291 The ability of the cell membrane-derived nanovesicles to kill deeply entrenched cancer cells may be improved by adding specific peptides or ligands.292,293 Finally, although the cell membrane-derived nanovesicles exhibit good in vivo biocompatibility and lower toxicity to healthy organs, further research is needed to determine their long-term biological impacts on living tissue. Since there are still significant amounts of administered cell membrane-camouflaged nanoparticles that accumulate in healthy organs, it is especially important to carefully evaluate their biological safety in healthy organs. Furthermore, the research work based on the preparation and modification of membrane-coated nanoparticles is still lagging behind in terms of processing parameters. Therefore, future work should mainly focus on the optimization of process parameters and modification strategies. The efficiency of modification strategies should be highlighted and assessed to develop high-quality functional nanomaterials. For this purpose, more relative terms should be introduced and evaluated, such as engineering cell membrane via cell modification for nanoscale therapies, innovative genetic engineering techniques to modify the cell surface, and production of novel bio-coating through membrane fusion.
Conflicts of interest
The authors declare no conflict of interest, financial or other.
Acknowledgements
This work has been financially supported by the National Natural Science Foundation of China (81973487, 81573617), the National Science and Technology major projects for “Major New Drugs Innovation and Development” (2018ZX09711003-008-002), and the Shanghai Committee of Science and Technology (22S21902100, 20S21900100 and 21S21902200). All the authors gratefully acknowledge the support provided by the National Natural Science Foundation of China, the National Science and Technology major projects, and the Shanghai Committee of Science and Technology.
References
- M. J. N. r. c. Ferrari, Cancer nanotechnology: opportunities and challenges, Nat. Rev. Cancer, 2005, 5(3), 161–171 CrossRef CAS PubMed
.
- R. Lozano,
et al., Global and regional mortality from 235 causes of death for 20 age groups in 1990 and 2010: a systematic analysis for the Global Burden of Disease Study 2010, Lancet, 2012, 380(9859), 2095–2128 CrossRef PubMed
.
- K. D. Miller,
et al., Cancer treatment and survivorship statistics, 2016, CA-Cancer J. Clin., 2016, 66(4), 271–289 CrossRef PubMed
.
- J. C. K. Amerigos Daddy,
et al., Co-Encapsulation of Mitoxantrone and β-Elemene in Solid Lipid Nanoparticles to Overcome Multidrug Resistance in Leukemia, Pharmaceutics, 2020, 12(2), 191 CrossRef
.
- M. W. Khan,
et al., Synergism of cisplatin-oleanolic acid co-loaded calcium carbonate nanoparticles on hepatocellular carcinoma cells for enhanced apoptosis and reduced hepatotoxicity, Int. J. Nanomed., 2019, 14, 3753 CrossRef CAS PubMed
.
-
D. Peer, et al., Nanocarriers as an emerging platform for cancer therapy, in Nano-Enabled Medical Applications, 2020, pp. 61–91 Search PubMed
.
- B. Yang, Y. Chen and J. J. A. m. Shi, Exosome biochemistry and advanced nanotechnology for next–generation theranostic platforms, Adv. Mater., 2019, 31(2), 1802896 CrossRef PubMed
.
- F. Raza,
et al., Paclitaxel-loaded pH responsive hydrogel based on self-assembled peptides for tumor targeting, Biomater. Sci., 2019, 7(5), 2023–2036 RSC
.
- Y. Zhu,
et al., Injectable pH and redox dual responsive hydrogel based on self-assembled peptides for anti-tumor drug delivery, Biomater. Sci., 2020, 8, 5415–5426 RSC
.
- Y. Liu,
et al., pH-Sensitive Peptide Hydrogels as a Combination Drug Delivery System for Cancer Treatment, Pharmaceutics, 2022, 14(3), 652 CrossRef CAS PubMed
.
- F. Raza,
et al., Recent advances in gelatin-based nanomedicine for targeted delivery of anti-cancer drugs, Curr. Pharm. Des., 2022, 28(5), 380–394 CrossRef CAS PubMed
.
- F. Raza,
et al., Recent advances in targeted delivery of paclitaxel nanomedicine for cancer therapy, Mater. Adv., 2022, 3, 2268–2290 RSC
.
- A. Ullah,
et al., A new approach based on CXCR4-targeted combination liposomes for the treatment of liver fibrosis, Biomater. Sci., 2022, 10(10), 2650–2664 RSC
.
- K. H. Ullah,
et al., Poloxamer 407 Based Gel Formulations for Transungual Delivery of Hydrophobic Drugs: Selection and Optimization of Potential Additives, Polymers, 2021, 13(19), 3376 CrossRef CAS
.
- X. Wang,
et al., Recent developments in mesoporous silica nanoparticles for tumor theranostic applications, Curr. Pharm. Des., 2022, 28, 151–164 CrossRef
.
- S. L. Topalian,
et al., Mechanism-driven biomarkers to guide immune checkpoint blockade in cancer therapy, Nat. Rev. Cancer, 2016, 16(5), 275–287 CrossRef CAS
.
- P. Gotwals,
et al., Prospects for combining targeted and conventional cancer therapy with immunotherapy, Nat. Rev. Cancer, 2017, 17(5), 286–301 CrossRef CAS PubMed
.
- T. Sun,
et al., Engineered nanoparticles for drug delivery in cancer therapy, Nanomaterials, 2021, 31–142 Search PubMed
.
- J. A. Kemp,
et al., “Combo” nanomedicine: co-delivery of multi-modal therapeutics for efficient, targeted, and safe cancer therapy, Adv. Drug Delivery Rev., 2016, 98, 3–18 CrossRef CAS
.
- J. I. Hare,
et al., Challenges and strategies in anti-cancer nanomedicine development: An industry perspective, Adv. Drug Delivery Rev., 2017, 108, 25–38 CrossRef CAS PubMed
.
- O. C. Farokhzad and R. J. A. n. Langer, Impact of nanotechnology on drug delivery, ACS Nano, 2009, 3(1), 16–20 CrossRef CAS PubMed
.
- G. Chen,
et al., Nanochemistry and nanomedicine for nanoparticle-based diagnostics and therapy, Chem. Rev., 2016, 116(5), 2826–2885 CrossRef CAS
.
- E.-K. Lim,
et al., Nanomaterials for theranostics: recent advances and future challenges, Chem. Rev., 2015, 115(1), 327–394 CrossRef CAS
.
- F. Raza,
et al., Cancer nanomedicine: focus on recent developments and self-assembled peptide nanocarriers, J. Mater. Chem. B, 2019, 7(48), 7639–7655 RSC
.
- M. W. Tibbitt, J. E. Dahlman and R. Langer, Emerging frontiers in drug delivery, J. Am. Chem. Soc., 2016, 138(3), 704–717 CrossRef CAS PubMed
.
- M. P. Stewart,
et al., In vitro and ex vivo strategies for intracellular delivery, Nature, 2016, 538(7624), 183–192 CrossRef CAS PubMed
.
- H. Zafar,
et al., Recent progress of nanomedicine-induced ferroptosis for cancer therapy, Biomater. Sci., 2021, 9, 5092–5115 RSC
.
- C. Liang,
et al., Emerging nanomedicine approaches fighting tumor metastasis: animal models, metastasis-targeted drug delivery, phototherapy, and immunotherapy, Chem. Soc. Rev., 2016, 45(22), 6250–6269 RSC
.
- T. Xu,
et al., Enhanced ferroptosis by oxygen-boosted phototherapy based on a 2-in-1 nanoplatform of ferrous hemoglobin for tumor synergistic therapy, ACS Nano, 2020, 14(3), 3414–3425 CrossRef CAS
.
- L. Zhang, H. Lin and Y. Chen,
et al., Mitochondria-Targeted Artificial” Nano-RBCs” for Amplified 12 Synergistic Cancer Phototherapy by a Single NIR Irradiation, Adv. Sci., 2018, 5(13), 1800049 CrossRef
.
- W. Sun,
et al., Boron dipyrromethene nano–photosensitizers for anticancer phototherapies, Small, 2019, 15(32), 1804927 CrossRef
.
- M. M. Agwa,
et al., Carbohydrate ligands-directed active tumor targeting of combinatorial chemotherapy/phototherapy-based nanomedicine: A review, Int. J. Biol. Macromol., 2023, 124294 CrossRef CAS
.
- Z. Xie,
et al., Emerging combination strategies with phototherapy in cancer nanomedicine, Chem. Soc. Rev., 2020, 49(22), 8065–8087 RSC
.
- C. W. Ng, J. Li and K. Pu, Phototherapy–Synergized Cancer Immunotherapy: Recent Progresses in Phototherapy–Synergized Cancer Immunotherapy (Adv. Funct. Mater. 46/2018), Adv. Funct. Mater., 2018, 28(46), 1870327 CrossRef
.
- S. Gan,
et al., Covalent organic framework–supported molecularly dispersed near–infrared dyes boost immunogenic phototherapy against tumors, Adv. Funct. Mater., 2019, 29(46), 1902757 CrossRef CAS
.
- M. Wang,
et al., Nir–triggered phototherapy and immunotherapy via an antigen–capturing nanoplatform for metastatic cancer treatment, Adv. Sci., 2019, 6(10), 1802157 CrossRef
.
- S. Garbujo, Design, fabrication, and biochemical investigation of cancer cell membrane derived nano-vesicles for the development of intelligent RNA delivery systems, Bicocca Open Archive, 2023 Search PubMed
, https://boa.unimib.it/handle/10281/408697.
- M. Wang,
et al., Engineering SIRPα cellular membrane-based nanovesicles for combination immunotherapy, Nano Res., 2023, 1–9 Search PubMed
.
- Q. F. Meng,
et al., Genetically programmable fusion cellular vesicles for cancer immunotherapy, Angew. Chem., 2021, 133(50), 26524–26530 CrossRef
.
- H. Shao,
et al., New technologies for analysis of extracellular vesicles, Chem. Rev., 2018, 118(4), 1917–1950 CrossRef CAS PubMed
.
- J. R. Lee,
et al., Targeted Delivery of Apoptotic Cell–Derived Nanovesicles prevents Cardiac Remodeling and Attenuates Cardiac Function Exacerbation, Adv. Funct. Mater., 2023, 33(23), 2210864 CrossRef CAS
.
- T. Feng,
et al., Engineered exosomes as a natural nanoplatform for cancer targeted delivery of metal-based drugs, Signal Transduction Targeted Ther., 2022, 454, 214325 CAS
.
- S. Rani and T. J. A. m. Ritter, The exosome–A naturally secreted nanoparticle and its application to wound healing, Adv. Mater., 2016, 28(27), 5542–5552 CrossRef CAS
.
- M. F. S. Lindenbergh and W. Stoorvogel, Antigen presentation by extracellular vesicles from professional antigen-presenting cells., Annu. Rev. Immunol., 2018, 36(1), 435–459 CrossRef CAS
.
- Y. Chen,
et al., Two-dimensional graphene analogues for biomedical applications, Chem. Soc. Rev., 2015, 44(9), 2681–2701 RSC
.
- X. Y. Zhu,
et al., Immunocyte–Derived Nanodrugs for Cancer Therapy, Adv. Funct. Mater., 2022, 32(51), 2207181 CrossRef CAS
.
- B. S. Pattni, V. V. Chupin and V. P. J. C. r. Torchilin, New developments in liposomal drug delivery, Chem. Rev., 2015, 115(19), 10938–10966 CrossRef CAS
.
- M. W. Tibbitt, J. E. Dahlman and R. Langer, Emerging frontiers in drug delivery, J. Am. Chem. Soc., 2016, 138(3), 704–717 CrossRef CAS
.
- Y. Min,
et al., Clinical translation of nanomedicine, Chem. Rev., 2015, 115(19), 11147–11190 CrossRef CAS
.
- D. Lopes,
et al., Bioengineered exosomal-membrane-camouflaged abiotic nanocarriers: neurodegenerative diseases, tissue engineering and regenerative medicine, Mil. Med. Res., 2023, 10(1), 1–26 Search PubMed
.
- J. Kowal, M. Tkach and C. Théry, Biogenesis and secretion of exosomes, Curr. Opin. Cell Biol., 2014, 29, 116–125 CrossRef CAS PubMed
.
- S. Kourembanas, Exosomes: vehicles of intercellular signaling, biomarkers, and vectors of cell therapy, Annu. Rev. Physiol., 2015, 77, 13–27 CrossRef CAS PubMed
.
- C. Gardiner,
et al., Techniques used for the isolation and characterization of extracellular vesicles: results of a worldwide survey, J. Extracell. Vesicles, 2016, 5(1), 32945 CrossRef PubMed
.
- M. L. Merchant,
et al., Isolation and characterization of urinary extracellular vesicles: implications for biomarker discovery, Nat. Rev. Physiol., 2017, 13(12), 731–749 CAS
.
- N. Yim,
et al., Exosome engineering for efficient intracellular delivery of soluble proteins using optically reversible protein–protein interaction module, Nat. Commun., 2016, 7(1), 1–9 Search PubMed
.
- E. Koh,
et al., Exosome-SIRPα, a CD47 blockade increases cancer cell phagocytosis, Biomaterials, 2017, 121, 121–129 CrossRef CAS PubMed
.
- S. Kamerkar,
et al., Exosomes facilitate therapeutic targeting of oncogenic KRAS in pancreatic cancer, Nature, 2017, 546(7659), 498–503 CrossRef CAS PubMed
.
- G. Kibria,
et al., Dual-ligand modification of PEGylated liposomes shows better cell selectivity and efficient gene delivery, J. Controlled Release, 2011, 153(2), 141–148 CrossRef CAS PubMed
.
- F. Raza,
et al., A review on recent advances in stabilizing peptides/proteins upon fabrication in hydrogels from biodegradable polymers, Pharmaceutics, 2018, 10(1), 16 CrossRef PubMed
.
-
Y.-H. Ou, et al., Extracellular Vesicle (EV) biohybrid systems for cancer therapy: Recent advances and future perspectives, in Seminars in Cancer Biology, 2021, Elsevier Search PubMed
.
- L. Cheng,
et al., Functional nanomaterials for phototherapies of cancer, Chem. Rev., 2014, 114(21), 10869–10939 CrossRef CAS PubMed
.
- G. Lan, K. Ni and W. J. C. c. r. Lin, Nanoscale metal–organic frameworks for phototherapy of cancer, Coord. Chem. Rev., 2019, 379, 65–81 CrossRef CAS PubMed
.
- V. Shanmugam, S. Selvakumar and C.-S. Yeh, Near-infrared light-responsive nanomaterials in cancer therapeutics, Chem. Soc. Rev., 2014, 43(17), 6254–6287 RSC
.
- W. Sun,
et al., Boron dipyrromethene nano–photosensitizers for anticancer phototherapies, Small, 2019, 15(32), 1804927 CrossRef PubMed
.
- J. Li and K. Pu, Development of organic semiconducting materials for deep-tissue optical imaging, phototherapy and photoactivation, Chem. Soc. Rev., 2019, 48(1), 38–71 RSC
.
- J. Liu,
et al., Recent progress in the development of multifunctional nanoplatform for precise tumor phototherapy, Adv. Healthcare Mater., 2021, 10(1), 2001207 CrossRef CAS
.
- M. P. Stewart,
et al., In vitro and ex vivo strategies for intracellular delivery, Nature, 2016, 538(7624), 183–192 CrossRef CAS PubMed
.
- Z. Li,
et al., Mesoporous silica nanoparticles in biomedical applications, Chem. Soc. Rev., 2012, 41(7), 2590–2605 RSC
.
- L. A. Austin,
et al., The optical, photothermal, and facile surface chemical properties of gold and silver nanoparticles in biodiagnostics, therapy, and drug delivery, Arch. Toxicol., 2014, 88(7), 1391–1417 CrossRef CAS
.
- R. Di Corato,
et al., Magnetic nanobeads decorated with silver nanoparticles as cytotoxic agents and photothermal probes, Small, 2012, 8(17), 2731–2742 CrossRef CAS PubMed
.
- C. Liang,
et al., Tumor metastasis inhibition by imaging–guided photothermal therapy with single–walled carbon nanotubes, Adv. Mater., 2014, 26(32), 5646–5652 CrossRef CAS PubMed
.
- G. Hong,
et al., Carbon nanomaterials for biological imaging and nanomedicinal therapy, Chem. Rev., 2015, 115(19), 10816–10906 CrossRef CAS PubMed
.
- A. Espinosa,
et al., Duality of iron oxide nanoparticles in cancer therapy: amplification of heating efficiency by magnetic hyperthermia and photothermal bimodal treatment, ACS Nano, 2016, 10(2), 2436–2446 CrossRef CAS PubMed
.
- H. Zhu,
et al., Ternary chalcogenide nanosheets with ultrahigh photothermal conversion efficiency for photoacoustic theranostics, Small, 2017, 13(16), 1604139 CrossRef
.
- W. Tao,
et al., Antimonene quantum dots: synthesis and application as near–infrared photothermal agents for effective cancer therapy, Angew. Chem., 2017, 129(39), 12058–12062 CrossRef
.
- K. Lu, C. He and W. Lin, A chlorin-based nanoscale metal–organic framework
for photodynamic therapy of colon cancers, J. Am. Chem. Soc., 2015, 137(24), 7600–7603 CrossRef CAS PubMed
.
- H. S. Jung,
et al., Organic molecule-based photothermal agents: an expanding photothermal therapy universe, Chem. Soc. Rev., 2018, 47(7), 2280–2297 RSC
.
- L. Y. T. Chou, K. Ming and W. C. W. Chan, Strategies for the intracellular delivery of nanoparticles, Chem. Soc. Rev., 2011, 40(1), 233–245 RSC
.
- W.-K. Oh,
et al., Cellular uptake, cytotoxicity, and innate immune response of silica− titania hollow nanoparticles based on size and surface functionality, ACS Nano, 2010, 4(9), 5301–5313 CrossRef CAS
.
- B. K. Lee, Y. H. Yun and K. Park, Smart nanoparticles for drug delivery: Boundaries and opportunities, Chem. Eng. Sci., 2015, 125, 158–164 CrossRef CAS PubMed
.
- H. J. A. d. d. r. Maeda, Toward a full understanding of the EPR effect in primary and metastatic tumors as well as issues related to its heterogeneity, Adv. Drug Delivery Rev., 2015, 91, 3–6 CrossRef CAS PubMed
.
- A. J. Wilhelm, Q. D. Tavares, S. Ohta, J. Audet, H. F. Dvorak and W. C. W. Chan, Nat. Rev. Mater., 2016, 1, 16014 CrossRef
.
- A. Sedlmeier and H. H. Gorris, Surface modification and characterization of photon-upconverting nanoparticles for bioanalytical applications, Chem. Soc. Rev., 2015, 44(6), 1526–1560 RSC
.
- K. Knop,
et al., Poly (ethylene glycol) in drug delivery: pros and cons as well as potential alternatives, Angew. Chem., Int. Ed., 2010, 49(36), 6288–6308 CrossRef CAS PubMed
.
- P. Zhang,
et al., Anti-PEG antibodies in the clinic: Current issues and beyond PEGylation, J. Controlled Release, 2016, 244, 184–193 CrossRef CAS
.
- S. Aryal,
et al., Erythrocyte membrane-cloaked polymeric nanoparticles for controlled drug loading and release, Nanomedicine, 2013, 8(8), 1271–1280 CrossRef CAS PubMed
.
- C.-M. J. Hu,
et al., Nanoparticle biointerfacing by platelet membrane cloaking, Nature, 2015, 526(7571), 118–121 CrossRef CAS PubMed
.
- L. Rao,
et al., Cancer cell membrane–coated upconversion nanoprobes for highly specific tumor imaging, Adv. Mater., 2016, 28(18), 3460–3466 CrossRef CAS PubMed
.
- R. H. Fang,
et al., Cell membrane-derived nanomaterials for biomedical applications, Biomaterials, 2017, 128, 69–83 CrossRef CAS PubMed
.
- R. H. Fang,
et al., Engineered nanoparticles mimicking cell membranes for toxin neutralization, Adv. Drug Delivery Rev., 2015, 90, 69–80 CrossRef CAS
.
- R. H. Fang,
et al., Cancer cell membrane-coated nanoparticles for anticancer vaccination and drug delivery, Nano Lett., 2014, 14(4), 2181–2188 CrossRef CAS PubMed
.
- R. H. Fang,
et al., Cell membrane coating nanotechnology, Adv. Mater., 2018, 30(23), 1706759 CrossRef PubMed
.
- J. H. Park,
et al., Virus–mimicking cell membrane–coated nanoparticles for cytosolic delivery of mRNA, Angew. Chem., 2022, 134(2), e202113671 CrossRef
.
- C.-M. J. Hu,
et al., Erythrocyte membrane-camouflaged polymeric nanoparticles as a biomimetic delivery platform, Proc. Natl. Acad. Sci. U. S. A., 2011, 108(27), 10980–10985 CrossRef CAS
.
- F. Raza,
et al., Recent Advances in Cell Membrane–Derived Biomimetic Nanotechnology for Cancer Immunotherapy, Adv. Healthcare Mater., 2021, 10(6), 2002081 CrossRef CAS PubMed
.
- D. Zhi,
et al., Photothermal therapy, J. Controlled Release, 2020, 325, 52–71 CrossRef CAS PubMed
.
- S. Liu, X. Pan and H. J. A. C. Liu, Two–dimensional nanomaterials for photothermal therapy, Angew. Chem., 2020, 132(15), 5943–5953 CrossRef
.
- W. Zhang,
et al., Immunosuppressive microenvironment improvement and treatment of aggressive malignancy pancreatic ductal adenocarcinoma based on local administration of injectable hydrogel, Nano Today, 2023, 50, 101832 CrossRef CAS
.
- X. Ren,
et al., Red blood cell membrane camouflaged magnetic nanoclusters for imaging-guided photothermal therapy, Biomaterials, 2016, 92, 13–24 CrossRef CAS PubMed
.
- X. Song,
et al., J–Aggregates of organic dye molecules complexed with iron oxide nanoparticles for imaging–guided photothermal therapy under 915−nm light, Small, 2014, 10(21), 4362–4370 CrossRef CAS PubMed
.
- Q. Ding,
et al., Mitochondria-targeted Fluorophores for in vivo NIR-II Imaging-guided PDT/PTT, Chem. Commun., 2023, 59, 8127–8130 RSC
.
- K. Yang,
et al., FeS nanoplates as a multifunctional nano-theranostic for magnetic resonance imaging guided photothermal therapy, Biomaterials, 2015, 38, 1–9 CrossRef CAS
.
- J. He,
et al., Core− shell structured hollow copper sulfide@ metal− organic framework for magnetic resonance imaging guided photothermal therapy in second near-infrared biological window, Biochem. Biophys. Res. Commun., 2023, 638, 51–57 CrossRef CAS PubMed
.
- L. Zhang,
et al., A novel single walled carbon nanotube (SWCNT) functionalization agent facilitating in vivo combined chemo/thermo therapy, Nanoscale, 2015, 7(39), 16204–16213 RSC
.
- C. Chen,
et al., Hemoglobin-Decorated Boron-Carbon Nanosheets with Catalytic Ability and Near-Infrared II Light Response for Tumor Photothermal-Chemodynamic Therapy, ACS Appl. Nano Mater., 2023, 6(9), 7572–7581 CrossRef CAS
.
- M. Rahman,
et al., Emerging advances in cancer nanotheranostics with graphene nanocomposites: opportunities and challenges, Nanomedicine, 2015, 10(15), 2405–2422 CrossRef CAS PubMed
.
- S. Mallidi,
et al., Beyond the barriers of light penetration: strategies, perspectives and possibilities for photodynamic therapy, Theranostics, 2016, 6(13), 2458 CrossRef CAS PubMed
.
- R. V. Huis in ‘t Veld,
et al., Current Challenges and Opportunities of Photodynamic Therapy against Cancer, Pharmaceutics, 2023, 15(2), 330 CrossRef PubMed
.
- M. Jain,
et al., Photodynamic therapy for the treatment of atherosclerotic plaque: Lost in translation?, Cardiovasc. Ther., 2017, 35(2), e12238 CrossRef PubMed
.
- C. Rajagopal and K. J. F. i. o. Harikumar, The origin and functions of exosomes in cancer, Front. Oncol., 2018, 8, 66 CrossRef PubMed
.
- K. Aubertin,
et al., Massive release of extracellular vesicles from cancer cells after photodynamic treatment or chemotherapy, Sci. Rep., 2016, 6(1), 1–11 CrossRef PubMed
.
- L. Rao,
et al., Red blood cell membrane as a biomimetic nanocoating for prolonged circulation time and reduced accelerated blood clearance, Small, 2015, 11(46), 6225–6236 CrossRef CAS PubMed
.
- Y. Li,
et al., Clinical progress and advanced research of red blood cells based drug delivery system, Biomaterials, 2021, 279, 121202 CrossRef CAS PubMed
.
- X. Zhen, P. Cheng and K. J. S. Pu, Recent advances in cell membrane–camouflaged nanoparticles for cancer phototherapy, Small, 2019, 15(1), 1804105 CrossRef PubMed
.
- Z. Cheng,
et al., Autologous erythrocytes delivery of berberine hydrochloride with long-acting effect for hypolipidemia treatment, Drug Delivery, 2020, 27(1), 283–291 CrossRef CAS PubMed
.
- Y. Lian,
et al., Erythrocyte membrane-coated arsenic trioxide-loaded sodium alginate nanoparticles for tumor therapy, Pharmaceutics, 2020, 12(1), 21 CrossRef CAS PubMed
.
- X. Wu,
et al., Red Blood Cell Membrane-Camouflaged Tedizolid Phosphate-Loaded PLGA Nanoparticles for Bacterial-Infection Therapy, Pharmaceutics, 2021, 13(1), 99 CrossRef CAS PubMed
.
- A. Antonelli,
et al., Development of long circulating magnetic particle imaging tracers: use of novel magnetic nanoparticles and entrapment into human erythrocytes, Nanomedicine, 2020, 15(08), 739–753 CrossRef CAS
.
- Y. R. Neupane,
et al., Cell-derived nanovesicles from mesenchymal stem cells as extracellular vesicle-mimetics in wound healing, Acta Pharm. Sin. B, 2023, 13(5), 1887–1902 CrossRef CAS PubMed
.
- E. Xu,
et al., Study on the protection of dextran on erythrocytes during drug loading, Colloids Surf., B, 2020, 189, 110882 CrossRef CAS PubMed
.
- S. Malhotra,
et al., Red blood cells membrane–derived nanoparticles: Applications and key challenges in their clinical translation, Wiley Interdiscip. Rev.: Nanomed. Nanobiotechnol., 2022, e1776 CAS
.
- Z. Kamal,
et al., Red blood cell membrane-camouflaged vancomycin and chlorogenic acid-loaded gelatin nanoparticles against multi-drug resistance infection mice model, J. Drug Delivery Sci. Technol., 2022, 103706 CrossRef CAS
.
- R. Rong,
et al., Blood cell-based drug delivery systems: a biomimetic platform for antibacterial therapy, Eur. J. Pharm. Biopharm., 2022, 177, 273–288 CrossRef CAS PubMed
.
- F. He,
et al., Red Blood Cell Membrane-Coated Ultrasmall NaGdF4 Nanoprobes for High-Resolution 3D Magnetic Resonance Angiography, ACS Appl. Mater. Interfaces, 2022, 14, 26372–26381 CrossRef CAS PubMed
.
- X. Liang,
et al., Photothermal cancer immunotherapy by erythrocyte membrane-coated black phosphorus formulation, J. Controlled Release, 2019, 296, 150–161 CrossRef CAS PubMed
.
- X. Wang,
et al., Enhanced photothermal therapy of biomimetic polypyrrole nanoparticles through improving blood flow perfusion, Biomaterials, 2017, 143, 130–141 CrossRef CAS PubMed
.
- M. Aquib,
et al., Targeted and stimuli–responsive mesoporous silica nanoparticles for drug delivery and theranostic use, J. Biomed. Mater. Res., Part A, 2019, 107(12), 2643–2666 CrossRef CAS PubMed
.
- S. Ye,
et al., Light/pH-triggered biomimetic red blood cell membranes camouflaged small molecular drug assemblies for imaging-guided combinational chemo-photothermal therapy, ACS Appl. Mater. Interfaces, 2019, 11(17), 15262–15275 CrossRef CAS PubMed
.
- Q. Jiang,
et al., Red blood cell membrane-camouflaged melanin nanoparticles for enhanced photothermal therapy, Biomaterials, 2017, 143, 29–45 CrossRef CAS PubMed
.
- W. Chen,
et al., Cell membrane camouflaged hollow prussian blue nanoparticles for synergistic photothermal–/chemotherapy of cancer, Adv. Funct. Mater., 2017, 27(11), 1605795 CrossRef
.
- B. Liu,
et al., RBC membrane camouflaged prussian blue nanoparticles for gamabutolin loading and combined chemo/photothermal therapy of breast cancer, Biomaterials, 2019, 217, 119301 CrossRef CAS PubMed
.
- H. Cao,
et al., Liposomes coated with isolated macrophage membrane can target lung metastasis of breast cancer, ACS Nano, 2016, 10(8), 7738–7748 CrossRef CAS PubMed
.
- Y. Yu,
et al., Boosting B Cell and Macrophage–Mediated Humoral Immunity with Fusion Nanovesicles for Triple–Negative Breast Cancer Combined Therapy, Adv. Healthcare Mater., 2023, 12(6), 2202209 CrossRef CAS PubMed
.
- M. Xuan,
et al., Macrophage cell membrane camouflaged mesoporous silica nanocapsules for in vivo cancer therapy, Adv. Healthcare Mater., 2015, 4(11), 1645–1652 CrossRef CAS PubMed
.
- M. Xuan,
et al., Macrophage cell membrane camouflaged Au nanoshells for in vivo prolonged circulation life and enhanced cancer photothermal therapy, ACS Appl. Mater. Interfaces, 2016, 8(15), 9610–9618 CrossRef CAS PubMed
.
- C.-M. J. Hu,
et al., Nanoparticle biointerfacing by platelet membrane cloaking, Nature, 2015, 526(7571), 118–121 CrossRef CAS PubMed
.
- J. Wang,
et al., Designer exosomes for active targeted chemo–photothermal synergistic tumor therapy, Adv. Funct. Mater., 2018, 28(18), 1707360 CrossRef
.
- Q.-F. Meng,
et al., Macrophage membrane-coated iron oxide nanoparticles for enhanced photothermal tumor therapy, Nanotechnology, 2018, 29(13), 134004 CrossRef PubMed
.
- H. Zhao,
et al., C–C chemokine ligand 2 (CCl2) recruits macrophage-membrane-camouflaged hollow bismuth selenide nanoparticles to facilitate photothermal sensitivity and inhibit lung metastasis of breast cancer, ACS Appl. Mater. Interfaces, 2018, 10(37), 31124–31135 CrossRef CAS PubMed
.
- U. Ruman,
et al., Nanocarrier-based therapeutics and theranostics drug delivery systems for next generation of liver cancer nanodrug modalities, Int. J. Nanomed., 2020, 15, 1437 CrossRef CAS PubMed
.
- Z. Dai,
et al., Platelet-derived extracellular vesicles ameliorate intervertebral disc degeneration by alleviating mitochondrial dysfunction, Mater. Today Bio, 2023, 18, 100512 CrossRef CAS PubMed
.
- J. Gao, D. Chu and Z. J. J. o. c. r. Wang, Cell membrane-formed nanovesicles for disease-targeted delivery, J. Controlled Release, 2016, 224, 208–216 CrossRef CAS PubMed
.
- S. T. Yurkin and Z. J. N. Wang, Cell membrane-derived nanoparticles: emerging clinical opportunities for targeted drug delivery, Nanomedicine, 2017, 12(16), 2007–2019 CrossRef CAS PubMed
.
- M. J. C. Holinstat and M. Reviews, Normal platelet function, Cancer Metastasis Rev., 2017, 36(2), 195–198 CrossRef CAS PubMed
.
- J. Kim,
et al., Co-coating of receptor-targeted drug nanocarriers with anti-phagocytic moieties enhances specific tissue uptake versus non-specific phagocytic clearance, Biomaterials, 2017, 147, 14–25 CrossRef CAS PubMed
.
- Q. Hu,
et al., Nanomedicine: Anticancer Platelet–Mimicking Nanovehicles (Adv. Mater. 44/2015), Adv. Mater., 2015, 27(44), 7014–7014 CrossRef CAS
.
- E. van der Pol and P. J. p. Harrison, From platelet dust to gold dust: physiological importance and detection of platelet microvesicles, Platelets, 2017, 28(3), 211–213 CrossRef CAS PubMed
.
- M. Żmigrodzka, O. Witkowska-Piłaszewicz and A. J. I. j. o. m. s. Winnicka, Platelets extracellular vesicles as regulators of cancer progression—an updated perspective, Int. J. Mol. Sci., 2020, 21(15), 5195 CrossRef PubMed
.
- Y. Ma,
et al., A cascade synergetic strategy induced by photothermal effect based on platelet exosome nanoparticles for tumor therapy, Biomaterials, 2022, 282, 121384 CrossRef CAS PubMed
.
- H. Ye,
et al., Bioinspired nanoplatelets for chemo-photothermal therapy of breast cancer metastasis inhibition, Biomaterials, 2019, 206, 1–12 CrossRef CAS PubMed
.
- L. Wu,
et al., Platelet membrane-coated nanoparticles for targeted drug delivery and local chemo-photothermal therapy of orthotopic hepatocellular carcinoma, J. Mater. Chem. B, 2020, 8(21), 4648–4659 RSC
.
- L. Rao,
et al., Antitumor platelet–mimicking magnetic nanoparticles, Adv. Funct. Mater., 2017, 27(9), 1604774 CrossRef
.
- S. Zhang,
et al., Autocatalytic Delivery of Brain Tumor–Targeting, Size–Shrinkable Nanoparticles for Treatment of Breast Cancer Brain Metastases, Adv. Funct. Mater., 2020, 30(14), 1910651 CrossRef CAS PubMed
.
- J. Li,
et al., Cell membrane coated semiconducting polymer nanoparticles for enhanced multimodal cancer phototheranostics, ACS Nano, 2018, 12(8), 8520–8530 CrossRef CAS PubMed
.
- H. He,
et al., Leutusome: a biomimetic nanoplatform integrating plasma membrane components of leukocytes and tumor cells for remarkably enhanced solid tumor homing, Nano Lett., 2018, 18(10), 6164–6174 CrossRef CAS PubMed
.
- G. Deng,
et al., Cell-membrane immunotherapy based on natural killer cell membrane coated nanoparticles for the effective inhibition of primary and abscopal tumor growth, ACS Nano, 2018, 12(12), 12096–12108 CrossRef CAS PubMed
.
- P. Vader,
et al., Extracellular vesicles for drug delivery, Adv. Drug Delivery Rev., 2016, 106, 148–156 CrossRef CAS PubMed
.
- Y. Teng,
et al., MVP-mediated exosomal sorting of miR-193a promotes colon cancer progression, Nat. Commun., 2017, 8(1), 1–16 CrossRef PubMed
.
- Y. Wang,
et al., Mesoporous silica nanoparticles in drug delivery and biomedical applications, Nanomedicine, 2015, 11(2), 313–327 CrossRef CAS PubMed
.
- H. E. Shin, S. W. Oh and W. Park, Hybrid nanovesicle of chimeric antigen receptor (CAR)-engineered cell-derived vesicle and drug-encapsulated liposome for effective cancer treatment, J. Ind. Eng. Chem., 2023, 122, 127–137 CrossRef
.
- Z. Cong,
et al., Size-transformable hyaluronan stacked self-assembling peptide nanoparticles for improved transcellular tumor penetration and photo–chemo combination therapy, ACS Nano, 2020, 14(2), 1958–1970 CrossRef CAS PubMed
.
- X. Guo,
et al., In vivo photothermal inhibition of methicillin-resistant Staphylococcus aureus infection by in situ templated formulation of pathogen-targeting phototheranostics, Nanoscale, 2020, 12(14), 7651–7659 RSC
.
- J. Li and K. Pu, Semiconducting polymer nanomaterials as near-infrared photoactivatable protherapeutics for cancer., Acc. Chem. Res., 2020, 53(4), 752–762 CrossRef CAS PubMed
.
- Y. Tang,
et al., Transformable nanotherapeutics enabled by ICG: towards enhanced tumor penetration under NIR light irradiation, Nanoscale, 2019, 11(13), 6217–6227 RSC
.
- X. Li,
et al., Core–satellite nanomedicines for in vivo real-time monitoring of enzyme-activatable drug release by fluorescence and photoacoustic dual-modal imaging, ACS Nano, 2018, 13(1), 176–186 CrossRef PubMed
.
- Y. Xin,
et al., Recent progress on nanoparticle-based drug delivery systems for cancer therapy, Cancer Biol. Med., 2017, 14(3), 228 CrossRef CAS PubMed
.
- H. Ye,
et al., Nanosponges of circulating tumor-derived exosomes for breast cancer metastasis inhibition, Biomaterials, 2020, 242, 119932 CrossRef CAS PubMed
.
- M. Wu,
et al., Melanoma cell membrane biomimetic versatile CuS nanoprobes for homologous targeting photoacoustic imaging and photothermal chemotherapy, ACS Appl. Mater. Interfaces, 2020, 12(14), 16031–16039 CrossRef CAS PubMed
.
- F. Yan,
et al., NIR-laser-controlled drug release from DOX/IR-780-loaded temperature-sensitive-liposomes for chemo-photothermal synergistic tumor therapy, Theranostics, 2016, 6(13), 2337 CrossRef CAS PubMed
.
- R. Tian,
et al., Tumor exosome mimicking nanoparticles for tumor combinatorial chemo-photothermal therapy, Front. Bioeng. Biotechnol., 2020, 8, 1010 CrossRef PubMed
.
- J. Shen,
et al., Cancer cell membrane camouflaged iridium complexes functionalized black-titanium nanoparticles for hierarchical-targeted synergistic NIR-II photothermal and sonodynamic therapy, Biomaterials, 2021, 275, 120979 CrossRef CAS PubMed
.
- Z. Chen,
et al., Cancer cell membrane–biomimetic nanoparticles for homologous-targeting dual-modal imaging and photothermal therapy, ACS Nano, 2016, 10(11), 10049–10057 CrossRef CAS PubMed
.
- X. Lin,
et al., Biomimetic nanoprobe-augmented triple therapy with photothermal, sonodynamic and checkpoint blockade inhibits tumor growth and metastasis, J. Nanobiotechnol., 2022, 20(1), 1–21 CrossRef PubMed
.
- H. Kim,
et al., Cooperative tumour cell membrane targeted phototherapy, Nat. Commun., 2017, 8(1), 1–10 CrossRef PubMed
.
- Y. Li,
et al., Biologically excretable AIE nanoparticles wear tumor cell-derived “exosome caps” for efficient NIR-II
fluorescence imaging-guided photothermal therapy, ACS Nano, 2021, 41, 101333 CAS
.
- Q. Chen,
et al., Photothermal therapy: Photothermal therapy promotes tumor infiltration and antitumor activity of CAR T cells (Adv. Mater. 23/2019), Adv. Mater., 2019, 31(23), 1970166 CrossRef
.
- J. Nam,
et al., Chemo-photothermal therapy combination elicits anti-tumor immunity against advanced metastatic cancer, Nat. Commun., 2018, 9(1), 1–13 CrossRef CAS PubMed
.
- X. Zhang,
et al., Somatostatin Receptor–Mediated Tumor–Targeting Nanocarriers Based on Octreotide–PEG Conjugated Nanographene Oxide for Combined Chemo and Photothermal Therapy, Small, 2016, 12(26), 3578–3590 CrossRef CAS PubMed
.
- Q. Chen,
et al., Photothermal therapy with immune-adjuvant nanoparticles together with checkpoint blockade for effective cancer immunotherapy, Nat. Commun., 2016, 7(1), 1–13 CrossRef
.
- Q. Liu,
et al., Immunogenic exosome-encapsulated black phosphorus nanoparticles as an effective anticancer photo-nanovaccine, Nanoscale, 2020, 12(38), 19939–19952 RSC
.
- I. Ferreira-Faria,
et al., Stem cell membrane-coated abiotic nanomaterials for biomedical applications, J. Controlled Release, 2022, S0168-3659(22) 00596 Search PubMed
.
- Y. Wu,
et al., A mesenchymal stem cell-derived nanovesicle-biopotentiated bovine serum albumin-bridged gelatin hydrogel for enhanced diabetic wound therapy, Mater. Des., 2023, 230, 111960 CrossRef CAS
.
- C. Gao,
et al., Stem cell membrane–coated nanogels for highly efficient in vivo tumor targeted drug delivery, Small, 2016, 12(30), 4056–4062 CrossRef CAS PubMed
.
- H. Ding,
et al., Erythrocyte membrane-coated NIR-triggered biomimetic nanovectors with programmed delivery for photodynamic therapy of cancer., Nanoscale, 2015, 7(21), 9806–9815 RSC
.
- E. Song,
et al., Antibody mediated in vivo delivery of small interfering RNAs via cell-surface receptors., Nat. Rev. Drug Discovery, 2005, 23(6), 709–717 CAS
.
- K. B. Knudsen,
et al., In vivo toxicity of cationic micelles and liposomes, Nanomedicine, 2015, 11(2), 467–477 CrossRef CAS PubMed
.
- S. Sharifi,
et al., Superparamagnetic iron oxide nanoparticles for in vivo molecular and cellular imaging, Contrast Media Mol. Imaging, 2015, 10(5), 329–355 CrossRef CAS PubMed
.
- X. Mu,
et al., EGFR-targeted delivery of DOX-loaded Fe3O4@ polydopamine multifunctional nanocomposites for MRI and antitumor chemo-photothermal therapy., Int. J. Nanomed., 2017, 12, 2899 CrossRef CAS PubMed
.
- R. Ge,
et al., Fe3O4@ polydopamine composite theranostic superparticles employing preassembled Fe3O4 nanoparticles as the core, ACS Appl. Mater. Interfaces, 2016, 8(35), 22942–22952 CrossRef CAS PubMed
.
- L.-S. Lin, Z.-X. Cong, J.-B. Cao, K.-M. Ke, Q.-L. Peng, J. Gao, H.-H. Yang, G. Liu and X. Chen, Multifunctional Fe3O4@ polydopamine core–shell nanocomposites for intracellular mRNA detection and imaging-guided photothermal therapy, ACS Nano, 2014, 8(4), 3876–3883 CrossRef CAS PubMed
.
- X. Mu,
et al., siRNA delivery with stem cell membrane-coated magnetic nanoparticles for imaging-guided photothermal therapy and gene therapy, ACS Biomater. Sci. Eng., 2018, 4(11), 3895–3905 CrossRef CAS PubMed
.
- L. Zou,
et al., Current approaches of photothermal therapy in treating cancer metastasis with nanotherapeutics, Theranostics, 2016, 6(6), 762 CrossRef CAS PubMed
.
- G. Li,
et al., Engineered exosome for NIR-triggered drug delivery and superior synergistic chemo-phototherapy in a glioma model, Signal Transduction Targeted Ther., 2020, 20, 100723 Search PubMed
.
- W.-L. Liu,
et al., Cytomembrane nanovaccines show therapeutic effects by mimicking tumor cells and antigen presenting cells, Nat. Commun., 2019, 10(1), 3199 CrossRef PubMed
.
- W. L. Liu,
et al., Expandable immunotherapeutic nanoplatforms engineered from cytomembranes of hybrid cells derived from cancer and dendritic cells, Adv. Mater., 2019, 31(18), 1900499 CrossRef PubMed
.
- Q. Chen,
et al., A hybrid eukaryotic–prokaryotic nanoplatform with photothermal modality for enhanced antitumor vaccination, Adv. Mater., 2020, 32(16), 1908185 CrossRef CAS PubMed
.
- D. Wang,
et al., Erythrocyte–cancer hybrid membrane camouflaged hollow copper sulfide nanoparticles for prolonged circulation life and homotypic-targeting photothermal/chemotherapy of melanoma, ACS Nano, 2018, 12(6), 5241–5252 CrossRef CAS PubMed
.
- Y. Liao,
et al., Biomimetic hybrid membrane-based nanoplatforms: synthesis, properties and biomedical applications, Nanoscale Horiz., 2020, 5(9), 1293–1302 RSC
.
- L. Rao,
et al., Platelet–leukocyte hybrid membrane–coated immunomagnetic beads for highly efficient and highly specific isolation of circulating tumor cells, Adv. Funct. Mater., 2018, 28(34), 1803531 CrossRef
.
- X. Han,
et al., Red blood cell–derived nanoerythrosome for antigen delivery with enhanced cancer immunotherapy, Sci. Adv., 2019, 5(10), eaaw6870 CrossRef CAS PubMed
.
- D. Wang,
et al., Bacterial vesicle-cancer cell hybrid membrane-coated nanoparticles for tumor specific immune activation and photothermal therapy, ACS Appl. Mater. Interfaces, 2020, 12(37), 41138–41147 CrossRef CAS PubMed
.
- L. L. Bu,
et al., Cancer stem cell–platelet hybrid membrane–coated magnetic nanoparticles for enhanced photothermal therapy of head and neck squamous cell carcinoma, Adv. Funct. Mater., 2019, 29(10), 1807733 CrossRef
.
- Y. Liu,
et al., Erythrocyte–platelet hybrid membranes coating polypyrrol nanoparticles for enhanced delivery and photothermal therapy, J. Mater. Chem. B, 2018, 6(43), 7033–7041 RSC
.
- Q. Jiang,
et al., Erythrocyte-cancer hybrid membrane-camouflaged melanin nanoparticles for enhancing photothermal therapy efficacy in tumors, Biomaterials, 2019, 192, 292–308 CrossRef CAS PubMed
.
- B. Ji,
et al., Hybrid membrane camouflaged copper sulfide nanoparticles for photothermal-chemotherapy of hepatocellular carcinoma, Acta Biomater., 2020, 111, 363–372 CrossRef CAS PubMed
.
- X. Ren,
et al., Red blood cell membrane camouflaged magnetic nanoclusters for imaging-guided photothermal therapy, Biomaterials, 2016, 92, 13–24 CrossRef CAS PubMed
.
- S. Ye,
et al., Light/pH-triggered biomimetic red blood cell membranes camouflaged small molecular drug assemblies for imaging-guided combinational chemo-photothermal therapy, ACS Appl. Mater. Interfaces, 2019, 11(17), 15262–15275 CrossRef CAS PubMed
.
- D. Zhang,
et al., Extracellular vesicles based self-grown gold nanopopcorn for combinatorial chemo-photothermal therapy, Biomaterials, 2019, 197, 220–228 CrossRef CAS PubMed
.
- J. Wang,
et al., Designer exosomes for active targeted chemo–photothermal synergistic tumor therapy, Adv. Funct. Mater., 2018, 28(18), 1707360 CrossRef
.
- Q.-F. Meng,
et al., Macrophage membrane-coated iron oxide nanoparticles for enhanced photothermal tumor therapy, Nanotechnology, 2018, 29(13), 134004 CrossRef PubMed
.
- X. Yang,
et al., Biomimetic Aggregation–Induced Emission Nanodots with Hitchhiking Function for T Cell–Mediated Cancer Targeting and NIR–II Fluorescence–Guided Mild–Temperature Photothermal Therapy, Adv. Funct. Mater., 2022, 2206346 CrossRef CAS
.
- H. Ye,
et al., Bioinspired nanoplatelets for chemo-photothermal therapy of breast cancer metastasis inhibition, Biomaterials, 2019, 206, 1–12 CrossRef CAS PubMed
.
- L. Wu,
et al., Platelet membrane-coated nanoparticles for targeted drug delivery and local chemo-photothermal therapy of orthotopic hepatocellular carcinoma, J. Mater. Chem. B, 2020, 8(21), 4648–4659 RSC
.
- L. Rao,
et al., Antitumor platelet–mimicking magnetic nanoparticles, Adv. Funct. Mater., 2017, 27(9), 1604774 CrossRef
.
- Y. Cao,
et al., Engineered exosome-mediated near-infrared-II region V2C quantum dot delivery for nucleus-target low-temperature photothermal therapy, ACS Nano, 2019, 13(2), 1499–1510 CAS
.
- X. Mu,
et al., siRNA delivery with stem cell membrane-coated magnetic nanoparticles for imaging-guided photothermal therapy and gene therapy, ACS Biomater. Sci. Eng., 2018, 4(11), 3895–3905 CrossRef CAS PubMed
.
- S. Kim,
et al., Extracellular vesicles with high dual drug loading for safe and efficient combination chemo-phototherapy, ACS Appl. Mater. Interfaces, 2022, 10(11), 2817–2830 CAS
.
- C. Luo,
et al., Biomimetic carriers based on giant membrane vesicles for targeted drug delivery and photodynamic/photothermal synergistic therapy, ACS Appl. Mater. Interfaces, 2019, 11(47), 43811–43819 CrossRef CAS PubMed
.
- X. Liu, M. M. Jansman and L. J. B. S. Hosta-Rigau, Haemoglobin-loaded metal organic framework-based nanoparticles camouflaged with a red blood cell membrane as potential oxygen delivery systems, Biomater. Sci., 2020, 8(21), 5859–5873 RSC
.
- F. Castro,
et al., Advances on erythrocyte-mimicking nanovehicles to overcome barriers in biological microenvironments, Adv. Drug Delivery Rev., 2021, 170, 312–339 CrossRef CAS PubMed
.
- A. Bidkar, P. Sanpui and S. S. Ghosh, Transferrin-conjugated red blood cell membrane-coated poly (lactic-co-glycolic acid) nanoparticles for the delivery of doxorubicin and methylene blue, ACS Appl. Nano Mater., 2020, 3(4), 3807–3819 CrossRef CAS
.
- X. Han, C. Wang and Z. J. B. c. Liu, Red blood cells as smart delivery systems, Bioconjugate Chem., 2018, 29(4), 852–860 CrossRef CAS PubMed
.
- X. Han,
et al., Red blood cell–derived nanoerythrosome for antigen delivery with enhanced cancer immunotherapy, Sci. Adv., 2019, 5(10), eaaw6870 CrossRef CAS PubMed
.
- S. Hossen,
et al., Smart nanocarrier-based drug delivery systems for cancer therapy and toxicity studies: A review, J. Adv. Res., 2019, 15, 1–18 CrossRef CAS PubMed
.
- Y. Chen,
et al., Synergistic chemo-photodynamic therapy mediated by light-activated ROS-degradable nanocarriers, J. Mater. Chem. B, 2019, 7(3), 460–468 RSC
.
- A. Bidkar, P. Sanpui and S. S. Ghosh, Transferrin-conjugated red blood cell membrane-coated poly (lactic-co-glycolic acid) nanoparticles for the delivery of doxorubicin and methylene blue, ACS Appl. Nano Mater., 2020, 3(4), 3807–3819 CrossRef CAS
.
- S.-p. Li,
et al., Exosomal cargo-loading and synthetic exosome-mimics as potential therapeutic tools, Acta Pharmacol. Sin., 2018, 39(4), 542–551 CrossRef CAS PubMed
.
- H. Qi,
et al., Blood exosomes endowed with magnetic and targeting properties for cancer therapy, ACS Nano, 2016, 10(3), 3323–3333 CrossRef CAS PubMed
.
- G. Kibria,
et al., Exosomes as a drug delivery system in cancer therapy: potential and challenges, Mol. Pharmaceutics, 2018, 15(9), 3625–3633 CrossRef CAS PubMed
.
- S.-Y. Li,
et al., Cancer cell membrane-coated biomimetic platform for tumor targeted photodynamic therapy and hypoxia-amplified bioreductive therapy, Biomaterials, 2017, 142, 149–161 CrossRef CAS PubMed
.
- S.-Y. Li,
et al., Cancer cell membrane camouflaged cascade bioreactor for cancer targeted starvation and photodynamic therapy, ACS Nano, 2017, 11(7), 7006–7018 CrossRef CAS PubMed
.
- H.-R. Jia,
et al., Plasma membrane activatable polymeric nanotheranostics with self-enhanced light-triggered photosensitizer cellular influx for photodynamic cancer therapy, J. Controlled Release, 2017, 255, 231–241 CrossRef CAS PubMed
.
- H.-R. Jia,
et al., Plasma membrane-anchorable photosensitizing nanomicelles for lipid raft-responsive and light-controllable intracellular drug delivery, J. Controlled Release, 2018, 286, 103–113 CrossRef CAS PubMed
.
- S. Y. Li,
et al., A Versatile Plasma Membrane Engineered Cell Vehicle for Contact–Cell–Enhanced Photodynamic Therapy, Theranostic, 2017, 27(12), 1604916 Search PubMed
.
- H. Cheng,
et al., Mitochondria and plasma membrane dual-targeted chimeric peptide for single-agent synergistic photodynamic therapy, Biomaterials, 2019, 188, 1–11 CrossRef CAS PubMed
.
- L. Pan, J. Liu and J. J. C. s. r. Shi, Cancer cell nucleus-targeting nanocomposites for advanced tumor therapeutics, Chem. Soc. Rev., 2018, 47(18), 6930–6946 RSC
.
- K. Han,
et al., Acidity–triggered tumor–targeted chimeric peptide for enhanced intra–nuclear photodynamic therapy, Front. Chem., 2016, 26(24), 4351–4361 CAS
.
- L. Rong,
et al., Biomedical applications of functional peptides in nano-systems, Mater. Today Chem., 2018, 9, 91–102 CrossRef CAS
.
- Y.-X. Zhu,
et al., Development of a light-controlled nanoplatform for direct nuclear delivery of molecular and nanoscale materials, J. Am. Chem. Soc., 2018, 140(11), 4062–4070 CrossRef CAS PubMed
.
- H. Cheng,
et al., Chimeric peptide engineered exosomes for dual-stage light guided plasma membrane and nucleus targeted photodynamic therapy, Biomaterials, 2019, 211, 14–24 CrossRef CAS PubMed
.
- Q. Pei,
et al., Light-activatable red blood cell membrane-camouflaged dimeric prodrug nanoparticles for synergistic photodynamic/chemotherapy, ACS Nano, 2018, 12(2), 1630–1641 CrossRef CAS PubMed
.
- W. Li,
et al., Targeting photodynamic and photothermal therapy to the endoplasmic reticulum enhances immunogenic cancer cell death, Nat. Commun., 2019, 10(1), 1–16 CrossRef PubMed
.
- D. Xia,
et al., Overcoming hypoxia by multistage nanoparticle delivery system to inhibit mitochondrial respiration for photodynamic therapy, Adv. Funct. Mater., 2019, 29(13), 1807294 CrossRef
.
- Z. Yang,
et al., Defeating relapsed and refractory malignancies through a nano-enabled mitochondria-mediated respiratory inhibition and damage pathway, Biomaterials, 2020, 229, 119580 CrossRef CAS PubMed
.
- F. Wang,
et al., Triple–Layered Metal–Organic Framework Hybrid for Tandem Response–Driven Enhanced Chemotherapy, Chem. – Asian J., 2021, 16(15), 2068–2074 CrossRef CAS PubMed
.
- Y. Hong,
et al., Photoswitchable AIE nanoprobe for lysosomal hydrogen sulfide detection and reversible dual-color imaging, Sens. Actuators, B, 2018, 272, 340–347 CrossRef CAS
.
- D. Wang and B. Z. Tang, Aggregation-induced emission luminogens for activity-based
sensing, Acc. Chem. Res., 2019, 52(9), 2559–2570 CrossRef CAS PubMed
.
- Y. Gao,
et al., A dual–functional photosensitizer for ultraefficient photodynamic therapy and synchronous anticancer efficacy monitoring, Adv. Funct. Mater., 2019, 29(32), 1902673 CrossRef
.
- Y. Li,
et al., Boosting the photodynamic therapy efficiency by using stimuli-responsive and AIE-featured nanoparticles, Biomaterials, 2020, 232, 119749 CrossRef CAS PubMed
.
- L. Shi,
et al., Hybrid nanospheres to overcome hypoxia and intrinsic oxidative resistance for enhanced photodynamic therapy, ACS Nano, 2020, 14(2), 2183–2190 CrossRef CAS PubMed
.
- J.-H. Qin,
et al., Ionic liquid induced highly dense assembly of porphyrin in MOF nanosheets for photodynamic therapy, Dalton Trans., 2020, 49(48), 17772–17778 RSC
.
- D. Yang,
et al., Progress, opportunity, and perspective on exosome isolation-efforts for efficient exosome-based theranostics, Theranostics, 2020, 10(8), 3684 CrossRef CAS PubMed
.
- A. Hoshino,
et al., Tumour exosome integrins determine organotropic metastasis, Nature, 2015, 527(7578), 329–335 CrossRef CAS PubMed
.
- I. Wortzel,
et al., Exosome-mediated metastasis: communication from a distance, Dev. Cell, 2019, 49(3), 347–360 CrossRef CAS PubMed
.
- Y. Yang,
et al., Virus–mimetic fusogenic exosomes for direct delivery of integral membrane proteins to target cell membranes, Adv. Mater., 2017, 29(13), 1605604 CrossRef PubMed
.
- E. V. Batrakova and M. S. Kim, Using exosomes, naturally-equipped nanocarriers, for drug delivery, J. Controlled Release, 2015, 219, 396–405 CrossRef CAS PubMed
.
- W. Jiang,
et al., Remodeling tumor vasculature to enhance delivery of intermediate-sized nanoparticles, ACS Nano, 2015, 9(9), 8689–8696 CrossRef CAS PubMed
.
- D. Zhu,
et al., Tumor–Exocytosed Exosome/Aggregation–Induced Emission Luminogen Hybrid Nanovesicles Facilitate Efficient Tumor Penetration and Photodynamic Therapy, Angew. Chem., 2020, 132(33), 13940–13947 CrossRef
.
- Y. Jang,
et al., Exosome-based photoacoustic imaging guided photodynamic and immunotherapy for the treatment of pancreatic cancer, J. Controlled Release, 2021, 330, 293–304 CrossRef CAS PubMed
.
- K. Aubertin,
et al., Massive release of extracellular vesicles from cancer cells after photodynamic treatment or chemotherapy, Sci. Rep., 2016, 6(1), 1–11 CrossRef PubMed
.
- C. Gao,
et al., Stem-cell-membrane camouflaging on near-infrared photoactivated upconversion nanoarchitectures for in vivo remote-controlled photodynamic therapy, ACS Appl. Mater. Interfaces, 2016, 8(50), 34252–34260 CrossRef CAS PubMed
.
- J. Feng,
et al., Stem cell membrane–camouflaged bioinspired nanoparticles for targeted photodynamic therapy of lung cancer, J. Nanopart. Res., 2020, 22(7), 1–11 CrossRef
.
- A. Pinto,
et al., Immune reprogramming precision photodynamic therapy of peritoneal metastasis by scalable stem-cell-derived extracellular vesicles, ACS Nano, 2021, 15(2), 3251–3263 CrossRef CAS PubMed
.
- D. Dehaini,
et al., Erythrocyte–platelet hybrid membrane coating for enhanced nanoparticle functionalization, Adv. Mater., 2017, 29(16), 1606209 CrossRef PubMed
.
- O. C. J. N. Farokhzad, Platelet mimicry, Nature, 2015, 526(7571), 47–48 CrossRef CAS PubMed
.
- Q. Hu,
et al., Engineering platelet-mimicking drug delivery vehicles, Front. Chem. Sci. Eng., 2017, 11(4), 624–632 CrossRef
.
- Q. Hu,
et al., Anticancer platelet–mimicking nanovehicles, Adv. Mater., 2015, 27(44), 7043–7050 CrossRef CAS PubMed
.
- R. Baskaran, J. Lee and S.-G. J. B. r. Yang, Clinical development of photodynamic agents and therapeutic applications, Biomater. Res., 2018, 22(1), 1–8 CrossRef PubMed
.
- C. Furlan, J. A. Berenbeim and C. E. J. M. Dessent, Photoproducts of the Photodynamic Therapy Agent Verteporfin Identified via Laser Interfaced Mass Spectrometry, Molecules, 2020, 25(22), 5280 CrossRef CAS PubMed
.
- D. K. Deda and K. Araki, Nanotechnology, Light and chemical action: an effective combination to kill cancer cells, J. Braz. Chem. Soc., 2015, 26, 2448–2470 CAS
.
- D. Dehaini,
et al., Erythrocyte–platelet hybrid membrane coating for enhanced nanoparticle functionalization, Adv. Mater., 2017, 29(16), 1606209 CrossRef PubMed
.
- L. Xu,
et al., Platelet membrane coating coupled with solar irradiation endows a photodynamic nanosystem with both improved antitumor efficacy and undetectable skin damage, Biomaterials, 2018, 159, 59–67 CrossRef CAS PubMed
.
- R. J. Bose,
et al., Cell membrane-coated nanocarriers: the emerging targeted delivery system for cancer theranostics, Drug Discovery Today, 2018, 23(4), 891–899 CrossRef CAS PubMed
.
- Y. Zhao,
et al., Hybrid membrane-coated biomimetic nanoparticles (HM@ BNPs): a multifunctional nanomaterial for biomedical applications, Biomacromolecules, 2021, 22(8), 3149–3167 CrossRef CAS PubMed
.
- Q. Xie,
et al., Hybrid-cell membrane-coated nanocomplex-loaded chikusetsusaponin IVa methyl ester for a combinational therapy against breast cancer assisted by Ce6, Biomater. Sci., 2021, 9(8), 2991–3004 RSC
.
- T. Zhang,
et al., Leukocyte/platelet hybrid membrane-camouflaged dendritic large pore mesoporous silica nanoparticles co-loaded with photo/chemotherapeutic agents for triple negative breast cancer combination treatment, Bioact. Mater., 2021, 6(11), 3865–3878 CAS
.
- X. Xu,
et al., A Biomimetic Aggregation–Induced Emission Photosensitizer with Antigen–Presenting and Hitchhiking Function for Lipid Droplet Targeted Photodynamic Immunotherapy, Adv. Mater., 2021, 33(33), 2102322 CrossRef CAS PubMed
.
- L. Xu,
et al., Platelet membrane coating coupled with solar irradiation endows a photodynamic nanosystem with both improved antitumor efficacy and undetectable skin damage, Biomaterials, 2018, 159, 59–67 CrossRef CAS PubMed
.
- H. Zuo,
et al., Platelet-mimicking nanoparticles co-loaded with W18O49 and metformin alleviate tumor hypoxia for enhanced photodynamic therapy and photothermal therapy, Acta Biomater., 2018, 80, 296–307 CrossRef CAS PubMed
.
- S. Pan,
et al., Passion fruit-like exosome-PMA/Au-BSA@ Ce6 nanovehicles for real-time fluorescence imaging and enhanced targeted photodynamic therapy with deep penetration and superior retention behavior in tumor, Biomaterials, 2020, 230, 119606 CrossRef CAS PubMed
.
- D. D. Taylor and S. J. M. Shah, Methods of isolating extracellular vesicles impact down-stream analyses of their cargoes, Methods, 2015, 87, 3–10 CrossRef CAS PubMed
.
- T. Lener,
et al., Applying extracellular vesicles based therapeutics in clinical trials–an ISEV position paper, J. Extracell. Vesicles, 2015, 4(1), 30087 CrossRef PubMed
.
- F. M. Lang,
et al., Mesenchymal stem cells as natural biofactories for exosomes carrying miR-124a in the treatment of gliomas, Neuro-Oncology, 2018, 20(3), 380–390 CrossRef CAS PubMed
.
- D. d. Yu,
et al., Exosomes in development, metastasis and drug resistance of breast cancer, Cancer Sci., 2015, 106(8), 959–964 CrossRef CAS PubMed
.
- P. Akuma, O. D. Okagu and C. C. Udenigwe, Naturally occurring exosome vesicles as potential delivery vehicle for bioactive compounds, Front. Sustain. Food Syst., 2019, 3, 23 CrossRef
.
- W. Meng,
et al., Prospects and challenges of extracellular vesicle-based drug delivery system: Considering cell source, Drug Delivery, 2020, 27(1), 585–598 CrossRef CAS PubMed
.
- S. Stremersch,
et al., Comparing exosome-like vesicles with liposomes for the functional cellular delivery of small RNAs, J. Controlled Release, 2016, 232, 51–61 CrossRef CAS PubMed
.
- D. Rufino-Ramos,
et al., Extracellular vesicles: Novel promising delivery systems for therapy of brain diseases, J. Controlled Release, 2017, 262, 247–258 CrossRef CAS PubMed
.
- F. Mehryab,
et al., Exosomes as a next-generation drug delivery system: an update on drug loading approaches, characterization, and clinical application challenges, Acta Biomater., 2020, 113, 42–62 CrossRef CAS PubMed
.
- E. Blanco, H. Shen and M. J. N. b. Ferrari, Principles of nanoparticle design for overcoming biological barriers to drug delivery, Nat. Biotechnol., 2015, 33(9), 941–951 CrossRef CAS PubMed
.
- M. J. Mitchell, R. K. Jain and R. Langer, Engineering and physical sciences in oncology: challenges and opportunities, Nat. Rev. Cancer, 2017, 17(11), 659–675 CrossRef CAS PubMed
.
- M. Srinivasarao, C. V. Galliford and P. S. Low, Principles in the design of ligand-targeted cancer therapeutics and imaging agents, Nat. Rev. Drug Discovery, 2015, 14(3), 203–219 CrossRef CAS PubMed
.
- J. Shi,
et al., Cancer nanomedicine: progress, challenges and opportunities, Nat. Rev. Cancer, 2017, 17(1), 20–37 CrossRef CAS PubMed
.
|
This journal is © The Royal Society of Chemistry 2024 |