DOI:
10.1039/D3AY01615C
(Paper)
Anal. Methods, 2024,
16, 51-61
PER-CRISPR/Cas14a system-based electrochemical biosensor for the detection of ctDNA EGFR L858R†
Received
13th September 2023
, Accepted 21st November 2023
First published on 7th December 2023
Abstract
The detection of epidermal growth factor receptor (EGFR) mutation L858R in circulating tumor DNA (ctDNA) is beneficial for the clinical diagnosis and personalized therapy of non-small cell lung cancer (NSCLC). Herein, for the first time, the combination of the primer exchange reaction (PER) and clustered regularly interspaced short palindromic repeats (CRISPR) and its associated nucleases (Cas) 14a was used in electrochemical biosensor construction for the detection of ctDNA EGFR L858R. EGFR L858R, as the target, induced the isothermal amplification of the PER reaction, and then the CRISPR/Cas14a system was activated; subsequently, the substrate ssDNA-MB was cleaved and the electron on the surface of the gold electrode transferred, resulting in the fluctuation of the electrochemical redox signal on the electrode surface, whereas the electrochemical signal will be stable when EGFR L858R is absent. Therefore, the concentration of EGFR L858R can be quantified by electrochemical signal analysis. The low detection limit is 0.34 fM and the dynamic detection range is from 1 fM to 1 μM in this work. The PER-CRISPR/Cas14a electrochemical biosensor greatly improved the analytical sensitivity. In addition, this platform also exhibited excellent specificity, reproducibility, stability and good recovery. This study provides an efficient and novel strategy for the detection of ctDNA EGFR L858R, which has great potential for application in the diagnosis and treatment of NSCLC.
Introduction
As one of the most common liquid biopsy tumor biomarkers, circulating tumor DNA (ctDNA) is a fragment of DNA that is released into the human blood system from tumor tissues and can carry the tumor's unique genes.1,2 These ctDNA, distinct from the normal genome, often contain gene mutations,3 which can truly reflect the frequency of gene mutation profiles in the solid tumor tissues and serve as an important monitoring indicator for both evaluation of the treatment efficacy and judgment of the prognosis during the post-treatment clinical follow-up.4,5 Epidermal growth factor receptor (EGFR) is a protein tyrosine kinase and more than 80% of the EGFR mutations are detected in exons 19–21. Furthermore, 41% of the mutations in exon 21 are amino acid substitutions (EGFR L858R).6,7 EGFR L858R is one of the most studied ctDNA associated with gene mutations.8 Mutations of EGFR at the L858R locus are commonly observed in non-small cell lung cancer (NSCLC) and are associated with the development of NSCLC. EGFR L858R has gradually become an important target in personalized treatment of NSCLC.9 Hence, the detection of EGFR L858R is significantly valuable both in the clinical diagnosis and in the treatment of NSCLC.10,11
Most of the standard technologies are available for analyzing gene mutations, including next-generation sequencing (NGS), quantitative polymerase chain reaction (qPCR), and digital polymerase chain reaction (dPCR).12–14 However, these traditional techniques have several drawbacks, such as high cost, long processing time, cumbersome operation and low sensitivity, which can significantly restrict their application. Therefore, there is an urgent need for a novel simple, efficient and highly sensitive detection technique to fulfill the requirements of ctDNA EGFR L858R detection for possible future application in clinical practice. To solve the above-described problems, some emerging and neoteric technologies have been developed in recent years, such as fluorescence,15,16 electrochemistry,17–19 electrochemiluminescence,20 photoelectric chemistry,21 and surface plasma technology.22 Of these, an electrochemical biosensor is considered as an efficient detection method with low cost, high sensitivity, fast signal readout and a large concentration response range.23 As a favorable technology, the electrochemical detection technique has been popularly used to detect and quantify different analytes, and has emerged as one of the most widely used types of biosensor.24,25
A highly efficient and simple amplification method is crucial for the detection of trace amounts of ctDNA. As a robust nucleic acid amplification technique, primer exchange reaction (PER) has attracted widespread attention due to its particular type of single-stranded DNA (ssDNA) synthesis via primer extension. Briefly, the primer can achieve autonomous extension on the hairpin template under the action of Bst DNA polymerase, resulting in forming long ssDNA containing countless repeats.26 Because of the simple raw material design, high specificity, stable reaction and mild reaction conditions, PER has been employed in the construction of various high-performance biosensors.27–30
Clustered regularly interspaced short palindromic repeats (CRISPR) and the associated nucleases (Cas) initially evolved from the adaptive immune system of natural archaea and bacteria.31 This system consists of Cas protein and guide RNA, whose main function is to effectively guide Cas protein to its target site and then degrade the foreign nucleic acids.32,33 In the CRISPR/Cas family, CRISPR/Cas12a is a superstar for application in biosensors. It can be used in the detection of nucleic acids coupled with other technologies, such as hybridization chain reaction,34 inductively coupled plasma mass spectrometry35 and strand displacement/hybridization chain reaction.36 CRISPR/Cas14a, as an endonuclease, has been used for the construction of an electrochemical biosensor,37–39 which displayed high specificity, sensitivity and cleavage efficiency, revealing enormous potential in nucleic acid detection.40 Although the molecular weight of Cas14a protein is relatively smaller (62 kDa) than other Cas proteins,41 Cas14a can bind to the target ssDNA and activate its ssDNA trans-cleavage activity, similar to Cas12a. Several recent studies have found that Cas14a can distinguish single nucleotide polymorphisms well, and the universal bacterial diagnostic method developed based on the CRISPR/Cas14a system can potentially differentiate six bacterial strains with a detection limit of 1 aM.42 Therefore, it is valuable to vigorously develop DNA electrochemical biosensors based on the CRISPR/Cas14a system. However, to date, no reports have been publishing showing that ctDNA detection by electrochemical biosensor based on PER combined with CRISPR/Cas14a system is feasible and effective.
In the present study, we developed a novel electrochemical biosensor platform combining PER with CRISPR/Cas14a for the specific and sensitive detection of ctDNA EGFR L858R. In the presence of EGFR L858R, hairpins H1 and H2 are opened sequentially, and then the PER proceeds under the action of Bst polymerase. As a result of the PER, a long nucleic acid chain composed of repeated Cas14a recognition signals is produced. These repetitive sequences can bind to many crRNAs and activate Cas14a, which triggers non-specific cleavage of ssDNA-MB, which is fixed on the surface of the gold electrode by disulfide bonds. Ultimately, the electrochemical redox signal changes and the results are recorded by an electrochemical workstation. Our current results indicate that the detection platform has excellent sensitivity, specificity, reproducibility, and stability, with good recovery. Taken together, the strategy proposed in this study provides an efficient and novel platform for the detection of ctDNA EGFR L858R, supporting its potential application for future clinical sample analysis.
Experimental
Materials and reagents
Acrylamide, N,N′-methylenebisacrylamide, tris(hydroxymethyl) aminoethane, tris(2-carboxyethyl) phosphine (TCEP), sodium phosphate monobasic dihydrate, sodium phosphate dibasic dodecahydrate, and sodium chloride (NaCl) were purchased from Aladdin Bio-Chem Technology Co., Ltd. (Shanghai, China). Ammonium persulfate, formaldehyde, K3Fe(CN)6, N,N,N′,N′-tetramethylethylenediamine (TEMED), and K4Fe(CN)6 were purchased from Macklin Biochemical Co., Ltd. (Shanghai, China). Hydrochloric acid (HCl) was purchased from Lingfeng Chemical Reagents Co., Ltd. (Shanghai, China). 2-[4-(2-Hydroxyethyl) piperazin-1-yl]ethanesulfonic acid (HEPES) was purchased from Yien Chemical Technology Co., Ltd. (Shanghai, China).
The tracrRNA and crRNA sequences were purchased from BiOligo Biotechnology Co., Ltd. (Shanghai, China), and the other sequences in this work were bought from Sangon Biotech Co., Ltd. (Shanghai, China). Cas14a protein was obtained from Magigen Biotechnology. Co. Ltd. (Guangzhou, China). All the sequences in this work are shown in Table S1.†
The DNA immobilization buffer used in this work consisted of 10 mM TCEP in 20 mM HEPES (pH 7.4) and 100 mM NaNO3. The DNA hybridization buffer comprised 50 mM Mg(NO3)2·6H2O in 20 mM HEPES (pH 7.4) and 100 mM NaNO3. The buffer used for the fluorescence experiment consisted of 10 mM MgCl2 in 20 mM Tris–HCl (pH 8.5) and 25 mM NaCl. All the relevant buffers were prepared by DEPC water.
Apparatus
The measurements of electrochemical impedance spectroscopy (EIS) and cyclic voltammetry (CV) were obtained with an Autolab PGSTAT 302 N electrochemical workstation (Metrohm Technology Co., Ltd., Herisau, Switzerland). The alternating current voltammetry (ACV) measurements were recorded with a CHI 660 E electrochemical workstation (Shanghai Chenhua Instruments Co., Ltd., Shanghai, China). The polyacrylamide gel electrophoresis experiments were performed using a DYCZ-25 D electrophoresis apparatus (Beijing 61 Biological Technology Co., Ltd., Beijing, China) and imaged on a Tanon 2500 BR Multifunctional gel imaging analyzer (Tanon, Shanghai, China). The results of the fluorescence experiments were measured using an FS5 fluorescence spectrofluorometer (Edinburgh Instruments Ltd., UK).
PER amplification
Prior to amplification, hairpin H1 and H2 were annealed in DNA hybridization buffer at 95 °C for 5 min and then cooled to room temperature. Subsequently, the target sequence, hairpin H1 (1 μM), H2 (1 μM), dNTP (1 mM, N = A, T, C), the cleaner sequence (1 μM), Bst polymerase (0.1 U), and MgSO4 (1 mM) were mixed in an EP tube and incubated at 37 °C 2 h. Finally, the PER reactant was heated to 80 °C for 5 min to terminate the reaction before mixing with CRISPR/Cas14a.
CRISPR/Cas14a reaction
Cas14a (1 μM) was preincubated with the tracrRNA:crRNA mixture (1 μM) in DNA hybridization buffer at 37 °C for half an hour, and then the PER reactant or the Cas14a recognition sequence (activator) was added. Fluorescent reporter probe (FAM-ssDNA-BHQ) (1 μM) was added as the substrate of this reaction. Thereafter, the mixture was shaken continuously at 37 °C for 1 h.
PER-CRISPR/Cas14a electrochemical biosensor construction
The gold electrodes (Φ = 2 mm) were polished with 0.3 μm and 0.05 μm alumina suspensions, and then they were cleaned through a series of oxidation and reduction cycles in four different solutions (0.5 M NaOH, 0.5 M H2SO4, 0.01 M KCl/0.1 M H2SO4 and 0.05 M H2SO4) in turn.
The cleaned gold electrode was modified with ssDNA-MB. The ssDNA-MB was diluted to 1 μM with the DNA immobilization buffer, treated with TCEP for 30 min, and then added on the surface of the gold electrode and left for 1 h at room temperature. Subsequently, the surface of the gold electrode was washed with HEPES solution and passivated with 1 mM MCH for 20 min. HEPES solution was used to rinse the electrodes after MCH incubation. After the modification of the gold electrode, 6 μL of the PER-CRISPR/Cas14a reactant was added on the surface of the gold electrode and incubated at 37 °C for 1 h. The electrochemical measurements were conducted after this reaction.
Fluorescence measurement
An FS5 fluorescence spectrofluorometer was used to measure the results of the CRISPR/Cas14a reaction. The emission spectrum was 510–600 nm and the results were recorded at an excitation wavelength of 490 nm. Furthermore, the slit width of the fluorescence spectrofluorometer was 3 nm.
Electrochemical measurements
The electrochemical measurements were performed using a three-electrode system. The working electrode, reference electrode and counter electrode were gold electrodes, Ag/AgCl (3 M KCl) and platinum wire, respectively. The CV experiments were carried out in a solution of 1 M KCl and 10 mM K3[Fe(CN)6]. The CV experimental voltage was −0.2 V to 0.7 V, and the scanning frequency was 0.1 V s−1. The EIS experiments were carried out in a solution of 1 M KCl and 10 mM K3[Fe(CN)6]/K4[Fe(CN)6]. The amplitude for the EIS was 0.01 V and the frequency range was 0.01 Hz to 1 × 105 Hz. The solution for the ACV test consisted of 0.1 M PB and 0.1 M NaNO3. The voltage for the ACV test was −0.5 V to 0 V, with an amplitude of 0.025 V and frequency of 100 Hz. The change rate of the current (ΔI%) was calculated with the formula ΔI% = (I0 − I)/I0, where I0 and I represent the current intensity in the absence or presence of the target, respectively.
Polyacrylamide gel electrophoresis (PAGE)
The electrophoresis was conducted with a 15% non-denaturing polyacrylamide gel at 100 V for 40 min. The gels were stained with silver staining for 20 min and imaged on a Tanon 2500 BR Multifunctional gel imaging analyzer.
Results and discussion
Principle of PER-CRISPR/Cas14a electrochemical biosensor
The principle of the PER-CRISPR/Cas14a electrochemical biosensor is shown in Scheme 1. The PER-CRISPR/Cas14a electrochemical biosensor consists of three parts: PER amplification, CRISPR/Cas14a reaction and electrochemical measurement. When the ctDNA EGFR L858R was present in the solution, the hybridization between the loop chain of the hairpin H1 and EGFR L858R enabled the stem chain of H1 to open. Subsequently, a complementary combination emerged between the stem chain of H1 and the protruding stem strand of hairpin H2. Under the action of Bst DNA polymerase, the stem chain of H1 was extended along the hairpin H2, producing double-stranded DNA (dsDNA). However, when the extension reached the end of the H2 stem strand, a termination site (C–G) blocked the continuation of the extension due to a lack of dGTP, and the extended product was spontaneously dissociated from H2 because of the inter-base forces and three branching migration interaction .43 This extended product could bind to the protruding stem chain of H2 again according to the sequence design of hairpin H2. The cycle of binding, extension and dissociation repeated and produced a long strip of sequence, which contained numerous repeats recognized by CRISPR/Cas14a. This process ceased only when the materials were exhausted. The repeats (ATCTCTTATT) were called activators in our work. The cleaner sequences were used to exhaust the potential dGTP in the solution to ensure the correct PER amplification. The CRISPR/Cas14a reaction involved Cas14a protein, tracrDNA, crRNA, and activator. When the activator was present, it could effectively bind to crRNA, and the trans-cleavage of Cas14a was activated. The ssDNA was used as the substrate of Cas14a. It could be cleaved indiscriminately by the activated Cas14a. The 3′ end of the ssDNA was modified with electro-active marker Methylene blue (MB) and the 5′ end of the ssDNA was fixed on the gold electrode surface by an Au–S bond. 6-Mercapto-1-hexanol (MCH) was used to block the non-specific sites on the gold electrode surface. Under the activity of Cas14a, the ssDNA was cleaved and MB was released, leading to the electron transfer on the surface of the gold electrode. Finally, the change of the electrochemical signal was recorded by an electrochemical workstation. In brief, when EGFR L858R was present, the PER reaction occurred and turned on the CRISPR/Cas14a system; subsequently, the ssDNA-MB was cleaved and the electron on the surface of the gold electrode transferred, and a fluctuating electrochemical signal was detected, whereas the electrochemical signal will be stable when EGFR L858R is absent. Therefore, the concentration of EGFR L858R could be quantified by electrochemical signal analysis. Compared to the traditional PER reaction, we added hairpin H1 to the PER reaction. The PER amplification will only be activated after the binding of the target and the hairpin H1. It has two advantages: (1) a longer detection sequence was allowed in this study, due to the large size of the loop chain of hairpin H1; and (2) the detection probes of different EGFR mutation sites could be constructed with the same hairpin H2 and reaction conditions; only hairpin H1 was changed. It will make the detection of EGFR mutation more convenient and consistent, and the screening of EGFR mutation sites can be achieved in one tube. Additionally, no literature has been reported on the combination of the CRISPR/Cas14a system and PER. Cas14a is not limited by protospacer adjacent motif (PAM) sites in the target sequences, and it can bind to the ssDNA target with higher recognition and fidelity,44 which improves the sensitivity and specificity of the detection of ctDNA EGFR L858R. At the same time, the sensitivity of this detection is also enhanced by the PER reaction. Thus, this method is sensitive and specific for ctDNA EGFR L858R detection.
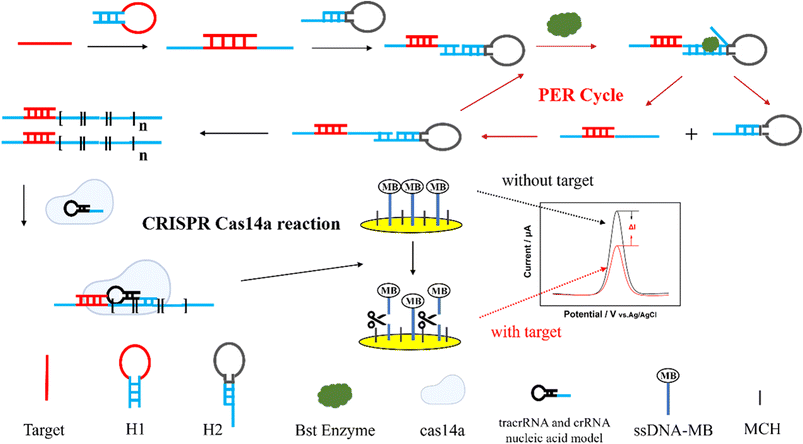 |
| Scheme 1 The schematic process of the PER-CRISPR/Cas14a electrochemical biosensor for the detection of ctDNA EGFR L858R. | |
Feasibility of PER
To confirm that the PER reaction was triggered by EGFR L858R, 15% PAGE electrophoresis was conducted. The results are shown in Fig. 1. Lanes 1, 2, 3, and 4 represent the four components that participate in the PER reaction (the target, hairpins H1 and H2 and the cleaner sequence). Their migration rates varied due to the base pair numbers. When the target EGFR L858R coexisted with hairpin H1, H1 was opened and they formed a new structure. The migration rate of this new structure was slower than the migration rates of EGFR L858R and H1, and there was some excess target and H1 in the solution, so three bands were observed in lane 5. Hairpins H1 and H2 did not interact with each other without the help of the target EGFR L858R, so in lane 6 there were only two bands: the bands of H1 and H2. When the target and hairpins H1 and H2 were all present, the PER reaction occurred and produced a lot of long nucleic acid chains containing different numbers of repeats recognized by Cas14a. Hence, we can see a series of gradient bands of high relative molecular mass in lane 7, and the excessive H1 and H2 were also found in this lane. The above results suggest the feasibility of the PER reaction in our work.
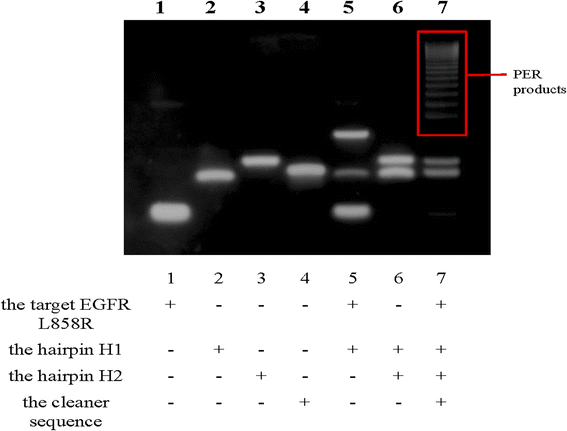 |
| Fig. 1 Gel electrophoresis image of the PER reaction. Lane 1, the target EGFR L858R; lane 2, hairpin H1; lane 3, hairpin H2; lane 4, the cleaner sequence; lane 5, EGFR L858R incubated with hairpin H1; lane 6, hairpin H1 incubated with hairpin H2; lane 7, EGFR L858R incubated with hairpins H1 and H2 and the cleaner sequence. | |
Feasibility of CRISPR/Cas14a reaction
The trans-cleavage activity of Cas14a is essential for the PER-CRISPR/Cas14a electrochemical biosensor. To verify the feasibility of the CRISPR/Cas14a reaction, a fluorescence spectrofluorometer was used. The ssDNA was modified with the fluorescent group FAM on the 5′ end and with the quenching group BHQ on the other end (FAM-ssDNA-BHQ), and FAM-ssDNA-BHQ was called the reporter in this part. When the activator is present, Cas14a will be activated to cleave the ssDNA sequence, and then the fluorescence signal will be enhanced with the increased distance between FAM and BHQ. No obvious characteristic fluorescence peaks were observed in the intact reporter that is not cleaved by Cas14a or in other components, such as crRNA-activator, tracrRNA:crRNA, Cas14a and the PER products (Fig. S1†). However there was a strong fluorescence characteristic peak when the components of the CRISPR/Cas14a reaction were all mixed together (the red curve in Fig. 2A). If one of the elements (reporter, crRNA-activator, tracrRNA:crRNA, Cas14a) was absent, the CRISPR/Cas14a reaction will not be turned on, resulting in no fluorescent signal being collected (the other color curves in Fig. 2A).
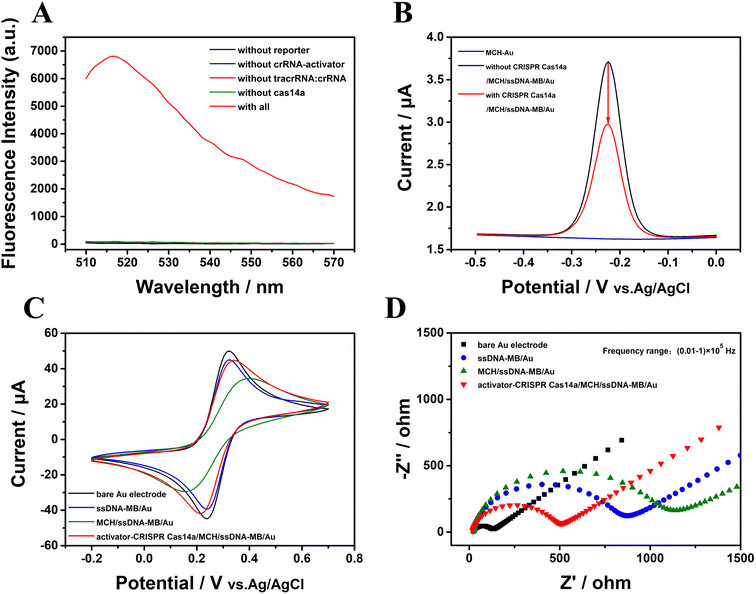 |
| Fig. 2 The verification of the feasibility of the CRISPR/Cas14a reaction. (A) The fluorescence spectra, (B) ACV results, (C) CV results, and (D) EIS results. | |
Electrochemical measurement techniques were also employed to verify the feasibility of the CRISPR/Cas14a reaction. ssDNA modified with MB was used as the reporter in this part. The ACV was used to estimate the current signal of the MB. The ACV response did not display any peak on the surface of the gold electrode modified with MCH (the blue curve in Fig. 2B); however, after the modification with ssDNA-MB, there was an obvious sharp high characteristic redox peak for MB (−0.3 V to −0.2 V) (the black curve in Fig. 2B). Interestingly, the peak of the current signal on the electrode decreased significantly after the CRISPR/Cas14a reaction occurred, caused by a rapid cleavage of the ssDNA-MB and the absence of the MB on the gold electrode (the red curve in Fig. 2B). The CV and EIS responses were also used to evaluate the feasibility of CRISPR/Cas14a reaction. The CV results in Fig. 2C show that a sharp symmetric redox peak (the black curve in Fig. 2C) was observed on the surface of the bare gold electrode, indicating good electrochemical conductivity. After the fixing of the ssDNA-MB on the exposed gold electrode surface, the current decreased significantly (the blue curve in Fig. 2C), and was reduced further after the modification of MCH (the green curve in Fig. 2C). It may be the result of the failure of the redox of [Fe(CN)6]3−/4− on the surface of the gold electrode, caused by repulsion or the seal on the electrode. However, when the CRISPR/Cas14a reaction was applied, the current increased (the red curve in Fig. 2C) as a result of the cleavage of the ssDNA-MB, which is beneficial for [Fe(CN)6]3−/4− to reach the surface of the gold electrode. The EIS curve consists of two distinct parts. The half-circle reflects the electron transfer resistance and the linear part indicates the electron diffusion. In Fig. 2D, the EIS results demonstrate that the impedance of the bare gold electrode is small (the black curve in Fig. 2D), indicating the high electron transfer efficiency of the bare electrode. After the modification of the ssDNA-MB, the impedance value increased (the blue curve in Fig. 2D), as a result of the repulsion between the phosphate backbone of the negatively charged ssDNA and the negatively charged [Fe(CN)6]3−/4−, and the electron transfer was hindered on the electrode surface. The impedance value increased further (the green curve in Fig. 2D) when the unbound sites were blocked by MCH. When the CRISPR/Cas14a reaction was activated, the impedance value decreased (the red curve in Fig. 2D) because ssDNA-MB was cleaved and fell off the gold electrode surface. The above results suggest the successful assembly of ssDNA-MB on the surface of the gold electrode and the successful cleavage of the CRISPR/Cas14a reaction induced by the activator indicated the feasibility of the CRISPR/Cas14a reaction in this study.
Feasibility of PER-CRISPR/Cas14a electrochemical biosensor
The CRISPR/Cas14a reaction was combined with the PER reaction. The PER products were used as the activators in the CRISPR/Cas14a reaction. Fluorescence spectra were measured to investigate the feasibility of PER-CRISPR/Cas14a reaction. The results are shown in Fig. 3. A strong characteristic fluorescence peak was observed when the target EGFR L858R was present (the red curve in Fig. 3A), however, no fluorescence peak was shown when the target (the blue curve in Fig. 3A) or the other components (reporter, tracrRNA:crRNA, Cas14a) (the black, rose-red, green curves in Fig. 3A) were absent, suggesting the successful cleavage of the CRISPR/Cas14a reaction induced by the PER products. The feasibility of PER-CRISPR/Cas14a reaction was also confirmed with the results obtained by the measurements of the ACV, CV and EIS responses (Fig. 3B–D). The ACV results showed that, compared with the current in the reaction without EGFR L858R, tracrRNA:crRNA, Cas14a or all of them (the blue, grape, green and black curves in Fig. 3B), there was a significant decrease of the ACV peak current in the reaction of CRISPR/Cas14a coupled with the PER reaction (the red curve in Fig. 3B). The CV and the EIS responses also showed an increased current or a decreased impedance value in the reaction of CRISPR/Cas14a coupled with the PER reaction (Fig. 3C and D). The above results indicate the feasibility of the PER-CRISPR/Cas14a electrochemical biosensor.
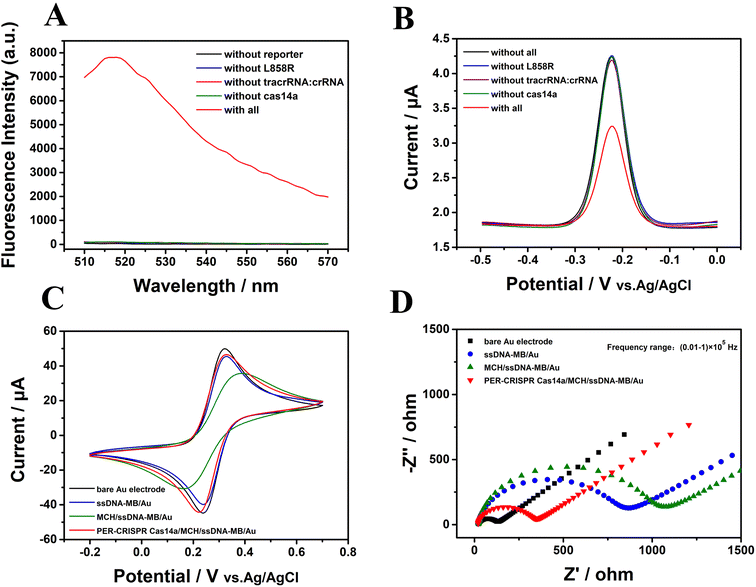 |
| Fig. 3 The feasibility verification of the CRISPR/Cas14a reaction coupled with the PER reaction. (A) Fluorescence spectra, (B) ACV results, (C) CV results, and (D) the EIS results. | |
Conditions optimization
The ssDNA-MB is an important link throughout this electrochemical biosensor platform. It was found that a too low concentration of ssDNA-MB could reduce the sensitivity of the platform, whereas a too high concentration could increase the steric hindrance and attenuate the efficiency of the platform. In this work, we found that the electrode surface signal was saturated when the concentration of ssDNA-MB was 1 μM (Fig. 4A). The incubation time was also important to the efficiency of this platform, and the appropriate incubation time was found to 1 h (Fig. 4B). Fig. 4C shows that the change rate of the current (ΔI%) reached its peak when the concentration of the hairpin H1 was 1 μM. ΔI% = (I0 − I)/I0, where I0 and I represent the current intensity in the absence or presence of the target, respectively. The concentration of tracrRNA:crRNA and the digestion time of CRISPR/Cas14a can directly affect the trans-cleavage efficiency. It was found that when the concentration of tracrRNA
:
crRNA was 1 μM
:
1 μM, ΔI% reached its peak (Fig. 4D). With the increase in the cutting reaction time of CRISPR/Cas14a, the cutting efficiency gradually increased. At 1 h, the cutting efficiency reached its peak and no longer increased, so 1 h was selected as the appropriate digestion time for the CRISPR/Cas14a reaction (Fig. 4E).
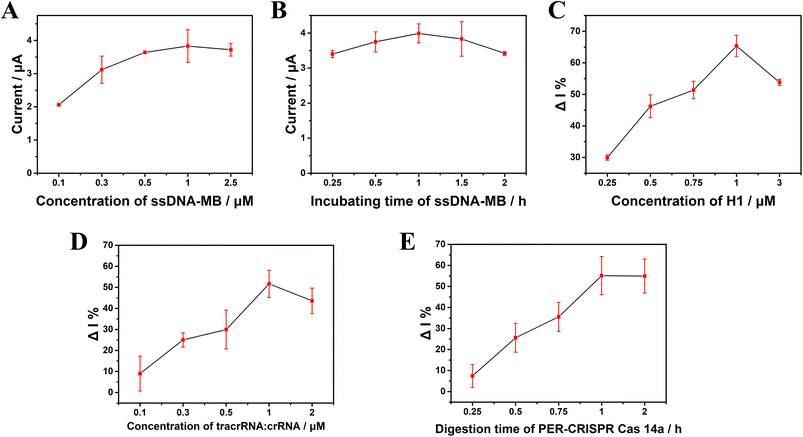 |
| Fig. 4 Optimization of the concentration of ssDNA-MB (A), the incubation time for ssDNA-MB (B), the concentration of hairpin H1 (C), the concentrations of tracrRNA:crRNA (D), and the digestion time for PER-CRISPR Cas14a (E). Error bars represent the standard deviation of three parallel experiments. | |
Analytical performance of the PER-CRISPR/Cas14a electrochemical biosensor
The concentration of EGFR L858R was detected under the optimized conditions. The ACV results are shown in Fig. 5A. The range of the concentration of EGFR L858R was from 1 fM to 1 μM. With the concentration of the target increased, the ACV peak current gradually decreased. The increased concentration of EGFR L858R induced more PER amplifier production, which ais recognized by CRISPR/Cas14a system, and then more cleavage of the ssDNA-MB on the surface of the gold electrode occurred, leading to a decrease in the current. Fig. 5B shows that there is a linear relationship between ΔI% and the logarithm of the concentration of the target (lg
C) in the range of 1 fM to 1 nM. This relationship can be described by the equation ΔI% = 9.84
lg
C + 9.06 (R2 = 0.984) (Fig. 5B). The limit of detection (LOD) is 0.34 fM in our work. The PER-CRISPR/Cas14a electrochemical biosensor has a wide detection range and a low LOD compared with previous studies of the detection of ctDNA by electrochemical methods (Table 1). This can be ascribed to the signal amplification of PER and the signal recognition of the CRISPR/Cas14a system. Compared with ref. 45, 46 and 48, there was no pre-amplification of nucleic acid in these three methods. The sensitivity of detection was improved by the use of the new materials. In ref. 49, a new efficient electrochemical tag was applied, which greatly enhanced the sensitivity of the electrochemical biosensor. The PER reaction and CRISPR/Cas14a system might make up for the deficiency of the sensitivity of MB in our study. This suggests that we can combine new pre-amplification reactions with new materials in the future to improve the sensitivity and efficiency of the detection. The difference between ref. 47 and our study is the order of the CRISPR/Cas system and the isothermal amplification reaction. It may depend on different detection targets. In our future work, the order of the different methods should also be considered in the establishment and optimization of the system. Although ref. 50 also had an isothermal amplification reaction, the pre-amplified products were signal molecules that were recognized by the target nucleic acid. However, in our study, the pre-amplified products were the target nucleic acid itself.
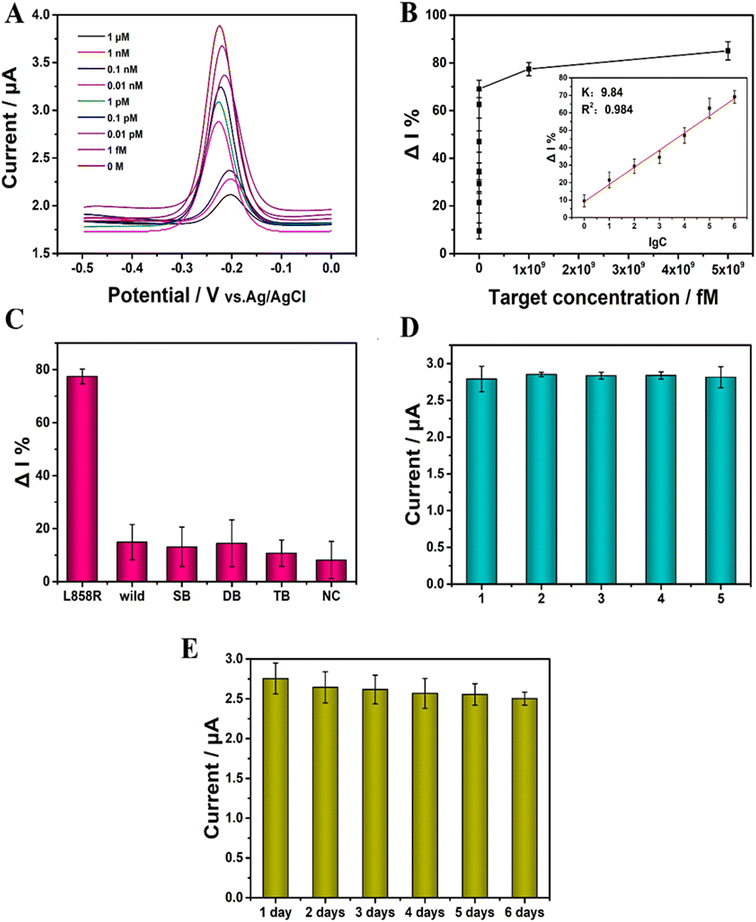 |
| Fig. 5 The performance of the PER-CRISPR/Cas14a electrochemical biosensor. (A) Measurement of the ACV response with different concentrations of EGFR L858R (0–1 μM). (B) Relationship between ΔI% and the concentration of EGFR L858R in the range of 0–5 μM. Inset: the linear relationship between ΔI% and the logarithm of the concentration of EGFR L858R in the range of 1 fM–1 nM. The linear equation is ΔI% = 9.84 lg C + 9.06 (R2 = 0.984). (C) The ΔI% values for EGFR L858R, the wild-type EGFR sequence (wild), the single-base-mismatched DNA sequence (SB), the double-base-mismatched DNA sequence (DB), the three-base-mismatched DNA sequence (TB) and the non-complementary DNA sequence (NC). All of them, the concentration is 1 μM. (D) Repeatability of the electrochemical sensors. (1, 2, 3, 4, and 5 represent five different electrochemical sensors constructed under identical conditions. Target concentration: 0.01 nM.) (E) Stability of the electrochemical sensors (target concentration: 0.01 nM). Error bars represent the standard deviation of three parallel experiments. | |
Table 1 Comparison with similar tests from previous studies
Detection method |
Analysis method |
Target (ctDNA) |
Detection range |
Detection limit |
Reference |
EC |
DPV |
EGFR L858R |
100 fM–100 nM |
7.65 fM |
45
|
EC |
Amperometric response |
EGFR L858R |
0.1 μM–3 μM |
120 nM |
46
|
EC |
DPV |
ctDNA EGFR |
0 pM–3 nM |
0.13 pM |
47
|
ECL |
ECL intensity |
Mutant KRAS |
1 fM–1 nM |
0.8 fM |
48
|
EC |
DPV |
ctDNA |
10 fM–10 nM |
1.6 fM |
49
|
EC |
DPV |
ctDNA PIK3CA E545K |
5 pM–0.5 nM |
3 pM |
50
|
EC
|
ACV
|
EGFR L858R
|
1 fM–1 μM
|
0.34 fM
|
This work
|
Specificity is crucial for the detection of ctDNA, especially in the detection of EGFR L858R. Five mismatch sequences (Table S2†) were used to investigate the specificity of this detection platform. The results showed that the ΔI% was significantly larger when the target was EGFR L858R than when the targets were the mismatch sequences (Fig. 5C). This suggests that the PER-CRISPR/Cas14a electrochemical biosensor platform has good specificity and can distinguish the target from the mismatch sequences well, even with a single base pair mismatch.
The relative standard deviation (RSD) was used to assess the reproducibility of the PER-CRISPR/Cas14a electrochemical biosensor platform. Five parallel electrochemical sensors were constructed under identical conditions, and the RSD was 3% (Fig. 5D), suggesting that the biosensor has good reproducibility.
The biosensor was stored at 4 °C for 6 days to investigate its stability. The ΔI% remained at 91% of the primary value after 6 days (Fig. 5E), indicating that the biosensor has good stability.
The feasibility of the PER-CRISPR/Cas14a electrochemical biosensor for application in human serum was also evaluated. Various concentrations of ctDNA EGFR L858R were added into five samples of human serum diluted 100-fold with PBS. The experiment was approved by the Ethics Committee of Nantong University Hospital. The ratio of the measured concentration of EGFR L858R to the added concentration is set as the recovery value. The recovery rate was between 94.61% and 115.19%, and the RSD was between 5.01% and 9.97% (Table 2). Serum samples from five NSCLC patients were chosen to detect the concentration of ctDNA EGFR L858R, and EGFR L858R was found in two of them. These results suggested that ctDNA EGFR L858R can be accurately detected in complex serum samples, implying that the developed biosensor is applicable in biological samples.
Table 2 The recovery and RSD of the PER-CRISPR/Cas14a electrochemical biosensor in serum samples spiked with various concentrations of EGFR L858R
Sample |
Spiked (fM) |
Measured (fM) |
Recovery (%) |
RSD (%) |
1 |
50.00 |
47.86 |
95.73 |
9.37 |
2 |
100.00 |
105.73 |
105.73 |
7.93 |
3 |
1000.00 |
1151.91 |
115.19 |
5.01 |
4 |
100.00 |
94.61 |
94.61 |
9.97 |
5 |
1000.00 |
1047.95 |
104.80 |
7.93 |
Conclusions
In conclusion, a specific and sensitive electrochemical biosensor platform based on PER and CRISPR/Cas14a was developed for the detection of ctDNA EGFR L858R. EGFR L858R, as the target, induced the isothermal amplification of the PER reaction, and then the CRISPR/Cas14a system was activated, resulting in the change of the electrochemical redox signal on the electrode surface. With an LOD of 0.34 fM and a dynamic detection range of 1 fM–μM, the PER-CRISPR/Cas14a electrochemical biosensor greatly improved the analytical sensitivity. In addition, this platform exhibited excellent specificity, reproducibility, and stability with good recovery, making it promising for the detection of ctDNA EGFR L858R in the clinical diagnosis and treatment of NSCLC.
Author contributions
Jing Qi: conceptualization; methodology; investigation; data curation; formal analysis; validation; writing–original draft preparation. Qianyi Qi: methodology; investigation. Yixuan Wu and Zhou Zhou: conducted experiments; analyzed data. Aiting Cai and Jinran Wu: conducted experiments; investigation. Bairong Chen: analyzed data. Qingxiang Wang: methodology; writing–review and editing. Lin Chen: conceptualization; methodology; funding acquisition; formal analysis; writing–review and editing. Feng Wang: supervision; funding acquisition; formal analysis; writing–review and editing. All authors read and approved the final manuscript.
Conflicts of interest
There are no conflicts to declare.
Acknowledgements
This work was financially supported by the National Natural Science Foundation of China (81873978 and 32250008), Key Project of Social Development in Jiangsu Province (BE2019691), Project of Jiangsu Commission of Health (Z2020011), and Postdoctoral Research Funding Project of Jiangsu Province (2021K012A).
References
- M. Nagasaka, M. H. Uddin, M. N. Al-Hallak, S. Rahman, S. Balasubramanian, A. Sukari and A. S. Azmi, Mol. Cancer, 2021, 20, 82 CrossRef.
- S. Jahr, H. Hentze, S. Englisch, D. Hardt, F. O. Fackelmayer, R. D. Hesch and R. Knippers, Cancer Res., 2001, 61, 1659–1665 Search PubMed.
- W. Li, J. B. Liu, L. K. Hou, F. Yu, J. Zhang, W. Wu, X. M. Tang, F. Sun, H. M. Lu, J. Deng, J. Bai, J. Li, C. Y. Wu, Q. L. Lin, Z. W. Lv, G. R. Wang, G. X. Jiang, Y. S. Ma and D. Fu, Mol. Cancer, 2022, 21, 25 CrossRef.
- J. C. Stadler, Y. Belloum, B. Deitert, M. Sementsov, I. Heidrich, C. Gebhardt, L. Keller and K. Pantel, Cancer Res., 2022, 82, 349–358 CrossRef PubMed.
- X. Wu, J. Li, A. Gassa, D. Buchner, H. Alakus, Q. Dong, N. Ren, M. Liu, M. Odenthal, D. Stippel, C. Bruns, Y. Zhao and R. Wahba, Int. J. Biol. Sci., 2020, 16, 1551–1562 CrossRef PubMed.
- J. Y. Yu, S. F. Yu, S. H. Wang, H. Bai, J. Zhao, T. T. An, J. C. Duan and J. Wang, Chin. J. Cancer, 2016, 35, 30 CrossRef PubMed.
- P. T. Harrison, S. Vyse and P. H. Huang, Semin. Cancer Biol., 2020, 61, 167–179 CrossRef CAS PubMed.
- X. Zhuang, C. Zhao, J. Li, C. Su, X. Chen, S. Ren, X. Li and C. Zhou, Cancer Med., 2019, 8, 2858–2866 CrossRef CAS.
- S. Lu, Y. Yu, Z. Li, R. Yu, X. Wu, H. Bao, Y. Ding, Y. W. Shao and H. Jian, J. Thorac. Oncol., 2019, 14, 732–736 CrossRef CAS PubMed.
- A. K. Krug, D. Enderle, C. Karlovich, T. Priewasser, S. Bentink, A. Spiel, K. Brinkmann, J. Emenegger, D. G. Grimm, E. Castellanos-Rizaldos, J. W. Goldman, L. V. Sequist, J. C. Soria, D. R. Camidge, S. M. Gadgeel, H. A. Wakelee, M. Raponi, M. Noerholm and J. Skog, Ann. Oncol., 2018, 29, 700–706 CrossRef CAS.
- S. Wang, J. Shi, Z. Ye, D. Dong, D. Yu, M. Zhou, Y. Liu, O. Gevaert, K. Wang, Y. Zhu, H. Zhou, Z. Liu and J. Tian, J. Eur. Respir., 2019, 53, 1800986 CrossRef.
- S. Hrebien, V. Citi, I. Garcia-Murillas, R. Cutts, K. Fenwick, I. Kozarewa, R. McEwen, J. Ratnayake, R. Maudsley, T. H. Carr, E. C. de Bruin, G. Schiavon, M. Oliveira and N. Turner, Ann. Oncol., 2019, 30, 945–952 CrossRef CAS PubMed.
- M. Chen and H. Zhao, Hum. Genomics, 2019, 13, 34 CrossRef.
- B. Busser, J. Lupo, L. Sancey, S. Mouret, P. Faure, J. Plumas, L. Chaperot, M. T. Leccia, J. L. Coll, A. Hurbin, P. Hainaut and J. Charles, BioMed Res. Int., 2017, 2017, 5986129 Search PubMed.
- S. Cai, P. M. T, A. Shibakawa, R. Ren, C. L. Bevan, S. Ladame, A. P. Ivanov and J. B. Edel, Nat. Commun., 2021, 12, 3515 CrossRef.
- H. Zhang, X. Huang, J. Liu and B. Liu, Chem. Sci., 2020, 11, 3812–3819 RSC.
- H. Y. Kim, J. Song, H. G. Park and T. Kang, Sens. Actuators, B, 2022, 360, 131666 CrossRef.
- P. Miao and Y. Tang, Anal. Chem., 2020, 92, 12026–12032 CrossRef.
- P. Miao and Y. Tang, Small, 2020, 16, e2004518 CrossRef PubMed.
- L. Zhu, J. Ye, M. Yan, Q. Zhu, S. Wang, J. Huang and X. Yang, ACS Sens., 2019, 4, 2778–2785 CrossRef PubMed.
- Z. W. Sun, Y. Tong, J. Li, Y. D. Wang, F. C. Gao, H. Li, C. X. Wang, L. T. Du and Y. Y. Jiang, Sens. Actuators, B, 2022, 368, 132244 CrossRef.
- J. H. Hwang, S. Park, J. Son, J. W. Park and J. M. Nam, Nano Lett., 2021, 21, 2132–2140 CrossRef PubMed.
- W. Xu, T. Jin, Y. Dai and C. C. Liu, Biosens. Bioelectron., 2020, 155, 112100 CrossRef.
- J. Kim, A. S. Campbell, B. E. de Ávila and J. Wang, Nat. Biotechnol., 2019, 37, 389–406 CrossRef.
- Y. Dai, R. A. Somoza, L. Wang, J. F. Welter, Y. Li, A. I. Caplan and C. C. Liu, Angew Chem. Int. Ed. Engl., 2019, 58, 17399–17405 CrossRef CAS PubMed.
- S. K. Saka, Y. Wang, J. Y. Kishi, A. Zhu, Y. Zeng, W. Xie, K. Kirli, C. Yapp, M. Cicconet, B. J. Beliveau, S. W. Lapan, S. Yin, M. Lin, E. S. Boyden, P. S. Kaeser, G. Pihan, G. M. Church and P. Yin, Nat. Biotechnol., 2019, 37, 1080–1090 CrossRef CAS PubMed.
- X. Li, L. Liao, B. Jiang, W. Zhou, R. Yuan and Y. Xiang, Anal. Chim. Acta, 2022, 1197, 339521 CrossRef CAS PubMed.
- L. L. Wang, W. Q. Chen, Y. R. Wang, L. P. Zeng, T. T. Chen, G. Y. Chen and J. H. Chen, Biosens. Bioelectron., 2020, 169, 112555 CrossRef CAS PubMed.
- X. Li, X. Li, D. Li, M. Zhao, H. Wu, B. Shen, P. Liu and S. Ding, Biosens. Bioelectron., 2020, 168, 112554 CrossRef CAS.
- Y. Dai, W. Xu, R. A. Somoza, J. F. Welter, A. I. Caplan and C. C. Liu, Angew Chem. Int. Ed. Engl., 2020, 59, 20545–20551 CrossRef CAS PubMed.
- F. Hille, H. Richter, S. P. Wong, M. Bratovič, S. Ressel and E. Charpentier, Cell, 2018, 172, 1239–1259 CrossRef CAS.
- H. Shivram, B. F. Cress, G. J. Knott and J. A. Doudna, Nat. Chem. Biol., 2021, 17, 10–19 CrossRef CAS PubMed.
- A. Kostyusheva, S. Brezgin, Y. Babin, I. Vasilyeva, D. Glebe, D. Kostyushev and V. Chulanov, Methods, 2022, 203, 431–446 CrossRef CAS.
- M. Li, N. Luo, X. Liao and L. Zou, Talanta, 2023, 257, 124395 CrossRef CAS PubMed.
- X. Zhan, J. Zhou, Y. Jiang, P. An, B. Luo, F. Lan, B. Ying and Y. Wu, Biosens. Bioelectron., 2023, 229, 115229 CrossRef CAS.
- Y. Jiang, C. Zheng, M. Jin, R. Zhou, Q. Wu, F. Huang, Y. Lou and L. Zheng, J. Agric. Food Chem., 2023, 71, 4193–4200 CrossRef CAS PubMed.
- C. Li, C. Liu, R. Liu, Y. Wang, A. Li, S. Tian, W. Cheng, S. Ding, W. Li, M. Zhao and Q. Xia, Biosens. Bioelectron., 2023, 225, 115098 CrossRef CAS PubMed.
- B. Zhou, R. Yang, M. Sohail, X. Kong, X. Zhang, N. Fu and B. Li, Talanta, 2023, 254, 124120 CrossRef PubMed.
- M. Chen, J. Zhang, Y. Peng, J. Bai, S. Li, D. Han, S. Ren, K. Qin, H. Zhou, T. Han, Y. Wang and Z. Gao, Biosens. Bioelectron., 2022, 218, 114792 CrossRef PubMed.
- R. Aman, A. Mahas and M. Mahfouz, ACS Synth. Biol., 2020, 9, 1226–1233 CrossRef PubMed.
- L. B. Harrington, D. Burstein, J. S. Chen, D. Paez-Espino, E. Ma, I. P. Witte, J. C. Cofsky, N. C. Kyrpides, J. F. Banfield and J. A. Doudna, Science, 2018, 362, 839–842 CrossRef PubMed.
- F. Song, Y. Wei, P. Wang, X. Ge, C. Li, A. Wang, Z. Yang, Y. Wan and J. Li, Biosens. Bioelectron., 2021, 185, 113262 CrossRef PubMed.
- C. S. Lee, R. W. Davis and N. Davidson, J. Mol. Biol., 1970, 48, 1–22 CrossRef PubMed.
- D. F. Savage, Biochemistry, 2019, 58, 1024–1025 CrossRef CAS PubMed.
- L. Guo, Z. Mu, B. Yan, J. Wang, J. Zhou and L. Bai, Sens. Actuators, B, 2022, 350, 130874 CrossRef CAS.
- Y. Shoja, A. Kermanpur and F. Karimzadeh, Biosens. Bioelectron., 2018, 113, 108–115 CrossRef CAS PubMed.
- M. Chen, D. Wu, S. Tu, C. Yang, D. Chen and Y. Xu, Biosens. Bioelectron., 2020, 173, 112821 CrossRef PubMed.
- Y. Feng, F. Sun, N. Wang, J. Lei and H. Ju, Anal. Chem., 2017, 89, 7659–7666 CrossRef CAS.
- L. Jia, R. Zhao, Z. Feng, M. Wang, R. Ma, W. Jia, L. Shang, W. Zhang, Q. Xue and H. Wang, Sens. Actuators, B, 2022, 350, 130849 CrossRef CAS.
- Y. Huang, M. Tao, S. Luo, Y. Zhang, B. Situ, X. Ye, P. Chen, X. Jiang, Q. Wang and L. Zheng, Anal. Chim. Acta, 2020, 1107, 40–47 CrossRef CAS PubMed.
|
This journal is © The Royal Society of Chemistry 2024 |