DOI:
10.1039/D4AN00832D
(Paper)
Analyst, 2024,
149, 5022-5033
Parahydrogen-enhanced pH measurements using [1-13C]bicarbonate derived from non-enzymatic decarboxylation of [1-13C]pyruvate-d3†
Received
12th June 2024
, Accepted 15th August 2024
First published on 16th August 2024
Abstract
Alterations in pH are a hallmark in several pathologies including cancer, ischemia, and inflammation. Non-invasive magnetic resonance methods to measure pH offer a new approach for early diagnosis of diseases characterized by acid–base imbalances. The hyperpolarization with parahydrogen-induced polarization (PHIP) enhances inherently low signals in magnetic resonance experiments by several orders of magnitude and offers a suitable platform to obtain biocompatible markers in less than one minute. Here, we present an optimized preparation of an hyperpolarized H13CO3−/13CO2 pH sensor via non-enzymatic decarboxylation with H2O2 of [1-13C]pyruvate-d3 obtained by PHIP at 7 T. An improved 13C polarization of purified [1-13C]pyruvate-d3 in water with 36.65 ± 0.06% polarization was obtained starting from 50 mM precursor. Subsequent decarboxylation, H13CO3−/13CO2 exhibited 12.46 ± 0.01% of polarization at physiological pH, 45 seconds after the reaction start. Considering the dilution factor that [1-13C]pyruvate-d3 exhibits in vivo, we optimized our methodology to test the accuracy of the pH sensor at single digit millimolar concentration. In vitro pH estimations on phantoms and cell culture media demonstrated accurate pH calculations with uncertainties of less than 0.08 units. These promising results highlight the efficiency of a pH sensor generated via PHIP in less than one minute, with remarkable polarization, and biocompatibility suitable for future in vivo studies.
Introduction
pH is robustly regulated in healthy tissues and is essential for physiological processes including tissue oxygenation, proper protein structure, and various biochemical reactions.1 However, perturbations of acid–base homeostasis are a common feature of several pathological conditions including cancer, ischemia, inflammation, renal disease, and infectious diseases.2–4 It is known that the acidic microenvironment generated by cancer cells correlates with aggressiveness, migration, invasion, and metastasis potential.5–7 All these events are associated with the Warburg effect, which involves increased glucose uptakes, anaerobic glycolysis, increased production of lactate, and an acidic extracellular pH.8 Moreover, it was reported that aberrant acidifications could contribute to Alzheimer's disease and Amyotrophic lateral sclerosis’ development.9–11 Therefore, the development of safe and rapid pH measurement may aid in the early diagnosis of acid–base imbalances and subsequent pathological disorders. Although different techniques such as magnetic resonance spectroscopy (MRS), magnetic resonance imaging (MRI), and positron emission tomography (PET) have been used to measure pH in preclinical environments, none of them was successfully translated to routine clinical use.4,12–22 Regarding MRS, 31P and 19F probes were used to measure pH in vivo. However, they present small pH dependent chemical shifts and lack of sensitivity.13,23–27 Concerning MRI focused techniques, several methods were developed (e.g. CEST and the use of Gd3+).27,28 Overall, the main reasons why the noninvasive developed probes are challenging to be clinically translatable are centered on, (i) the potential toxicity of the existing probes which makes them unfeasible to be injected in routine imaging studies in patients,2–4,29–32 and (ii) the low signal sensitivity of the nuclear magnetic resonance techniques. For these reasons, the use of biocompatible probes combined with signal enhancement techniques offers great potential. MRS and MRI stands out as highly versatile and valuable tools for a wide array of applications including human imaging, in vitro and in vivo chemical and biochemical studies, and unraveling molecular structures. Despite its remarkable capabilities, its effectiveness is hindered by its inherent low sensitivity.33 However, this limitation is effectively addressed through hyperpolarized (HP) magnetic resonance. HP enhances MRS signals by over four orders of magnitude34 relying on the generation of isotopically enriched, non-toxic contrast agents that enable in vitro and in vivo metabolic kinetics studies in real-time and that have been used among other for patient pH imaging.34–36 To generate hyperpolarized 13C metabolites, two approaches are largely employed. Between them, dissolution Dynamic Nuclear Polarization (dDNP) is a technique that has been used for producing HP pyruvate,37,38 and also to produce HP bicarbonate to perform in vitro and in vivo pH measurements.2,39–44 However, clinical scale dDNP is expensive and it is a slow technique that requires times between ten minutes to hours to produce HP metabolites.45,46 Although several HP probes were successfully developed using dDNP, the needed of sophisticated and expensive equipment, as well as, the long polarization times are most likely the reasons why translation is challenging.2,3,40–42,47–54 An alternative approach to hyperpolarize metabolites is based on parahydrogen-induced polarization (PHIP). This technique converts the nuclear spin singlet of parahydrogen (pH2) into enhanced signals within seconds. One of the pH2-based hyperpolarization methods, known as Signal Amplification by Reversible Exchange (SABRE), operates through reversible exchange processes between a target molecule and a metal catalyst.55 For the classical PHIP method, an unsaturated precursor is required to which hydrogen is added. The development of PHIP-SAH (PHIP by means of the Side Arm Hydrogenation) opened up new opportunities to hyperpolarize metabolites.46 Thereby, the target molecule is functionalized with an unsaturated side arm, e.g. via esterification. The molecule with the side arm serves as an efficient precursor to which the parahydrogen is added and this approach has demonstrated the highest yielding levels of polarization with pH2 so far. In particular fully deuterated precursors have shown to deliver optimal polarization conditions due to the reduction of dipolar couplings to hydrogen originating from parahydrogen, prolonging T1.56 Overall, the unsaturated bond is hydrogenated by using pH2 and a catalyst, usually in organic solvents. Next, the yielded proton spin order is transferred to a target 13C nucleus. In our work we use the spin order transfer sequence called MINERVA (Maximizing Insensitive Nuclei Enhancement Reached Via parahydrogen Amplification) for this transfer.56 Following the magnetization transfer, the side chain is removed by hydrolysis enabled by the addition of a base. The organic solvent is removed by evaporation and after subsequent filtration, the pH is adjusted to physiological values, and finally, after around one minute, the HP 13C-compound is obtained.34 The procedures including used precursors are shown in Fig. 1A and PHIP-SAH has overall allowed to enhance important metabolites for in vivo studies.34–36,46,57–64 Thereby 13C-pyruvate is used as key metabolite for a non-invasive kinetic evaluation of conversions from pyruvate to lactate in real-time in vitro and in vivo.34,56,59,65 Even though metabolites and especially pyruvate can be hyperpolarized with parahydrogen, so far, no biocompatible parahydrogen derived pH sensor is available at sufficient polarization (>10% 13C polarization). The latter we are introducing in this work by performing a non-enzymatic decarboxylation in which H2O2 present a nucleophilic addition to the α-carbonyl group of pyruvate to generate an unstable intermediate, which reacts to CO2 that is in equilibrium with HCO3−, acetate, and water at neutral pH (Fig. 1B).35,66
 |
| Fig. 1 Schematic representation of the PHIP-SAH workflow; (A) full workup that includes: pH2 bubbling (20 s), MINERVA polarization transfer (10 s), base addition (1 s), acetone evaporation (8 s), buffer addition (1 s) and filtration (3 s); (B) decarboxylation process to obtain PHIP-H13CO3−/PHIP-13CO2 (reaction takes 45 s), and pH measurement at 7 T. Own Fig. created with BioRender.com. | |
Materials and methods
Chemicals
All chemicals were purchased from Sigma Aldrich, except the precursors that were synthesized according to published procedure.35,61,67 Please refer to the ESI† for further information (Schemes S1 and S2†).
Hyperpolarization and non-enzymatic decarboxylation: procedure and equipment
[1,4-Bis(diphenylphosphino)butane](1,5-cyclooctadien)rhodium(I)-tetrafluorborat (Sigma Aldrich, catalog # 79255-71-3) was dissolved in acetone-d6 and used as catalyst for the PHIP procedure with a final concentration of 10 mM. After mixing with the precursor (vinyl pyruvate-d6 at 50 mM or phenyl propargyl pyruvate ester-d6 at 5.5 mM), the samples were filled into a 7-inch 5 mm NMR tube and bubbled with N2 for 10 seconds for degassification. para-enriched hydrogen was obtained at 20 K with a He-cooled parahydrogen generator provided by a Cryocooler system (Sumimoto HC-4A helium compressor, Sumimoto Cold Head CH-204 with pH2 reaction chamber by ColdEdge Technologies, temperature controller Lake Shore Cryotronics, Inc.) and delivered by a home-built valve and tubing system. Hyperpolarization and polarization transfer were carried out in a Bruker Avance III HD 300 narrow bore spectrometer operating at 7.05 T field strength equipped with a room temperature broadband observe (BBO) probe head for sample diameters of 5 mm (PA BBO 300S1 BBF-H-D-05 Z). Vinyl pyruvate-d6 was hyperpolarized by using the MINERVA sequence as described previously (detailed in the ESI, Fig. S2A and B†).35,65 For hyperpolarization of phenyl propargyl pyruvate ester-d6, a field-independent MINERVA sequence for the transfer of longitudinal spin order of parahydrogen polarization was applied as previously published (detailed in the ESI†).36 After finalization of both processes (30 s), 200 μL of a Na2CO3 in D2O (75 mM for 50 mM of the vinyl pyruvate-d6 precursor or 7.5 mM for 5.5 mM of the phenyl propargyl pyruvate ester-d6 precursor), containing EDTA (1 mM in D2O, which prolongs T1 and stabilize the polarization),68 and sodium ascorbate (50 mM in D2O, which decreases the polarization loss),69 were added to cleave the side arm and release hyperpolarized [1-13C]pyruvate-d3. Then, the NMR tube was placed outside the magnet and acetone was evaporated by passing N2 at 7 bar pressure through the tube, and by immersing the tube in warm water (∼79 °C) for 8 seconds.34 After evaporation, indicated by a reduction of the total volume by one half in these 8 s (the volume of acetone and base added were 200 μL each, so the reduction of the acetone volume was visually checked), 50 μL of HEPES (100 mM, pH 10 in D2O) were added to obtain physiological conditions in the final solution (1 s). The aqueous solution was then filtered from the NMR tube into a syringe by using a PVDF filter with a pore size of 1.0 μm (Fisher Scientific) to obtain in 3 s the catalyst-free signal-enhanced [1-13C]pyruvate-d3.34 Finally, 50 μL of H2O2 at 326 mM (final concentration 50 mM, 0.15%) was added to induce the non-enzymatic decarboxylation. 25 μL CaCl2 at a concentration of 3 mM was injected together with H2O2 to increase the decarboxylation rate. Fig. 1 and Fig. S1 (ESI)† show a schematic representation of the entire procedure. In Fig. 2 is shown the spectrum recorded after 45 s of the reaction.
 |
| Fig. 2 Non-enzymatic decarboxylation of HP [1-13C]pyruvate-d3 (50 mM) with H2O2 (50 mM) at 50 °C. Single 13C spectrum recorded at 7 T after 45 s of H2O2 injection. | |
Monitoring of pyruvate decarboxylation
The decarboxylation reaction of pyruvate was followed spectroscopically at 50 °C using a series of 90° single scan 1H NMR at 7 T every 5 s, after mixing 30 mM of Sodium pyruvate in D20 (concentration of pyruvate expected in the decarboxylation solution when starting from 50 mM in hyperpolarization experiments due to dilution by addition of buffer, H2O2, and CaCl2), with 50 mM of H2O2, and 3 mM of CaCl2 both in D2O. The integral intensities of the 1H signals of the methyl group of each species were converted to concentrations. Below is detailed the model described by Tickner et al. (2020),70 followed for the respective equations:
[A]t+δt = [A]t + (−kH2O2[A]t[D]t − kHy[A]t + k-Hy[B]t)∂t |
[B]t+δt = [B]t + (kHy[A]t − k-Hy[B]t)∂t |
[C]t+δt = [C]t + (kH2O2[A]t[D]t)∂t |
[D]t+δt = [D]t − (kH2O2[A]t[D]t)∂t |
where A represents sodium pyruvate, B is pyruvate hydrate, C is acetate, and D is the rate of reaction between pyruvate and H2O2 obtained by fitting the integral intensities of 1H CH3 resonances of A, B, and C. k-Hy, kHy, and kH2O2 are the rates of each reaction, and in the equations δt is an incremental time difference.
As described by Tickner et al. (2020),70 rate constants were found by minimizing the differences between concentrations determined experimentally and the calculated values. We also maintained the same assumptions, in which species as H2O, pyruvate dimers or enol pyruvate, and the pathway that allows pyruvate hydrate reaction with H2O2 were not included in the model. In the ESI† are detailed the obtained kinetic parameters and the fitting (Table S1 and Fig. S3†).
PHIP H13CO3−/13CO2 pH measurements in phantoms
HEPES buffer solutions in D2O were prepared at different pH values (6.40, 6.50, 6.61, 6.70, 6.81, 6.90, 7.20, and 7.40). The pH of each solution was adjusted using HCl (100 mM in D2O) and NaOH (100 mM in D2O), as necessary, and verified using a calibrated pH electrode. The pH measurements were performed in a Bruker Avance IV 300 7.05 T wide bore spectrometer equipped with a MicWB40 rf probe and Micro 2.5 WB gradient system at room temperature. The phantom consisted in eight empty NMR tubes labelled from 1–8 and placed surrounding a urea phantom tube, which was used as a positioning reference (Fig. 4A). 350 μL of HEPES at pH 6.4 were injected into tube 1, immediately before the injection of 175 μL of the H13CO3−/13CO2 (5.5 mM). After injection of H13CO3−/13CO2, we conducted non-localized spectroscopic experiments with low-flip angle excitations to obtain the H13CO3−/13CO2 integrals. Specifically, seven seconds after injection, a train of 20° flip angle pulses was initiated and a spectrum was acquired every 3 seconds. After achieving the steady state (state in which the rates of the forward and reverse reactions are equal, leading to constant concentrations of the involved species), the pH value of the respective tube was calculated by using the Henderson–Hasselbalch equation (more details in the ESI†). This procedure was repeated for each subsequent tube (2–8) to conduct the pH measurements. Each experiment was separated by a 5-minute interval to ensure that no residual signal was detected from the previous tube. This interval allowed any potential HP signal to dissipate, ensuring accurate readings. Once the pH measurements were finished for all the tubes, 500 μL of H2O were injected into each tube to obtain a representative 1H image of the setup (Fig. 4A). For this, a FLASH sequence (fast low angle shot magnetic resonance imaging) with a field of view of 30 × 30 mm2, a matrix size of 256 × 256, and a central slice with 3 mm thickness in the transversal plane were used. Finally, the 1H image and pH values obtained from the spectroscopic measurements were employed to generate a pH color-representative graph using MATLAB software (Fig. 4B).
PHIP H13CO3−/13CO2 pH measurements in cell culture media
Mouse pancreatic ductal adenocarcinoma cell line (PanC02) were kindly provided by Professor Stine Pedersen (University of Copenhagen) and Professor Frauke Alves (MPI NAT, Goettingen), and they were cultured in DMEM without bicarbonate, with HEPES, supplemented with 10% Fetal bovine Serum, and 1% penicillin/streptomycin, at 37 °C, 5% CO2 in a humidified incubator. After four days in vitro, cell culture media was collected from different flasks and the pH was measured with a pH-electrode. Furthermore, fresh media-pH was also measured with the electrode. Immediately after that, we carried out pH measurement with our probe, using identical conditions as detailed before for phantoms measurements. Table S4 in the ESI† shows the individual pH values obtained for each experiment.
Measurement of polarization and T1
Polarization values were determined from six independent experiments, by comparing the signal intensity from one hyperpolarized sample recorded with a 90° pulse to a spectrum measured at thermal equilibrium. The thermal spectrum was measured with the same acquisition parameters, T = 290 K using 160 scans. Peak areas from the hyperpolarized spectra were corrected according the flip angle (20°) and fitted to a decaying exponential to approximate T1 values.
Data analysis, % polarization calculation, and statistics
The spectra were processed using TopSpin software version 4.0.8. Phase correction, line-broadening (LB 5 for EM), and baseline corrections were applied. Further analyses and 3D plot were performed using MATLAB software version R2019b. In each spectrum, the peaks of HCO3− and CO2 were integrated to determine the area under the curves (AUCs). From the ratio of the AUCs, pH values were calculated by using Henderson–Hasselbalch equation. Correlation and statistical analysis were perform in GraphPad Prism version 9 software.
Polarization level was calculated using the following equation:
In this equation, Phyp represents the polarization level of the sample in the hyperpolarized spectra, and Pth the polarization value of the sample in the thermal spectra. I represents the integration in the same spectra region (e.g.13C NMR signal of pyruvate at around 170 ppm) in both, hyperpolarized (Ihyp) and thermal spectra (Ith). rg is the receiver gain of the spectrometer, sin(θth) = 1 and nshyp = 1, as the thermal experiments were acquired with a flip angle of 90° degrees, and a singles scan is used in hyperpolarization experiments. A comparison between one single 13C scan recorded for 50 mM of hyperpolarized (HP) [1-13C]-pyruvate-d6 and its corresponding thermal spectrum is showed in the ESI (Fig. S4).†
To calculate polarization levels for the H13CO3−/13CO2 species, we took the most conservative calculation using H13CO3−/13CO2 integrals after purification workup, and 45 s after H2O2 injection, in at least three different experiments.
Results and discussion
PHIP pH probe development
In this work, a H13CO3−/13CO2 probe was developed to measure extracellular pH. This probe was obtained in D2O at physiological conditions by non-enzymatic decarboxylation of signal enhanced 1-13C-pyruvate (see Fig. 1B).35 Fully deuterated precursors were used to restrict the effective nuclear spin system and minimizing the loss of signal enhancement.35 In a first step the hyperpolarization of [1-13C]pyruvate-d3 obtained from vinyl pyruvate-d6 was optimized and an average polarization of 36.65 ± 0.06% (see ESI†) was obtained starting from 50 mM 1-13C-vinyl pyruvate-d6 precursor. It is worth to mention that the highly obtained polarization on pyruvate would enable the preparation of the metabolic probes with liquid nitrogen cooled parahydrogen for which polarization levels would be a factor of three less but still exceed 10% 13C polarization. Note that this is the measured polarization of purified pyruvate in D2O. The purification procedure was performed by cleavage of the side arm with Na2CO3 (75 mM) supplemented with EDTA (1 mM), and sodium ascorbate (50 mM). After obtaining free [1-13C]pyruvate-d3 at 50 °C, the pH was adjusted to biocompatible conditions with HEPES-buffered D2O. Followed by pH adjustment, H2O2 was added to induce the non-enzymatic decarboxylation, together with CaCl2 to increase the decarboxylation rate.2,71,72 We also investigated our previously introduced Phenyl propargyl pyruvate ester precursor (PPE) at high field together with a field-independent pulsed PHIP-SAH method, which however yielded low degrees of pyruvate polarization. The complete process yielded 2.45 ± 0.01% pyruvate polarization that might be due to reduced relaxation times as compared to low field applications.36
We attribute that remarkable polarization value achieved on [1-13C]pyruvate-d3 starting from vinyl pyruvate-d6 to some changes implemented in the purification and pH stabilization procedure. In contrast to previous purification methodologies employed by us,34–36,56,65 that involved the use of Na2CO3 (100 mM) in H2O, the present methodology employed the same base, but in D2O, with a concentration of 75 mM, and supplemented with EDTA (which prolongs T1 and stabilize the polarization, according to dDNP experiments),68 and sodium ascorbate (which decreases the polarization loss during cleavage as previously reported).69 Notably, the evaporation procedure involved the use of N2 bubbling, differing from the conventional vacuum-assisted evaporation.36
The final adjustment of pH was performed through the addition of HEPES dissolved in D2O, departing from the prior use of HEPES in H2O. It was reported that retaining pyruvate in PBS-D2O, instead of PBS-H2O leads to lengthening of relaxation times which may play a crucial role in this process.45 Moreover, in a recently published study, a similar effect was observed when H13CO3−/13CO2 were obtained via hydrolysis of HP [1-13C]1, 2-glycerol carbonate. Hydrolysis conducted with NaOH in D2O affords superior polarization values and extended lifetimes compared to its counterpart in H2O.52 In this point, it is important mentioning that D2O is safe for in vivo and humans injection, as was previously informed.45,73 In a next step, we added H2O2 (50 mM) and found that after 45 s the reaction intermediates had sufficiently been converted into H13CO3−/13CO2. A total 13C polarization of 12.46 ± 0.01% was estimated based on the initial 13C thermal signal of the precursor. Fig. 2 illustrates a 13C spectrum recorded at 7 T using a single-90 degree pulse, post-purification, and subsequent to 45 s of H2O2 injection. H13CO3−/13CO2 are the main species present, together with small traces of [1-13C]pyruvate-d3 (∼171 ppm), the intermediate (2-hydroxyperoxy-2-hydroxypropanoate, ∼176 ppm), and pyruvate hydrate (∼178 ppm).35,74
[1-13C]pyruvate-d3 decarboxylation kinetics
Furthermore, we monitored the sodium pyruvate decarboxylation rate following the model previously reported by Tickner et al.70 Briefly, 30 mM of sodium pyruvate (concentration expected in the decarboxylation solution when starting from 50 mM in hyperpolarization experiments) were decarboxylated by the addition of 50 mM of H2O2 in D2O and 3 mM of CaCl2 in D2O. The reaction was monitored spectroscopically at 50 °C using a series of 90° single scan 1H NMR at 7 T every 5 s. The integral intensities of pyruvate, acetate, and pyruvate hydrate were converted to concentration and plotted over time (see ESI†). By fitting these data to the kinetic model described by Tickner et al. using the same assumptions (e.g. omission of H2O, pyruvate dimers, or enol pyruvate species, as well as, omission of the reaction between pyruvate hydrate and H2O2),70 the rate of the reaction between pyruvate and H2O2 was calculated. The kH2O2 value was estimated as (0.88 ± 0.14) dm3 mol−1 s−1 (please refer to the ESI† for further details). Even though this value demonstrate a rapid decarboxylation rate of pyruvate, and pyruvate conversion was approximately of 76%. 9 mM of uncarboxylated pyruvate (24%) were present after 45 s of H2O2 addition. Given the signal strengths shown in Fig. 2 we do not expect interferences due to the low concentration. However, if it is considered that uncarboxylated pyruvate could be transformed in vivo to lactate (∼182 ppm) and alanine (∼176 ppm), there are no major concerns since the residual products will not interfere with the signal of the species used for the pH measurements H13CO3− (∼160 ppm), and 13CO2 (∼128 ppm), and actually could provide valuable information about real-time metabolic kinetics. Concerning H2O2 concentration, assuming a 1
:
1 relation between acetate formed and H2O2 consumed, it was observed that at 45 s the remaining concentration of H2O2 is approximately 20 mM (0.06%) in 325 μL. Human studies exist in which H2O2 in concentrations between (0.06–0.48)% were injected via intra-arterial or intravenous (evaluating its therapeutic potential on head and neck cancer, arteriosclerosis, wound healing) did not show toxicity.75 On in vivo experiments, the reported intravenous (median lethal dose) LD50 in mice was founded to be 50
000 mg per kg body weight.76 Therefore, given that facts, and the fact that that our solution would dilute by the blood volume (∼6 L in humans, 77–80 μL g−1 in mice), the residual concentration does not appear to pose a concern to humans or mice. Nevertheless, upon a potential advancement into clinical trials these procedures would need to be optimized by ideally further reducing the H2O2 content and performing a toxicology assessment.
Regarding CaCl2 at the final solution, this is added during decarboxylation at 3 mM, concentration that is much lower than used safely in humans and mice for calcium replenishment in arrhythmias associated with hypocalcemia, hyperkalemia, an antidote for magnesium poisoning, as co-adjuvant in hemostatic agents preparations, as well as, to resolve refractory hypotension and complete heart block, etc.77–81 Additionally, Kawai et al. (2011) reported the use of CaCl2 injected intravenously (via tail vein) in mice to show how this compound accelerate wound healing. In these experiments a dose of 0.6 mg per mouse was used without any observed side effects.82 In our injections, even if all the initial CaCl2 were present in the final solution, it will represent a dose of 0.0033 mg per mouse, so side effects are unlikely. Respect to rhodium residuals, we have previously reported that the levels at the final solution after filtration is (17 ± 5) μM.56 This concentration have not generated any side effects in vivo or toxicity in cells experiments, even when double injection of the probe was used.34,56,59,65 Finally, acetate is expected to be present in the final solution. However, it has been previously reported the use of hyperpolarized acetate as a tracer in different diseases and events (e.g. diabetes, cardiac and renal metabolism, glioma, etc.) on in vivo and human studies demonstrating that this compound does not pose challenges.83–87 In addition to that, acetate-buffers were extensively used as first-line intravenous resuscitation fluids in patients, for example during hemorrhagic shock.88–90
PHIP H13CO3−/13CO2 validation in phantoms
During in vivo experiments, [1-13C]pyruvate-d3 is injected into the mouse's tail vein, thus suffering a dilution because of the mouse blood volume. Considering that the approximate blood volume of a mouse is 77–80 μL g−1,91,92 and that in our previous in vivo experiments with pyruvate usually C57BL6 mice with a weight of ∼20 g (10–12 weeks) are used,34,65 the final concentration of biomarkers when injected into the vein tail (volume injected ∼50–100 μL) will decrease about by a factor of ten.
Taking that into account, we wanted to evaluate the accuracy of the pH sensor at a 10-fold lower concentration. Following establishment of the procedure, pH measurements of eight phantoms were performed in a 7 T imaging spectrometer (more details in the ESI†). Each phantom contained HEPES buffers at different pH (6.40, 6.50, 6.61, 6.70, 6.81, 6.90, 7.20, and 7.40) in D2O. After filtration and decarboxylation, the solution containing H13CO3−/13CO2 was injected into the different tubes (one by one, and after an interval of 5 min, as explained in Materials and Methods section) containing the mentioned buffers. Upon injection, 13C-spectra were acquired with low-flip angle excitations (20°) to obtain H13CO3−/13CO2 integrals (Fig. 3 shows one example). Once achieving the steady state (state with constant concentration, according to two-way ANOVA followed by the Bonferroni's test for multiple comparisons), pH was calculated by using the Henderson–Hasselbalch equation: pH = 6.2 + log([HCO3−]/[CO2]),93 and the ratio of the H13CO3−/13CO2 integrals. Table 1 shows the means ± SD for H13CO3−/13CO2-measured pH values and their corresponding electrode-measured values, obtained after three independent experiments. Following spectroscopic measurements, the pH of the final solutions was determined with a pH electrode, and the values were in good agreement with those determined from the spectroscopic data (Table 1). This pH sensor methodology demonstrates accuracy with standard deviations lower than 0.07 pH units (expanded details in the ESI†).
 |
| Fig. 3 Example for a time series of 13C NMR spectra recorded with a flip angle of 20° upon injection of signal-enhanced H13CO3−/13CO2 in a phantom of pH 6.5, after non-enzymatic decarboxylation of hyperpolarized [1-13C]pyruvate-d3. A urea phantom was used in order to ensure correct position of the phantom tubes during the measurements, and its signal is in antiphase showing non-hyperpolarization. | |
Table 1 Measured pH by using our probe and spectroscopic data, and pH measured with an electrode
Phantom # |
Electrode pH (Mean ± SD) |
PHIP H13CO3−/13CO2 pH (Mean ± SD) |
1 |
6.40 ± 0.01 |
6.37 ± 0.01 |
2 |
6.50 ± 0.01 |
6.50 ± 0.05 |
3 |
6.61 ± 0.01 |
6.60 ± 0.007 |
4 |
6.70 ± 0.01 |
6.65 ± 0.02 |
5 |
6.81 ± 0.01 |
6.76 ± 0.03 |
6 |
6.90 ± 0.01 |
6.91 ± 0.06 |
7 |
7.20 ± 0.01 |
7.15 ± 0.01 |
8 |
7.40 ± 0.01 |
7.35 ± 0.07 |
Likewise, H13CO3−/13CO2 pH values did not differ significantly from the electrode-measured ones, according to two-way ANOVA followed by the Bonferroni's test for multiple comparisons. Fig. 4A shows a 1H image of our measurement setup (as explained in Materials and Methods). To ensure the correct position of the tubes, a urea-13C phantom was placed in the center. In (B) a color map represents the pH values obtained with our probe. (C) and (D) shows the correlation between the mean of H13CO3−/13CO2 pH values obtained from three independent experiments and electrode pH. The close correlation obtained reflects a rapid establishment of chemical equilibrium and equilibration of polarization between the H13CO3−/13CO2 species. Although previous studies reported a difference of ∼0.3 pH units where the H13CO3−/13CO2 pH was higher than the electrode-measured pH in the absence of the enzyme carbonic anhydrase (by using voxel by voxel estimation),39 we did not observe the same effect in our spectroscopic data. Our hypothesis relies on an improvement of T1,45,52 attributable to the generation of the signal-enhanced H13CO3−/13CO2 in D2O (instead of H2O).
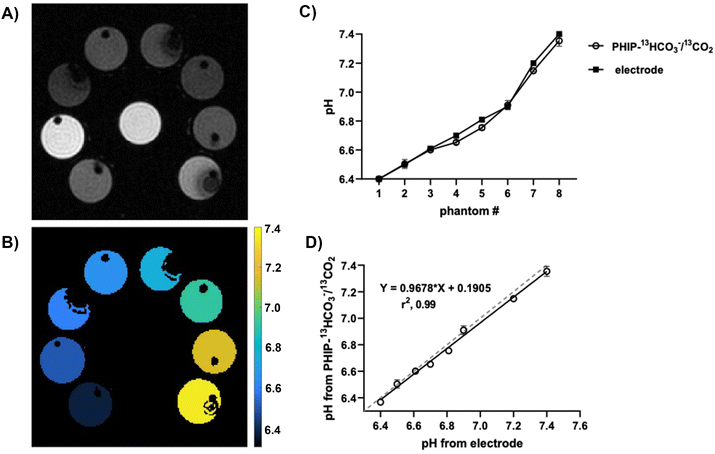 |
| Fig. 4 (A) Proton imaging of the setup acquired at the end of the measurements by adding H2O to all the measured tubes. The tube in the center corresponds to a urea phantom, which was used as a positioning reference. (B) Color graphical representation of the pH values generated in MATLAB software by using the pH values obtained spectroscopically from the ratio of hyperpolarized H13CO3−/13CO2 integrals, and the 1H image showed in A. (C) pH vs. phantom number (#) for PHIP H13CO3−/13CO2-pH and electrode-pH. No statistically differences were found after analysis by two way ANOVA followed by the Bonferroni's test for multiple comparisons using GraphPad Prism 9 software. (D) Correlation of the PHIP H13CO3−/13CO2-pH from spectroscopic data with the values measured using a pH electrode. A solid linear regression line with its corresponding equation is shown for the measured pH with our probe, and a dashed line that represents direct proportionality between the two variables. | |
The T1 values for these species in D2O at 7 T and room temperature were (54.3 ± 9.7) s for H13CO3−, and (49.0 ± 3.0) s for 13CO2. This might facilitate a rapid exchange between H13CO3−/13CO2, and the rate of polarization loss for both species becomes similar (according to t-student, p-value from the comparison of the T1 values is 0.2, not statistically different). That rapid interconversion could contribute to maintain pH values during the steady state as is shown in Fig. 5, increasing the accuracy of the measurements.2 Another fact that could contribute to the improved correlation between PHIP H13CO3−/13CO2-pH and electrode-pH, is based on the use of an alkaline buffer (pH = 10) in our procedure to achieve physiological conditions (pH = 7), this step would be responsible for reducing CO2 losses prior to injection.3
 |
| Fig. 5 Profile of normalized integral (arbitrary unit) of H13CO3− and 13CO2, and pH vs. time, after injection of signal-enhanced H13CO3−/13CO2 into a HEPES phantom of pH 6.5. pH values from the steady state were selected to calculate the ones showed in Table 1. | |
PHIP H13CO3−/13CO2 validation in cell culture media
In order to test our probe in a more biological-complex environment, pH measurements were conducted in conditioned media from pancreatic cancer cells (PanC02 cell line) corresponding to 4 days in vitro (div). These measurements were compared with fresh media containing all the same supplements than the media used for PanC02 culture. Specifically, the media used was DMEM high glucose, without bicarbonate supplemented with 10% fetal bovine serum and 1% penicillin/streptomycin. Fig. 6 shows the correlation of the PHIP H13CO3−/13CO2-pH from spectroscopic data with the values measured using an electrode.
 |
| Fig. 6 Correlation PHIP H13CO3−/13CO2-pH and electrode-pH values obtained from cell culture media. A solid linear regression line and equation is shown for the measured H13CO3−/13CO2-pH, the dashed line that represents direct proportionality between the two variables. | |
The mean for pH values measured with our probe are summarized in Table 2 (please see the ESI† for more details).
Table 2 Measured pH values in conditioned media from PanC02 cells and fresh media using our probe and spectroscopic data, and pH measured with electrodes
Media |
PHIP H13CO3−/13CO2 pH |
Electrode pH |
PanC02, 4 div |
6.87 ± 0.08 |
6.88 ± 0.01 |
Fresh media |
7.45 ± 0.06 |
7.51 ± 0.01 |
In this case, accurate pH values were also found in agreement with those measured by using the electrode.
Lastly, we present a probe prepared by using highly polarized pyruvate (36.65%), and a non-enzymatic decarboxylation reaction to achieve the H13CO3−/13CO2 pH sensor, with a polarization value of 12.46%. It is important to note that the polarization losses for the H13CO3−/13CO2 sensor can be attributed mainly to relaxation effects (T1 54.3 s for H13CO3−, and 49.0 s for 13CO2), which could be further improved in the future by optimized reactions in an automatized setup. We have validated our probe in vitro by measuring pH on phantoms and pancreatic cancer cell media with highly accuracy. Previous H13CO3−/CO2 studies were conducted with dDNP achieving similar levels of polarization: Ghosh et al. (2014), reached H13CO3− with notable polarization (∼16%) by employing α-keto carboxylic acid-derivatives decarboxylation with H2O2 and validated the probe on perfused rat lungs.2 Besides, Mu et al. (2023) applied chemical reactions as hydrolysis followed by neutralization on 1,2-glycerol carbonate (GLC) to obtain H13CO3− with a polarization of ∼20%, and its validation was carried out in several in vivo healthy and cancer mouse models.52 Other probes derived from synthetic compounds and amino acids were developed as HP chemical-shift based pH sensors.47,48,51,94 As mentioned above, dDNP is facing challenges on the development to routine clinical use. With respect to pH sensors for example, polarization times of 2 h are needed (probe obtained by direct polarization on CsH13CO3),39 1.5 h (probe obtained by decarboxylation of HP α-keto carboxylic acid-derivatives),2 and 7 h (probe obtained by base-catalyzed hydrolysis followed by neutralization on 13C-GLC),52 together with all the time needed for reaction and post dilution steps. Therefore, parahydrogen based methods pose a scalable solution, as hyperpolarized agents can be rapidly produced on-site and even in portable devices. Finally, assessment of parahydrogen enhanced contrast agents in large volumes (40 mL as in dDNP) and patient studies are currently not feasible and are part of our ongoing work.
Conclusions
In conclusion, we present an optimized methodology to obtain biocompatible hyperpolarized H13CO3−/13CO2 pH sensor within a minute using PHIP to perform pH measurements at physiological conditions with high accuracy. To achieve the probe, purified [1-13C]pyruvate-d3 with a notable 13C polarization of 36.65 ± 0.06% in aqueous solution and at physiological pH is decarboxylated. For this, H2O2 with an addition of CaCl2 yield signal-enhanced H13CO3− and 13CO2 in D2O with an average 13C-polarization of 12.46 ± 0.01%. We attribute these remarkable polarization values to optimizations performed in our purification and pH adjustment procedures. We envision that the presented procedures, which offer advantages in polarization efficiency, accuracy, and speed, can be rapidly adopted for preclinical investigations and future clinical parahydrogen procedures for patient imaging.
Author contributions
Author contributions are the following: M. D. Santi, conceptualization, data curation, formal analysis, investigation, methodology, visualization, and writing-original draft; T. L. K. Hune, investigation, formal analysis, and writing-review and editing; G. G. Rodriguez, investigation, formal analysis, and writing-review and editing; L. M. Fries, investigation, formal analysis, and writing-review and editing; R. Mei, investigation, formal analysis, and writing-review and editing; S. Sternkopf, visualization, and writing-review and editing; Josef Elaßer investigation; and S. Glöggler, conceptualization, validation, funding acquisition, resources, supervision, and writing-review and editing. All authors have given approval to the final version of the manuscript.
Data availability
The data supporting this article have been included as part of the ESI.†
Conflicts of interest
Stefan Glöggler is co-founder of MagniKeen
Acknowledgements
The authors thank Dr Stevanato for his support with the experimental set up, and Dr Belov and the Chemical synthesis facility team for their support in the scale up of the deuterated pyruvate precursor. Pancreatic cancer cell line (PanC02) kindly provided by Professor Stine Pedersen from University of Copenhagen. This project has received funding from the European Research Council (ERC) under the European Union's Horizon 2020 research and innovation program (Grant Agreement No. 949180). Stefan Glöggler acknowledges funding by the Max Planck Society and the German Research Foundation (DFG) under Grant 495627437, 491827624 and 450146057.
References
-
E. Hopkins, T. Sanvictores and S. Sharma, StatPearls, Treasure Island (FL), 2024 Search PubMed.
- R. K. Ghosh, S. J. Kadlecek, M. Pourfathi and R. R. Rizi, Efficient production of hyperpolarized bicarbonate by chemical reaction on a DNP precursor to measure pH, Magn. Reson. Med., 2015, 74, 1406–1413 CrossRef CAS PubMed.
- F. A. Gallagher, M. I. Kettunen and K. M. Brindle, Imaging pH with hyperpolarized 13C, NMR Biomed., 2011, 24, 1006–1015 CrossRef CAS PubMed.
- R. J. Gillies, N. Raghunand, M. L. Garcia-Martin and R. A. Gatenby, pH imaging. A review of pH measurement methods and applications in cancers, IEEE Eng. Med. Biol. Mag., 2004, 23, 57–64 CrossRef PubMed.
- D. Czaplinska, R. Ialchina, H. B. Andersen, J. Yao, A. Stigliani, J. Dannesboe, M. Flinck, X. Chen, J. Mitrega, S. P. Gnosa, O. Dmytriyeva, F. Alves, J. Napp, A. Sandelin and S. F. Pedersen, Crosstalk between tumor acidosis, p53 and extracellular matrix regulates pancreatic cancer aggressiveness, Int. J. Cancer, 2023, 152, 1210–1225 CrossRef CAS PubMed.
- F. Visioli, Y. Wang, G. N. Alam, Y. Ning, P. V. Rados, J. E. Nor and P. J. Polverini, Glucose-regulated protein 78 (Grp78) confers chemoresistance to tumor endothelial cells under acidic stress, PLoS One, 2014, 9, e101053 CrossRef PubMed.
- M. Kato, K. Maeda, R. Nakahara, H. Hirose, A. Kondo, S. Aki, M. Sugaya, S. Hibino, M. Nishida, M. Hasegawa, H. Morita, R. Ando, R. Tsuchida, M. Yoshida, T. Kodama, H. Yanai, T. Shimamura and T. Osawa, Acidic extracellular pH drives accumulation of N1-acetylspermidine and recruitment of protumor neutrophils, PNAS Nexus, 2023, 2, pgad306 CrossRef PubMed.
- O. Warburg, On the origin of cancer cells, Science, 1956, 123, 309–314 CrossRef CAS PubMed.
- H. Prasad and R. Rao, Amyloid clearance defect in ApoE4 astrocytes is reversed by epigenetic correction of endosomal pH, Proc. Natl. Acad. Sci. U. S. A., 2018, 115, E6640–E6649 CrossRef CAS PubMed.
- S. W. Kuo, M. Jiang and C. Heckman, Potential involvement of intracellular pH in a mouse model of amyotrophic lateral sclerosis, Amyotrophic Lateral Scler. Frontotemporal Degener., 2014, 15, 151–153 CrossRef PubMed.
- A. Rahman, B. Janic, T. Rahman, H. Singh, H. Ali, R. Rattan, M. Kazi and M. M. Ali, Immunotherapy Enhancement by Targeting Extracellular Tumor pH in Triple-Negative Breast Cancer Mouse Model, Cancers, 2023, 15, 4931 CrossRef CAS PubMed.
- G. V. Martinez, X. Zhang, M. L. Garcia-Martin, D. L. Morse, M. Woods, A. D. Sherry and R. J. Gillies, Imaging the extracellular pH of tumors by MRI after injection of a single cocktail of T1 and T2 contrast agents, NMR Biomed., 2011, 24, 1380–1391 CrossRef CAS PubMed.
- R. J. Gillies, Z. Liu and Z. Bhujwalla, 31P-MRS measurements of extracellular pH of tumors using 3-aminopropylphosphonate, Am. J. Physiol., 1994, 267, C195–C203 CrossRef CAS PubMed.
- S. Gil, P. Zaderenzo, F. Cruz, S. Cerdan and P. Ballesteros, Imidazol-1-ylalkanoic acids as extrinsic 1H NMR probes for the determination of intracellular pH, extracellular pH and cell volume, Bioorg. Med. Chem., 1994, 2, 305–314 CrossRef CAS PubMed.
- R. van Sluis, Z. M. Bhujwalla, N. Raghunand, P. Ballesteros, J. Alvarez, S. Cerdan, J. P. Galons and R. J. Gillies, In vivo imaging of extracellular pH using 1H MRSI, Magn. Reson. Med., 1999, 41, 743–750 CrossRef CAS.
- Z. M. Bhujwalla, D. Artemov, P. Ballesteros, S. Cerdan, R. J. Gillies and M. Solaiyappan, Combined vascular and extracellular pH imaging of solid tumors, NMR Biomed., 2002, 15, 114–119 CrossRef CAS PubMed.
- M. L. Garcia-Martin, G. Herigault, C. Remy, R. Farion, P. Ballesteros, J. A. Coles, S. Cerdan and A. Ziegler, Mapping extracellular pH in rat brain gliomas in vivo by 1H magnetic resonance spectroscopic imaging: comparison with maps of metabolites, Cancer Res., 2001, 61, 6524–6531 CAS.
- A. K. Jindal, M. E. Merritt, E. H. Suh, C. R. Malloy, A. D. Sherry and Z. Kovacs, Hyperpolarized 89Y complexes as pH sensitive NMR probes, J. Am. Chem. Soc., 2010, 132, 1784–1785 CrossRef CAS PubMed.
- S. Aime, A. Barge, D. Delli Castelli, F. Fedeli, A. Mortillaro, F. U. Nielsen and E. Terreno, Paramagnetic lanthanide(III) complexes as pH-sensitive chemical exchange saturation transfer (CEST) contrast agents for MRI applications, Magn. Reson. Med., 2002, 47, 639–648 CrossRef CAS PubMed.
- N. Raghunand, S. Zhang, A. D. Sherry and R. J. Gillies, In vivo magnetic resonance imaging of tissue pH using a novel pH-sensitive contrast agent, GdDOTA-4AmP, Acad. Radiol., 2002, 9(Suppl 2), S481–S483 CrossRef PubMed.
- D. A. Rottenberg, J. Z. Ginos, K. J. Kearfott, L. Junck, V. Dhawan and J. O. Jarden, In vivo measurement of brain tumor pH using [11C]DMO and positron emission tomography, Ann. Neurol., 1985, 17, 70–79 CrossRef CAS PubMed.
- X. Zhang, Y. Lin and R. J. Gillies, Tumor pH and its measurement, J. Nucl. Med., 2010, 51, 1167–1170 CrossRef CAS PubMed.
- V. D. Mehta, P. V. Kulkarni, R. P. Mason, A. Constantinescu, S. Aravind, N. Goomer and P. P. Antich, 6-Fluoropyridoxol: a novel probe of cellular pH using 19F NMR spectroscopy, FEBS Lett., 1994, 349, 234–238 CrossRef CAS PubMed.
- R. P. Mason, Transmembrane pH gradients in vivo: measurements using fluorinated vitamin B6 derivatives, Curr. Med. Chem., 1999, 6, 481–499 CrossRef CAS PubMed.
- S. Hunjan, R. P. Mason, V. D. Mehta, P. V. Kulkarni, S. Aravind, V. Arora and P. P. Antich, Simultaneous intracellular and extracellular pH measurement in the heart by 19F NMR of 6-fluoropyridoxol, Magn. Reson. Med., 1998, 39, 551–556 CrossRef CAS PubMed.
- O. Maxouri, Z. Bodalal, M. Daal, S. Rostami, I. Rodriguez, L. Akkari, M. Srinivas, R. Bernards and R. Beets-Tan, How to 19F MRI: applications, technique, and getting started, BJR Open, 2023, 5, 20230019 Search PubMed.
- G. E. Soto, Z. Zhu, J. L. Evelhoch and J. J. Ackerman, Tumor 31P NMR pH measurements in vivo: a comparison of inorganic phosphate and intracellular 2-deoxyglucose-6-phosphate as pHnmr indicators in murine radiation-induced fibrosarcoma-1, Magn. Reson. Med., 1996, 36, 698–704 CrossRef CAS PubMed.
- D. A. Beauregard, D. Parker and K. M. Brindle, Relaxation-Based mapping of tumour pH, International Society for Magnetic Resonance in Medicine Conference Abstract, 1998 Search PubMed.
- C. F. Chang and C. C. Lin, Current concepts of contrast-induced nephropathy: a brief review, J. Chin. Med. Assoc., 2013, 76, 673–681 CrossRef PubMed.
- E. Kanal and M. F. Tweedle, Residual or retained gadolinium: practical implications for radiologists and our patients, Radiology, 2015, 275, 630–634 CrossRef PubMed.
- T. Grobner, Gadolinium–a specific trigger for the development of nephrogenic fibrosing dermopathy and nephrogenic systemic fibrosis?, Nephrol., Dial., Transplant., 2006, 21, 1104–1108 CrossRef CAS PubMed.
- E. P. Rahrmann, D. Shorthouse, A. Jassim, L. P. Hu, M. Ortiz, B. Mahler-Araujo, P. Vogel, M. Paez-Ribes, A. Fatemi, G. J. Hannon, R. Iyer, J. A. Blundon, F. C. Lourenco, J. Kay, R. M. Nazarian, B. A. Hall, S. S. Zakharenko, D. J. Winton, L. Zhu and R. J. Gilbertson, The NALCN channel regulates metastasis and nonmalignant cell dissemination, Nat. Genet., 2022, 54, 1827–1838 CrossRef CAS PubMed.
- J. B. Hovener, A. N. Pravdivtsev, B. Kidd, C. R. Bowers, S. Gloggler, K. V. Kovtunov, M. Plaumann, R. Katz-Brull, K. Buckenmaier, A. Jerschow, F. Reineri, T. Theis, R. V. Shchepin, S. Wagner, P. Bhattacharya, N. M. Zacharias and E. Y. Chekmenev, Parahydrogen-Based Hyperpolarization for Biomedicine, Angew. Chem., Int. Ed., 2018, 57, 11140–11162 CrossRef PubMed.
- T. Hune, S. Mamone, A. B. Schmidt, I. Mahú, N. D'Apolito, D. Wiedermann, J. Brüning and S. Glöggler, Hyperpolarized Multi-organ Spectroscopy of Liver and Brain Using 1-13C-Pyruvate Enhanced via Parahydrogen, Appl. Magn. Reson., 2023, 54, 1283–1295 CrossRef CAS.
- G. Stevanato, Y. Ding, S. Mamone, A. P. Jagtap, S. Korchak and S. Gloggler, Real-Time Pyruvate Chemical Conversion Monitoring Enabled by PHIP, J. Am. Chem. Soc., 2023, 145, 5864–5871 CrossRef CAS PubMed.
- S. Mamone, A. P. Jagtap, S. Korchak, Y. Ding, S. Sternkopf and S. Gloggler, A Field-Independent Method for the Rapid Generation of Hyperpolarized [1-(13) C]Pyruvate in Clean Water Solutions for Biomedical Applications, Angew. Chem., Int. Ed., 2022, 61, e202206298 CrossRef CAS PubMed.
- J. H. Ardenkjaer-Larsen, S. Bowen, J. R. Petersen, O. Rybalko, M. S. Vinding, M. Ullisch and N. C. Nielsen, Cryogen-free dissolution dynamic nuclear polarization polarizer operating at 3.35 T, 6.70 T, and 10.1 T, Magn. Reson. Med., 2019, 81, 2184–2194 CrossRef CAS PubMed.
- J. H. Ardenkjaer-Larsen, B. Fridlund, A. Gram, G. Hansson, L. Hansson, M. H. Lerche, R. Servin, M. Thaning and K. Golman, Increase in signal-to-noise ratio of > 10,000 times in liquid-state NMR, Proc. Natl. Acad. Sci. U. S. A., 2003, 100, 10158–10163 CrossRef CAS PubMed.
- F. A. Gallagher, M. I. Kettunen, S. E. Day, D. E. Hu, J. H. Ardenkjaer-Larsen, R. Zandt, P. R. Jensen, M. Karlsson, K. Golman, M. H. Lerche and K. M. Brindle, Magnetic resonance imaging of pH in vivo using hyperpolarized 13C-labelled bicarbonate, Nature, 2008, 453, 940–943 CrossRef CAS PubMed.
- N. Drachman, S. Kadlecek, M. Pourfathi, Y. Xin, H. Profka and R. Rizi, In vivo pH mapping of injured lungs using hyperpolarized [1-(13) C]pyruvate, Magn. Reson. Med., 2017, 78, 1121–1130 CrossRef CAS PubMed.
- D. E. Korenchan, R. R. Flavell, C. Baligand, R. Sriram, K. Neumann, S. Sukumar, H. VanBrocklin, D. B. Vigneron, D. M. Wilson and J. Kurhanewicz, Dynamic nuclear polarization of biocompatible (13)C-enriched carbonates for in vivo pH imaging, Chem. Commun., 2016, 52, 3030–3033 RSC.
- D. E. Korenchan, R. Bok, R. Sriram, K. Liu, R. D. Santos, H. Qin, I. Lobach, N. Korn, D. M. Wilson, J. Kurhanewicz and R. R. Flavell, Hyperpolarized in vivo pH imaging reveals grade-dependent acidification in prostate cancer, Oncotarget, 2019, 10, 6096–6110 CrossRef PubMed.
- N. Bogh, E. S. S. Hansen, C. O. Mariager, L. B. Bertelsen, S. Ringgaard and C. Laustsen, Cardiac pH-Imaging With Hyperpolarized
MRI, Front. Cardiovasc. Med., 2020, 7, 603674 CrossRef PubMed.
-
M. A. M. Alixander S Khan, J. D. Kaggie, I. Horvat-Menih, T. Matys, R. F. Schulte, M. J. Locke, A. Grimmer, P. Wodtke, E. Latimer, A. Frary, M. J. Graves and F. A. Gallagher, Measuring extracellular human brain pH and amino acid metabolism with hyperpolarized [1-13C]pyruvate, 2023, DOI:10.1101/2023.03.23.23287579.
- H. de Maissin, P. R. Gross, O. Mohiuddin, M. Weigt, L. Nagel, M. Herzog, Z. Wang, R. Willing, W. Reichardt, M. Pichotka, L. Hess, T. Reinheckel, H. J. Jessen, R. Zeiser, M. Bock, D. von Elverfeldt, M. Zaitsev, S. Korchak, S. Gloggler, J. B. Hovener, E. Y. Chekmenev, F. Schilling, S. Knecht and A. B. Schmidt, In Vivo Metabolic Imaging of [1-(13) C]Pyruvate-d(3) Hyperpolarized By Reversible Exchange With Parahydrogen, Angew. Chem., Int. Ed., 2023, 62, e202306654 CrossRef CAS PubMed.
- F. Reineri, T. Boi and S. Aime, ParaHydrogen Induced Polarization of 13C carboxylate resonance in acetate and pyruvate, Nat. Commun., 2015, 6, 5858 CrossRef CAS PubMed.
- S. Duwel, C. Hundshammer, M. Gersch, B. Feuerecker, K. Steiger, A. Buck, A. Walch, A. Haase, S. J. Glaser, M. Schwaiger and F. Schilling, Imaging of pH in vivo using hyperpolarized (13)C-labelled zymonic acid, Nat. Commun., 2017, 8, 15126 CrossRef PubMed.
- C. Hundshammer, S. Duwel, S. S. Kocher, M. Gersch, B. Feuerecker, C. Scheurer, A. Haase, S. J. Glaser, M. Schwaiger and F. Schilling, Deuteration of Hyperpolarized (13) C-Labeled Zymonic Acid Enables Sensitivity-Enhanced Dynamic MRI of pH, ChemPhysChem, 2017, 18, 2422–2425 CrossRef CAS PubMed.
- D. E. Korenchan, C. Taglang, C. von Morze, J. E. Blecha, J. W. Gordon, R. Sriram, P. E. Z. Larson, D. B. Vigneron, H. F. VanBrocklin, J. Kurhanewicz, D. M. Wilson and R. R. Flavell, Dicarboxylic acids as pH sensors for hyperpolarized (13)C magnetic resonance spectroscopic imaging, Analyst, 2017, 142, 1429–1433 RSC.
- T. Nishihara, Y. Kameyama, H. Nonaka, Y. Takakusagi, F. Hyodo, K. Ichikawa and S. Sando, A Strategy to Design Hyperpolarized (13) C Magnetic Resonance Probes Using [1-(13) C]alpha-Amino Acid as a Scaffold Structure, Chem.– Asian J., 2017, 12, 949–953 CrossRef CAS PubMed.
- C. Hundshammer, S. Duwel, D. Ruseckas, G. Topping, P. Dzien, C. Muller, B. Feuerecker, J. B. Hovener, A. Haase, M. Schwaiger, S. J. Glaser and F. Schilling, Hyperpolarized Amino Acid Derivatives as Multivalent Magnetic Resonance pH Sensor Molecules, Sensors, 2018, 18, 600 CrossRef PubMed.
- C. Mu, X. Liu, Y. Kim, A. Riselli, D. E. Korenchan, R. A. Bok, R. Delos Santos, R. Sriram, H. Qin, H. Nguyen, J. W. Gordon, J. Slater, P. E. Z. Larson, D. B. Vigneron, J. Kurhanewicz, D. M. Wilson and R. R. Flavell, Clinically Translatable Hyperpolarized (13)C Bicarbonate pH Imaging Method for Use in Prostate Cancer, ACS Sens., 2023, 8, 4042–4054 CrossRef CAS PubMed.
- M. Grashei, C. Hundshammer, F. H. A. van Heijster, G. J. Topping and F. Schilling, pH Dependence of T(2) for Hyperpolarizable (13)C-Labelled Small Molecules Enables Spatially Resolved
pH Measurement by Magnetic Resonance Imaging, Pharmaceuticals, 2021, 14, 327 CrossRef CAS PubMed.
- W. Jiang, L. Lumata, W. Chen, S. Zhang, Z. Kovacs, A. D. Sherry and C. Khemtong, Hyperpolarized 15N-pyridine derivatives as pH-sensitive MRI agents, Sci. Rep., 2015, 5, 9104 CrossRef PubMed.
- D. A. Barskiy, K. V. Kovtunov, I. V. Koptyug, P. He, K. A. Groome, Q. A. Best, F. Shi, B. M. Goodson, R. V. Shchepin, A. M. Coffey, K. W. Waddell and E. Y. Chekmenev, The feasibility of formation and kinetics of NMR signal amplification by reversible exchange (SABRE) at high magnetic field (9.4 T), J. Am. Chem. Soc., 2014, 136, 3322–3325 CrossRef CAS PubMed.
- Y. Ding, S. Korchak, S. Mamone, A. P. Jagtap, G. Stevanato, S. Sternkopf, D. Moll, H. Schroeder, S. Becker, A. Fischer, E. Gerhardt, T. F. Outeiro, F. Opazo, C. Griesinger and S. Glöggler, Rapidly Signal–enhanced Metabolites for Atomic Scale Monitoring of Living Cells with Magnetic Resonance, Chem.: Methods, 2022, 2, e202200023 CAS.
- E. Cavallari, C. Carrera, S. Aime and F. Reineri, Studies to enhance the hyperpolarization level in PHIP-SAH-produced C13-pyruvate, J. Magn. Reson., 2018, 289, 12–17 CrossRef CAS PubMed.
- S. Korchak, S. Mamone and S. Gloggler, Over 50% (1)H and (13)C Polarization for Generating Hyperpolarized Metabolites-A para-Hydrogen Approach, ChemistryOpen, 2018, 7, 672–676 CrossRef CAS PubMed.
- Y. Ding, G. Stevanato, F. von Bonin, D. Kube and S. Gloggler, Real-time cell metabolism assessed repeatedly on the same cells via para-hydrogen induced polarization, Chem. Sci., 2023, 14, 7642–7647 RSC.
- S. Knecht, J. W. Blanchard, D. Barskiy, E. Cavallari, L. Dagys, E. Van Dyke, M. Tsukanov, B. Bliemel, K. Munnemann, S. Aime, F. Reineri, M. H. Levitt, G. Buntkowsky, A. Pines, P. Blumler, D. Budker and J. Eills, Rapid hyperpolarization and purification of the metabolite fumarate in aqueous solution, Proc. Natl. Acad. Sci. U. S. A., 2021, 118, e2025383118 CrossRef CAS PubMed.
- S. Korchak, S. Yang, S. Mamone and S. Gloggler, Pulsed Magnetic Resonance to Signal-Enhance Metabolites within Seconds by utilizing para-Hydrogen, ChemistryOpen, 2018, 7, 344–348 CrossRef CAS PubMed.
- S. Korchak, M. Emondts, S. Mamone, B. Blumich and S. Gloggler, Production of highly concentrated and hyperpolarized metabolites within seconds in high and low magnetic fields, Phys. Chem. Chem. Phys., 2019, 21, 22849–22856 RSC.
- L. Dagys, A. P. Jagtap, S. Korchak, S. Mamone, P. Saul, M. H. Levitt and S. Gloggler, Nuclear hyperpolarization of (1-(13)C)-pyruvate in aqueous solution by proton-relayed side-arm hydrogenation, Analyst, 2021, 146, 1772–1778 RSC.
- L. Kaltschnee, A. P. Jagtap, J. McCormick, S. Wagner, L. S. Bouchard, M. Utz, C. Griesinger and S. Gloggler, Hyperpolarization of Amino Acids in Water Utilizing Parahydrogen on a Rhodium Nanocatalyst, Chemistry, 2019, 25, 11031–11035 CrossRef CAS PubMed.
- T. Hune, S. Mamone, H. Schroeder, A. P. Jagtap, S. Sternkopf, G. Stevanato, S. Korchak, C. Fokken, C. A. Muller, A. B. Schmidt, D. Becker and S. Gloggler, Metabolic Tumor Imaging with Rapidly Signal-Enhanced 1-(13) C-Pyruvate-d(3), ChemPhysChem, 2023, 24, e202200615 CrossRef CAS PubMed.
- V. A. Guarino, W. M. Oldham, J. Loscalzo and Y. Y. Zhang, Reaction rate of pyruvate and hydrogen peroxide: assessing antioxidant capacity of pyruvate under biological conditions, Sci. Rep., 2019, 9, 19568 CrossRef CAS PubMed.
- A. P. Jagtap, S. Mamone and S. Gloggler, Molecular precursors to produce para-hydrogen enhanced metabolites at any field, Magn. Reson. Chem., 2023, 61, 674–680 CrossRef CAS PubMed.
- J. W. Gordon, S. B. Fain and I. J. Rowland, Effect of lanthanide ions on dynamic nuclear polarization enhancement and liquid-state T1 relaxation, Magn. Reson. Med., 2012, 68, 1949–1954 CrossRef CAS PubMed.
- E. Cavallari, C. Carrera, M. Sorge, G. Bonne, A. Muchir, S. Aime and F. Reineri, The (13)C hyperpolarized pyruvate generated by ParaHydrogen detects the response of the heart to altered metabolism in real time, Sci. Rep., 2018, 8, 8366 CrossRef PubMed.
- B. J. Tickner, P. J. Rayner and S. B. Duckett, Using SABRE Hyperpolarized (13)C NMR Spectroscopy to Interrogate Organic Transformations of Pyruvate, Anal. Chem., 2020, 92, 9095–9103 CrossRef CAS PubMed.
- R. L. Bailone, H. C. S. Fukushima, L. K. de Aguiar and R. C. Borra, Calcium Chloride Toxicology for Food Safety Assessment Using Zebrafish (Danio rerio) Embryos, Comp. Med., 2022, 72, 342–348 CrossRef CAS PubMed.
-
A. L. Nelson and L. Porter, StatPearls, Treasure Island (FL), 2024 Search PubMed.
- D. J. Kushner, A. Baker and T. G. Dunstall, Pharmacological uses and perspectives of heavy water and deuterated compounds, Can. J. Physiol. Pharmacol., 1999, 77, 79–88 CrossRef CAS PubMed.
- C. Asmus, O. Mozziconacci and C. Schoneich, Low-temperature NMR characterization of reaction of sodium pyruvate with hydrogen peroxide, J. Phys. Chem. A, 2015, 119, 966–977 CrossRef CAS PubMed.
-
S. L. P. Gianturco, L. Laura, K. D. Storm, S. Y. Yoon, V. Melissa and A. N. Mattingly, Hydrogen peroxide: Summary Report, University of Maryland Center of Excellence in Regulatory Science and Innovation (M-CERSI) and University of Maryland School of Pharmacy, 2020 Search PubMed.
-
M. Abdollahi and A. Hosseini, Hydrogen Peroxide, in Encyclopedia of Toxicology, ed. P. Wexler, Elsevier Inc., Academic Press, 3rd edn, 2014, vol. 2, pp. 967–970 Search PubMed.
- Medscape, https://reference.medscape.com/drug/cacl-or-cacl-2-calcium-chloride-344432.
- N. C. I. thesaurus, Calcium Chloride (Code C28901), National Cancer Institute, 2024 Search PubMed.
- E. Anitua, R. Prado, M. Troya, M. Zalduendo, M. de la Fuente, A. Pino, F. Muruzabal and G. Orive, Implementation of a more physiological plasma rich in growth factor (PRGF) protocol: Anticoagulant removal and reduction in activator concentration, Platelets, 2016, 27, 459–466 CrossRef CAS PubMed.
- L. P. Msezane, M. H. Katz, O. N. Gofrit, A. L. Shalhav and K. C. Zorn, Hemostatic agents and instruments in laparoscopic renal surgery, J. Endourol., 2008, 22, 403–408 CrossRef PubMed.
- D. W. Lee and B. Cohan, Refractory cardiogenic shock and complete heart block after verapamil SR and metoprolol treatment. A case report, Angiology, 1995, 46, 517–519 CrossRef CAS PubMed.
- K. Kawai, B. J. Larson, H. Ishise, A. L. Carre, S. Nishimoto, M. Longaker and H. P. Lorenz, Calcium-based nanoparticles accelerate skin wound healing, PLoS One, 2011, 6, e27106 CrossRef CAS PubMed.
- U. Koellisch, C. Laustsen, T. S. Norlinger, J. A. Ostergaard, A. Flyvbjerg, C. V. Gringeri, M. I. Menzel, R. F. Schulte, A. Haase and H. Stodkilde-Jorgensen, Investigation of metabolic changes in STZ-induced diabetic rats with hyperpolarized [1-13C]acetate, Physiol. Rep., 2015, 3, e12474 CrossRef PubMed.
- A. Flori, M. Liserani, F. Frijia, G. Giovannetti, V. Lionetti, V. Casieri, V. Positano, G. D. Aquaro, F. A. Recchia, M. F. Santarelli, L. Landini, J. H. Ardenkjaer-Larsen and L. Menichetti, Real-time cardiac metabolism assessed with hyperpolarized [1-(13) C]acetate in a large-animal model, Contrast Media Mol. Imaging, 2015, 10, 194–202 CrossRef CAS PubMed.
- E. F. R. Mikkelsen, C. O. Mariager, T. Norlinger, H. Qi, R. F. Schulte, S. Jakobsen, J. Frokiaer, M. Pedersen, H. Stodkilde-Jorgensen and C. Laustsen, Hyperpolarized [1-(13)C]-acetate Renal Metabolic Clearance Rate Mapping, Sci. Rep., 2017, 7, 16002 CrossRef PubMed.
- J. Steinhauser, P. Wespi, G. Kwiatkowski and S. Kozerke, Production of highly polarized [1-(13) C]acetate by rapid decarboxylation of [2-(13) C]pyruvate - application to hyperpolarized cardiac spectroscopy and imaging, Magn. Reson. Med., 2019, 82, 1140–1149 CrossRef CAS PubMed.
- V. Ruiz-Rodado, J. R. Brender, M. K. Cherukuri, M. R. Gilbert and M. Larion, Magnetic resonance spectroscopy for the study of cns malignancies, Prog. Nucl. Magn. Reson. Spectrosc., 2021, 122, 23–41 CrossRef CAS PubMed.
- C. A. Pfortmueller and E. Fleischmann, Acetate-buffered crystalloid fluids: Current knowledge, a systematic review, J. Crit. Care, 2016, 35, 96–104 CrossRef CAS PubMed.
- B. Ergin, A. Kapucu, P. Guerci and C. Ince, The role of bicarbonate precursors in balanced fluids during haemorrhagic shock with and without compromised liver function, Br. J. Anaesth., 2016, 117, 521–528 CrossRef CAS PubMed.
- K. L. Ellekjaer, A. Perner, M. M. Jensen and M. H. Moller, Lactate versus acetate buffered intravenous crystalloid solutions: a scoping review, Br. J. Anaesth., 2020, 125, 693–703 CrossRef CAS PubMed.
-
B. M. Mitruka and H. M. Rawnsley, Clinical, biochemical and hematological reference values in normal experimental animals and normal humans, Masson Publishing, New York, 1981 Search PubMed.
-
J. E. Harkness and J. E. Wagner, Biology and husbandry, Lea &Febiger, Philadelphia, 1989 Search PubMed.
-
J. N. Butler, Carbon Dioxide Equilibria and Their Applications, New York, 1 edn, 1991 Search PubMed.
- M. Grashei, P. Wodtke, J. G. Skinner, S. Suhnel, N. Setzer, T. Metzler, S. Gulde, M. Park, D. Witt, H. Mohr, C. Hundshammer, N. Strittmatter, N. S. Pellegata, K. Steiger and F. Schilling, Simultaneous magnetic resonance imaging of pH, perfusion and renal filtration using hyperpolarized (13)C-labelled Z-OMPD, Nat. Commun., 2023, 14, 5060 CrossRef CAS PubMed.
|
This journal is © The Royal Society of Chemistry 2024 |
Click here to see how this site uses Cookies. View our privacy policy here.