DOI:
10.1039/D4AN00559G
(Paper)
Analyst, 2024,
149, 3522-3529
Super-assembled periodic mesoporous organosilica membranes with hierarchical channels for efficient glutathione sensing†
Received
12th April 2024
, Accepted 8th May 2024
First published on 24th May 2024
Abstract
Bioinspired nanochannel-based sensors have elicited significant interest because of their excellent sensing performance, and robust mechanical and tunable chemical properties. However, the existing designs face limitations due to material constraints, which hamper broader application possibilities. Herein, a heteromembrane system composed of a periodic mesoporous organosilica (PMO) layer with three-dimensional (3D) network nanochannels is constructed for glutathione (GSH) detection. The unique hierarchical pore architecture provides a large surface area, abundant reaction sites and plentiful interconnected pathways for rapid ionic transport, contributing to efficient and sensitive detection. Moreover, the thioether groups in nanochannels can be selectively cleaved by GSH to generate hydrophilic thiol groups. Benefiting from the increased hydrophilic surface, the proposed sensor achieves efficient GSH detection with a detection limit of 1.2 μM by monitoring the transmembrane ionic current and shows good recovery ranges in fetal bovine serum sample detection. This work paves an avenue for designing and fabricating nanofluidic sensing systems for practical and biosensing applications.
Introduction
Glutathione (GSH), a vital cellular thiol, plays a critical role in physiological and pathological processes, including redox homeostasis and signal transduction.1,2 Nevertheless, GSH deficiency can induce oxidative stress in cells and other diseases such as Parkinson's and HIV infection.3–7 Additionally, the GSH concentration level in tumor tissue is higher than that in normal tissue (at a millimolar level).8,9 Thereby, the detection of GSH is essential for disease diagnosis and treatment. Until now, researchers have developed several high-performance analytical methods and sensors for the detection of ions, small molecules and biomolecules, such as fluorescence methods, and electrode-based electrochemical sensors.10–20 These techniques and methods overcome the limitations of traditional analytical methods and provide insights into molecular detection. However, some existing analytical methods for GSH detection still require complicated labeling processes, which are expensive and time-consuming. Moreover, the limited stability of some enzyme-based electrochemical biosensors hinders their broader application. Therefore, it is highly desirable to develop simple, stable and efficient systems for the precise determination of GSH.
Biological ion channels are crucial in regulating mass transport across cell membranes, selectively responding to specific stimuli, biomolecules or ions.21–23 Drawing inspiration from biological channels, artificial nanochannels have attracted wide interest due to their robust mechanical and controllable chemical properties.24–26 The surface properties of these nanochannels, such as wettability and charge, can be modulated by external stimuli, such as pH, light, molecules and electric fields. This modulation, in turn, affects ion permeability and transport.21,27–30 This intriguing characteristic positions them for diverse applications, from sensors to controlled drug delivery systems.27,31,32 Notably, owing to the striking features of the confined environment at the nanoscale and high-throughput screening, the nanochannels can be utilized as sensors to monitor the target substances through the changes of ionic current signals with high sensitivity and specificity.33–35 Thus, the nanofluidic sensors have great potential for the label-free and high-performance detection of GSH.
Recently, numerous materials, including polyethylene terephthalate, mesoporous silica, and graphene oxide, have been utilized to develop smart nanochannel-based sensors.24,36–38 Despite this diversity, the singular functional groups offered by most materials often fall short of the requirements for advanced applications, necessitating post-modification. This additional step can introduce complexities, a risk of channel blockage, incomplete modification, and degradation of surface modifications, all of which can lead to inefficient detection and hinder the progress of nanofluidic sensor development.39,40 Therefore, there is still a significant demand for developing straightforward and effective strategies to engineer stable and efficient nanochannel-based sensors. Such advancements could significantly broaden the scope of nanofluidic sensing techniques and enhance disease diagnosis.
Combining the advantages of organic and inorganic components, the periodic mesoporous organosilica (PMO) materials with organic-group-incorporated frameworks and mechanical stability have found broad applications in catalysis, absorbents, enzyme immobilization, and so on.41–46 The functional organic groups can be easily and directly introduced in the predetermined framework via covalent bonds during the synthetic process without post-modification, and bring materials with high organic matter loading capability and desirable properties such as hydrophilicity/hydrophobicity.39,47 In contrast to mesoporous silica materials modified with organic groups, the organic groups in PMO are distributed homogeneously and completely within the pore walls, crucially without blocking the pore channels. This feature ensures unimpeded flow through the channels, enhancing the material's effectiveness for various applications.39 Regardless of this advanced progress, to the best of our knowledge, there have been few reports about constructing a nanofluidic system platform based on PMO. To gain an excellent sensing performance, high ions flux and channels at the nanoscale are necessary for nanofluidic sensors.33,48 As part of the mesoporous material family, PMO boasts abundant nanochannels, a large surface area, and a highly ordered mesoporous structure.39 These characteristics position PMO as a promising candidate for constructing nanofluidic devices.
Here, we demonstrate a hierarchically porous heteromembrane system (PMO/AAO) for GSH detection (Fig. 1). The heterogeneous membrane composed of a thioether-bridged yolk–shell PMO packing layer and anode alumina oxide (AAO) support was constructed by a super-assembly method without further modification steps. Besides the gap channels formed by closely packed PMO particles (PMOs), the rich mesoporous channels and interior cavities provided three-dimensional (3D) connected nanochannels and abundant recognition sites, enabling high ion flux and sensitive detection. Meanwhile, GSH could induce the redox-responsive breaking of the disulfide bonds (S–S) in organosilica frameworks, and generate thiol groups (–SH). Upon detection, the membrane became more hydrophilic and this ultimately led to the ionic current variation. As proof-of-concept demonstrations, the PMO/AAO was used to detect GSH in a fetal bovine serum (FBS) sample. This research is expected to trigger the construction of other PMO membrane systems with different organic groups, opening up an avenue for sensitive nanofluidic sensing.
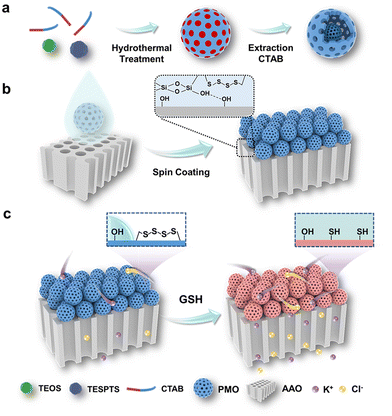 |
| Fig. 1 Schematic illustration of (a and b) the preparation process of the PMO/AAO membrane via the super-assembly method and (c) the responsive process. | |
Experimental
Materials
Tetraethyl orthosilicate (TEOS), cetyltrimethylammonium bromide (CTAB), (5,5′-dithio-bis-[2-nitrobenzoic acid]) (DTNB), DOX and L-lysine (L-Lys) were purchased from Aladdin. 1,4-Bis(triethoxysily)propane tetrasulfide (TESPTS), poly(vinylidene fluoride-co-hexafluoropropylene) (PVDF–HFP, average MW ≈ 455
000, average Mn ≈ 110
000) and L-arginine (L-Arg) were obtained from Sigma-Aldrich. Concentrated ammonium hydroxide (28 wt%), L-cysteine (L-Cys), hydrochloric acid (HCl), ethanol, and acetone were received from Shanghai Chemical Corp. Potassium chloride (KCl), L-glutamic acid (L-Glu), and glycine (Gly) were acquired from Macklin. GSH was obtained from RHAWN. Phosphate-buffered saline (PBS solution, pH = 7.2) was purchased from Beijing Labgic Technology Co., Ltd. Ethylene diamine tetraacetic acid (EDTA) was received from Energy Chemical Corp. FBS was received from Thermo Fisher Scientific.
Characterization methods
Scanning electron microscopy (SEM) was carried out using a Shimadzu S-4800 microscope. Transmission electron microscopy (TEM) was performed using a Hitachi HT-7700 microscope. Elemental mapping images were collected using a Tecnai G2 F20 S-Twin scanning transmission electron microscope. Nitrogen (N2) adsorption/desorption isotherms were measured using a Micromeritics Tristar II 3020 analyzer. X-ray photoelectron spectroscopy (XPS) data were collected using PHI5000C and PHI5300 X-ray photoelectron spectrometers. The water contact angle (CA) data were recorded using a DCAT21 and OCA 20 system (Germany). Fourier transform infrared (FT-IR) spectra were obtained from a Nicolet iS10 of ThermoFisher spectrometer. The solid-state NMR experiment was carried out using a Bruker AVANCE III 400WB spectrometer. A Lambda 35 ultraviolet-visible (UV-vis) spectrophotometer was used for measuring the UV-vis spectra.
Synthesis of PMOs
The thioether-bridged PMOs were prepared via a one-step hydrothermal treatment method.41,49 Typically, CTAB (0.16 g) was added to the mixture solution consisting of ammonia aqueous solution (1 mL), ethanol (30 mL) and water (75 mL). After heating to 35 °C, TESPTS (0.1 mL) and TEOS (0.25 mL) were added under vigorous stirring, and the obtained solution was continually stirred for 24 h. Collected by centrifugation, the product was washed with ethanol, dispersed in water (30 mL) and hydrothermally treated at 150 °C for 12 h. Finally, the surfactant was removed by solvent extraction to obtain the thioether-bridged PMOs.
Synthesis of the PMO/AAO membrane
The PMO/AAO membrane was prepared by a super-assembly method. First, as-prepared PMOs (6 mg) were dissolved in acetone (500 μL) solution containing PVDF–HFP binder (15 mg) by sonicating for 30 min. Afterwards, the mixture solution was spin-coated onto AAO membrane (pore size 60 nm) at 500 rpm. Finally, the PMO/AAO membrane with 40 wt% (mPMOs
:
mPVDF–HFP = 40 wt%) PMOs was heated at 90 °C under vacuum for 12 h. To obtain PMO/AAO membranes with various PMO contents (25 wt%, 30 wt%, 35 wt%, 45 wt%), different amounts of PMOs were added in the first step.
Electrochemical measurements
The electrochemical performance tests were carried out using a Keithley 6487 picoammeter. The membranes were set between two custom-made electrochemical cells, and clamped between two silicon wafers. A pair of Ag/AgCl electrodes was settled in half-cells respectively to apply potential with the anode setting on the PMO side. The effective testing area is 0.03 mm2. The current data were obtained by five parallel trials (n = 5).
Detection of GSH
The membranes were immersed in PBS solution (pH = 7.2) containing different concentrations of GSH for 60 min at 25 °C. Then, the membrane was washed with water 3 times for further electrochemical measurements. The electrolyte solution is KCl (0.1 M). For Ellman's test: the PMOs (0.1 mg mL−1) were dispersed in PBS solution with or without GSH (10 mM). After incubation for 60 min, the precipitates were collected by centrifugation. Afterwards, the precipitates were washed and reacted with 5 mM DTNB in PBS/EDTA (10 mL, pH = 8) solution for 10 min. The supernatants were obtained by centrifugation and tested by UV-vis spectroscopy. For CA measurements, the PMO/AAO was treated with 10 mM GSH for 60 min. For the determination of GSH in FBS sample, the FBS sample was diluted 1000 times. The spiked samples were obtained by adding GSH with different concentrations (0.05 and 0.1 mM) to the diluted FBS solution. The detection procedure for GSH in the FBS sample is similar to that in the PBS solution.
Results and discussion
Preparation and characterization of the PMO/AAO membranes
In this study, the thioether-bridged PMO yolk–shell particles were synthesized via one-step hydrothermal treatment (Fig. 1a).41,49 TEM and SEM images revealed that the PMO spheres had a uniform diameter of about 180 nm (Fig. 2a, S1 and S2†). The elemental mappings clearly exhibited that the elements of S, Si and O were homogeneously distributed in the PMO framework (Fig. 2b). In addition, the composition of the mesoporous nanoparticles was analyzed by FT-IR spectroscopy and 29Si NMR. As shown in Fig. S3,† the peaks at 2930, 1449, and 1409 cm−1 were assigned to the C–H vibration, and the peak at 686 cm−1 was ascribed to the C–S vibration, suggesting that the silane TESPTS had condensed in PMO.49,50 Additionally, the 29Si NMR spectrum displayed three signals at −67, −103 and −111 ppm belonging to T3 (C–Si(OSi)3), Q3 (Si(OSi)3(OX)), and Q4 (Si(OSi)4) species (Fig. S4†), indicating the high condensation degree of the PMO nanoparticles.49,51
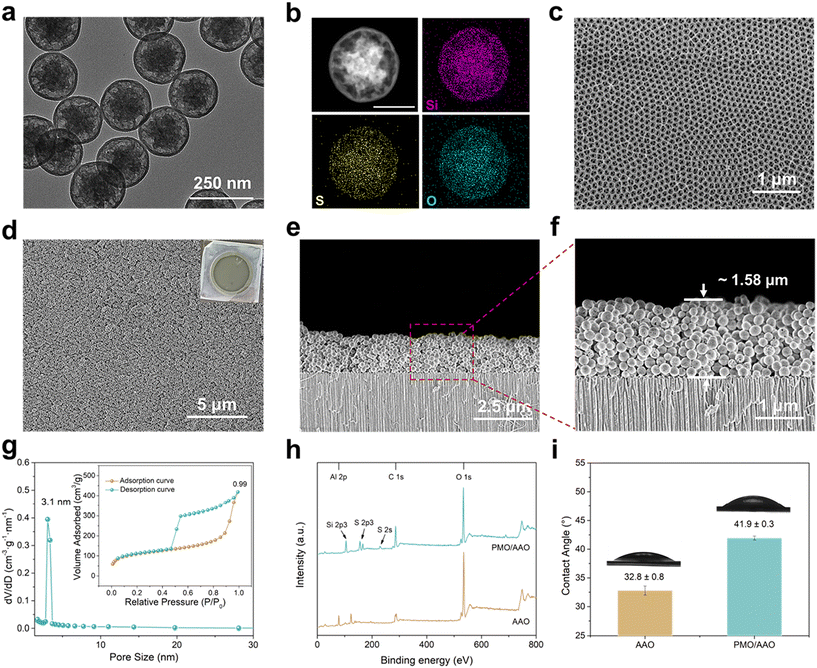 |
| Fig. 2 Characterization of the PMO/AAO membrane. (a) TEM image of PMOs. (b) Corresponding EDS elemental mapping images of PMOs (scale bar is 100 nm). Surface SEM image of (c) AAO, (d) PMO/AAO membrane (the inset is the optical photograph). (e) Cross-sectional SEM image and (f) a magnified view of the PMO/AAO membrane. (g) Pore size distribution of PMOs; the inset is the N2 adsorption/desorption isotherms of PMOs. (h) XPS spectra for AAO and PMO/AAO. (i) CAs of AAO and PMO/AAO, n = 3 replicates. | |
Furthermore, PMOs can serve as the building units to fabricate a hybrid membrane via the super-assembly method (Fig. 1b). The PMOs were spin-coated on the surface of AAO support with the assistance of a PVDF–HFP binder to ensure the mechanical stability of the hybrid membrane. As a result of doping PMOs, the membrane transformed from transparent to white (Fig. S5†). The morphologies of the naked AAO and hybrid membranes are shown in the SEM images (Fig. 2c–f and S6†). Meanwhile, Fig. 2d shows the top-view SEM image of as-assembled PMO/AAO membrane, where the AAO was fully coated with a crack-free PMO layer. From the SEM cross-sectional images (Fig. 2e and f), the PMO layer with a thickness of about 1.58 μm was densely assembled on the 60 μm-thick AAO. In addition, the thickness of the PMO layer can be regulated by varying the PMO contents (Experimental section). The N2 adsorption/desorption isotherms of PMOs depicted clearly typical type-IV behavior with a sharp capillary condensation step at p/p0 = 0.5–1.0, indicating a uniform mesoporous structure (Fig. 2g). The Brunauer–Emmett–Teller (BET) surface area of PMOs was calculated to be 377.25 ± 6.25 m2 g−1, indicating the presence of abundant nanochannels and reaction sites. The pore size distribution of PMOs was calculated as a mean value of 3.1 nm based on the Barrett–Joyner–Halenda (BJH) model, which can allow the efficient penetration of ions into the interior cavities.52 These above results suggest that the PMO/AAO has a 3D interconnected network with hierarchical pores across the membrane.53,54 Subsequently, AAO and PMO/AAO with 40 wt% PMOs were further characterized. The XPS spectra showed characteristic peaks of S 2s, S 2p3 and Si 2p3 in the PMO/AAO membrane, which were not observed in the AAO membrane (Fig. 2h). Additionally, with the assembly of PMOs on AAO, the CAs increased from 32.8 ± 0.8° to 41.9 ± 0.3°, indicating the less hydrophilic surface (Fig. 2i). This may be ascribed to the introduced hydrophobic S–S groups. These results demonstrate the successful incorporation of PMOs on AAO. The special 3D network structure provides plentiful interconnected nanochannels and enlarges the contact area between the disulfide bonds and target molecules. This feature endows PMO/AAO with high ion flux and enables sensitive detection.55
Ion transport performance of the PMO/AAO membrane
The transmembrane ionic transport properties of the PMO/AAO membrane were studied by measuring the current–voltage (I–V) response. As shown in Fig. 3a, the membrane was set between two homemade conductivity cells. A pair of Ag/AgCl electrodes was immersed in KCl electrolyte to apply the transmembrane voltage. On account of the hierarchical pore structure, the ions can not only pass through interstitial voids, but also migrate through the mesochannels and hollow interiors of the PMO layer. Therefore, such a structure may result in rapid ion flux and large surface area for further sensing application. The ionic current and conductance of the PMO/AAO membrane with respect to the KCl concentration were investigated, and the results are shown in Fig. 3b and c. The transmembrane ionic conductance exhibits a linear relationship at high concentration owing to the bulk diffusion behavior. However, the conductance apparently deviates upon decreasing the KCl concentration (<0.1 M), suggesting a surface-charge-governed ion transport behavior.56 These results indicate that the PMO/AAO membrane has an evident nanofluidic characteristic.36 Furthermore, to explore the effect of PMO contents on the ion transport performance, hybrid membranes with different PMOs contents were fabricated (Experimental section). The I–V curves of the membranes were recorded in 0.1 M KCl solution. As shown in Fig. 3d and S7–S10,† with the increase of PMO contents (25–45 wt%), the thickness of the PMO layer increases gradually. In addition, the ion flux also improves accordingly until 40 wt% loading, which may be assigned to the increase of porosity and 3D connected pathways for fast ion transport (Fig. 3e).52 However, upon reaching 45 wt% PMO loading, the current decreases. This may be due to the partial aggregation of PMOs (Fig. 3f and S10†), which produces more complex channel structure and increases the inner resistance of the membrane.57 Consequently, based on the characterization and electrochemical tests, PMO/AAO with 40 wt% PMOs was chosen for further testing to observe better performance in GSH detection as it showed the highest ion flux.
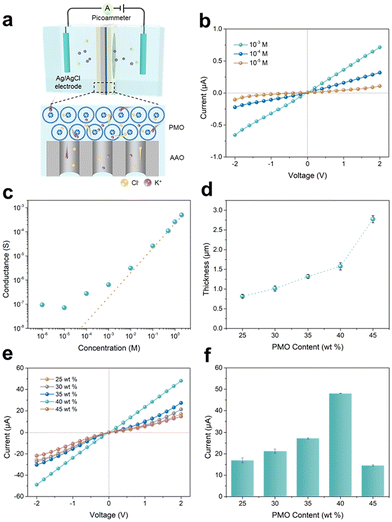 |
| Fig. 3 Ion transport properties of the PMO/AAO. (a) Schematic illustration of the ion transport process of the PMO/AAO. (b) I–V curves of the PMO/AAO under different concentrations of KCl solution. (c) Effect of KCl concentrations on conductance of the PMO/AAO membrane. (d) The thickness of the PMO layer with various PMO contents, n = 3 replicates. (e) The I–V curves and (f) the ionic current at +2 V of the hybrid membranes with various PMO contents. | |
Response of the PMO/AAO membrane to GSH
The disulfide bonds in the mesoporous framework can be reduced by GSH, enabling the generation of thiol groups.58 Firstly, the feasibility of the PMO/AAO in response to GSH was investigated via Ellman's test (Experimental section). According to the UV-vis spectrometry in Fig. 4a, the Ellman's reagent (DTNB) solution displayed no obvious absorption when treated with PMOs alone. However, notable absorbance was observed for the DTNB solution treated with PMOs that had been pre-incubated with GSH (PMOs + GSH). The strong absorption is ascribed to the by-product TNB2−, which was formed by the reaction between thiol groups and DTNB.58 This result verified that the GSH efficiently induces the generation of thiol groups at the mesoporous framework. In addition, the wettability of the GSH-incubated membrane (PMO/AAO + GSH) was tested, and the CA of the PMO/AAO changed from 41.9 ± 0.3° to 28.8 ± 0.5° after reacting with GSH (Fig. 4b). The significant increase in the hydrophilicity primarily results from the efficient conversion of disulfide bonds into more hydrophilic thiol components. Then, the ion transport of PMO/AAO in response to GSH was studied with 0.1 M KCl as an electrolyte. According to the I–V results in Fig. 4c and d, the current of PMO/AAO increased from 47.9 μA to 69.9 μA at +2 V after exposure to 0.1 mM GSH. In contrast, the I–V curves of the bare AAO membrane showed no obvious change after treating with GSH, confirming the presence of interaction between GSH and PMO/AAO (Fig. 4e). The change in the wettability of the membranes plays a critical role in the ion transport.59 Thus, the increasing current is attributed to the corresponding change of surface wettability, since the electrolyte can easily permeate into more hydrophilic channels to facilitate ion migration.60
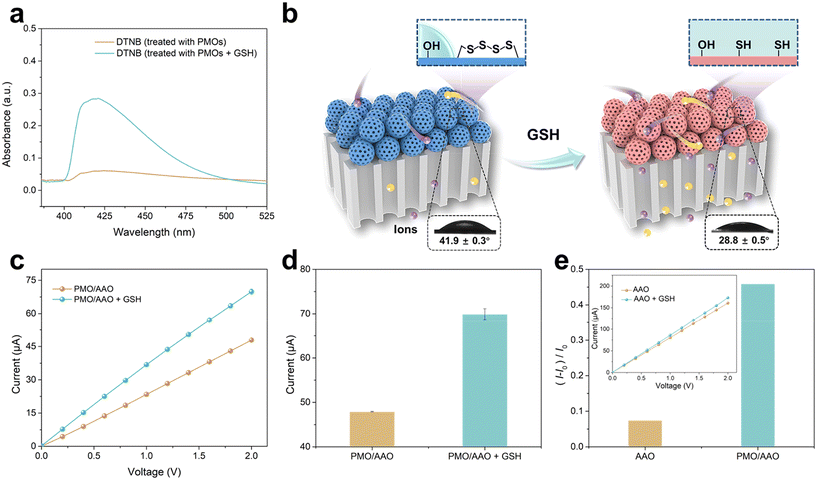 |
| Fig. 4 Response of PMO/AAO membrane to GSH. (a) UV-vis spectra of DTNB solution after incubation with PMOs or PMOs after reaction with 10 mM GSH. (b) Scheme of the response process and the CAs of PMO/AAO before and after reacting with 10 mM GSH, n = 3 replicates. (c) I–V curves and (d) the current of PMO/AAO before and after reacting with 0.1 mM GSH at +2 V. (e) The current change ratio of AAO and PMO/AAO before and after reacting with 0.1 mM GSH at +2 V. | |
Sensing performance of the PMO/AAO membrane
To evaluate the performance of PMO/AAO, the GSH response kinetics was studied by I–V measurements. The I–V curves of the PMO/AAO membrane after interacting with 0.1 mM and 10 mM GSH with response time from 0 to 60 min were recorded, respectively. As shown in Fig. 5a and b, the sensor can rapidly detect GSH at 10 min. Moreover, the current at +2 V gradually increases and reaches a stable state before 60 min (Fig. 5c). The overall trends of the signal suggest that GSH reacted with disulfide bonds in PMO/AAO gradually and eventually reached the equilibrium. Afterwards, the I–V curves were recorded with immersing the membrane in GSH solution with a series of concentrations. The results show that the current at +2 V increases gradually with the concentration increasing from 0.01 mM to 10 mM (Fig. 5d). The relationship between the ionic current change (I – I0) at +2 V and GSH concentration (C) shows two linear correlations over the range of 0.01–0.1 mM with an equation of (I – I0) = 185.15 × C + 0.59 (R2 = 0.993) and 0.1–10 mM with the equation (I – I0) = 2.16 × C + 21.49 (R2 = 0.958) (Fig. 5e). The detection limit is 1.2 μM. In order to demonstrate the specificity and selectivity of PMO/AAO, the membrane was treated with other amino acids at the same concentration of 0.1 mM, including L-Arg, L-Lys, L-Glu, Gly, and L-Cys. As shown in Fig. 5f, the current change of the membrane upon reaction with GSH is higher than those of most amino acids, indicating that the sensor has good selective reactivity towards GSH over these amino acids, except L-Cys. The –SH of L-Cys may be involved in the thiol–disulfide exchange reaction.61,62 Given that GSH is the most abundant biothiol in cytoplasm, with concentrations much higher than those of L-Cys and other biothiols, the potential interference from L-Cys and other biothiols can be considered negligible in diluted samples.62 In addition, we investigated the influence of temperature and pH on the detection. As shown in Fig. S11a,† the PMO/AAO can respond to GSH within the temperature range of 20–40 °C, indicating the potential applicability of PMO/AAO for GSH detection under physiological temperature conditions. Moreover, it can be observed that the change in current after the PMO/AAO reaction with GSH under acidic conditions is smaller than that under neutral or alkaline conditions (Fig. S11b†), which may be attributed to the nucleophilic ability of the –SH of GSH being decreased under acidic conditions, resulting in reduction in the reaction rate and effectiveness.63 To illustrate the practicability of this method, the membrane was used to detect GSH in FBS samples as proof-of-concept demonstrations (Experimental section). The recoveries are in the range of 90.6–118.4% (Table S1†), demonstrating that the membrane is a promising platform for practical GSH sensing.
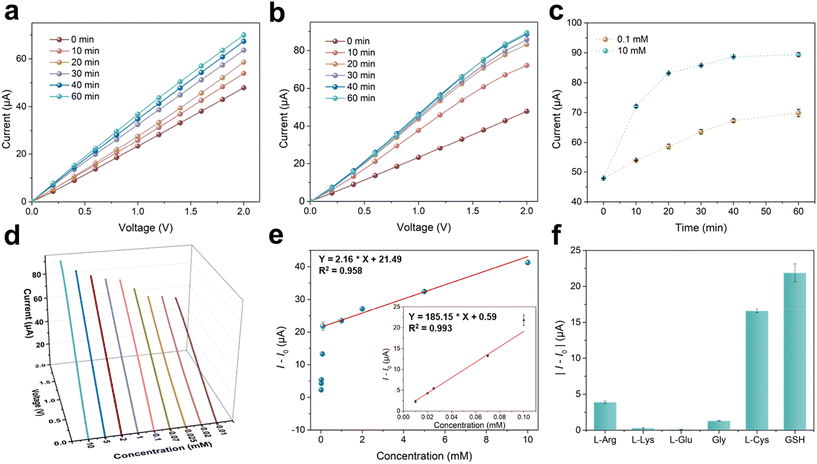 |
| Fig. 5 Sensing performance of PMO/AAO. I–V curves of the PMO/AAO membrane after interacting with (a) 0.1 mM GSH, and (b) 10 mM GSH with varied response time. (c) Time-dependent current variation trend at +2 V. (d) I–V curves of the PMO/AAO membrane after treatment with incremental GSH concentrations. (e) The calibration curves of the ionic current changes versus the GSH concentrations at +2 V in the range of 0.01–0.1 mM (the inset) and 0.1–10 mM. (f) The absolute current changes values of the PMO/AAO membrane treated with different analytes, n = 5 replicates. | |
Conclusions
In summary, we adopted a super-assembly strategy without further chemical modification to construct a PMO/AAO membrane for efficient GSH sensing. Thioether-bridged organosilica frameworks of PMOs endow the hybrid membrane with GSH-responsive switchable wettability. The sensing process was tracked by the ionic current across the membrane. Benefiting from the yolk–shell structure with enlarged surface area and plentiful mesochannels for fast ion transport, the fabricated membrane achieved sensitive and selective GSH detection with a detection limit of 1.2 μM. This sensor had potential application in practical sensing of GSH. This research paves the way for fabricating nanochannel-based sensors for biosensing application and facilitates the nanofluidic sensing technique.
Author contributions
H. Zeng: conceptualization, methodology, software, investigation, writing – original draft, investigation, formal analysis, and validation. S. Zhou, X. Zhang, Q. Liang, and M. Yan: methodology and formal analysis. Y. Xu, Y. Guo, and X. Hu: formal analysis. L. Jiang: writing – reviewing & editing. B. Kong: conceptualization, writing – reviewing & editing, supervision, resources, funding acquisition, and project administration.
Conflicts of interest
There are no conflicts to declare.
Acknowledgements
This work was supported by the National Key Research and Development Program of China (2019YFC1604601, 2019YFC1604600, 2017YFA0206901, 2017YFA0206900, and 2018YFC1602301), the National Natural Science Foundation of China (21705027, 21974029, and 22105042), and the Yiwu Research Institute Program of Fudan University (20-1-04).
References
- X. Jiang, J. Chen, A. Bajić, C. Zhang, X. Song, S. L. Carroll, Z.-L. Cai, M. Tang, M. Xue, N. Cheng, C. P. Schaaf, F. Li, K. R. MacKenzie, A. C. M. Ferreon, F. Xia, M. C. Wang, M. Maletić-Savatić and J. Wang, Nat. Commun., 2017, 8, 16087 CrossRef CAS PubMed.
- M. L. Reniere, A. T. Whiteley, K. L. Hamilton, S. M. John, P. Lauer, R. G. Brennan and D. A. Portnoy, Nature, 2015, 517, 170–173 CrossRef CAS PubMed.
- Z. G. Khan, M. R. Patil, S. N. Nangare, A. G. Patil, S. H. S. Boddu, R. S. Tade and P. O. Patil, J. Nanostruct. Chem., 2022, 12, 1053–1074 CrossRef.
- G. Bjørklund, M. Peana, M. Maes, M. Dadar and B. Severin, Neurosci. Biobehav. Rev., 2021, 120, 470–478 CrossRef PubMed.
- Q. Gao, W. Zhang, B. Song, R. Zhang, W. Guo and J. Yuan, Anal. Chem., 2017, 89, 4517–4524 CrossRef CAS PubMed.
- L. A. Herzenberg, S. C. De Rosa, J. G. Dubs, M. Roederer, M. T. Anderson, S. W. Ela, S. C. Deresinski and L. A. Herzenberg, Proc. Natl. Acad. Sci. U. S. A., 1997, 94, 1967–1972 CrossRef CAS PubMed.
- C. Kinoshita, K. Aoyama, N. Matsumura, K. Kikuchi-Utsumi, M. Watabe and T. Nakaki, Nat. Commun., 2014, 5, 3823 CrossRef CAS PubMed.
- Y. Xiong, C. Xiao, Z. Li and X. Yang, Chem. Soc. Rev., 2021, 50, 6013–6041 RSC.
- S. Bauhuber, C. Hozsa, M. Breunig and A. Göpferich, Adv. Mater., 2009, 21, 3286–3306 CrossRef CAS PubMed.
- H. Wang, L. Yang, S. Chu, B. Liu, Q. Zhang, L. Zou, S. Yu and C. Jiang, Anal. Chem., 2019, 91, 9292–9299 CrossRef CAS PubMed.
- H. Wang, L. Da, L. Yang, S. Chu, F. Yang, S. Yu and C. Jiang, J. Hazard. Mater., 2020, 392, 122506 CrossRef CAS PubMed.
- S. Han, L. Yang, Z. Wen, S. Chu, M. Wang, Z. Wang and C. Jiang, J. Hazard. Mater., 2020, 398, 122894 CrossRef CAS PubMed.
- J. Zhu, J. Shen, B. Hu, L. Yang and C. Jiang, Anal. Chem., 2022, 94, 1126–1134 CrossRef CAS PubMed.
- F. Yang, D. Lin, L. Pan, J. Zhu, J. Shen, L. Yang and C. Jiang, Anal. Chem., 2021, 93, 14506–14513 CrossRef CAS PubMed.
- B. Hu, X. Kang, S. Xu, J. Zhu, L. Yang and C. Jiang, Anal. Chem., 2023, 95, 3587–3595 CrossRef CAS PubMed.
- B. Hu, J. Zhu, J. Shen, L. Yang and C. Jiang, Anal. Chem., 2022, 94, 7559–7566 CrossRef CAS PubMed.
- R. P. Shukla, M. Aroosh, A. Matzafi and H. Ben-Yoav, Adv. Funct. Mater., 2021, 31, 2004146 CrossRef CAS.
- Q. Ma, M. Wang, H. Cai, F. Li, S. Fu, Y. Liu and Y. Zhao, J. Mater. Chem. B, 2021, 9, 3563–3572 RSC.
- M. C. C. Areias, K. Shimizu and R. G. Compton, Analyst, 2016, 141, 2904–2910 RSC.
- J. Li, C. Cao, H. Li, S. Chen, X. Gong and S. Wang, Sens. Actuators, B, 2024, 409, 135597 CrossRef CAS.
- D. Zhang, Y. Sun, Z. Wang, F. Liu and X. Zhang, Nat. Commun., 2023, 14, 1901 CrossRef PubMed.
- Y. Sun, S. Chen, X. Chen, Y. Xu, S. Zhang, Q. Ouyang, G. Yang and H. Li, Nat. Commun., 2019, 10, 1323 CrossRef PubMed.
- T. Ye, G. Hou, W. Li, C. Wang, K. Yi, N. Liu, J. Liu, S. Huang and J. Gao, Nat. Commun., 2021, 12, 5231 CrossRef CAS PubMed.
- K. Wu, X.-Y. Kong, K. Xiao, Y. Wei, C. Zhu, R. Zhou, M. Si, J. Wang, Y. Zhang and L. Wen, Adv. Funct. Mater., 2019, 29, 1807953 CrossRef.
- M. Cheng, F. Zhu, S. Zhang, X. Zhang, M. K. Dhinakaran and H. Li, Nano Lett., 2021, 21, 4086–4091 CrossRef CAS PubMed.
- Y. Sun, F. Zhang, J. Quan, F. Zhu, W. Hong, J. Ma, H. Pang, Y. Sun, D. Tian and H. Li, Nat. Commun., 2018, 9, 2617 CrossRef PubMed.
- Q. Zhang, J. Kang, Z. Xie, X. Diao, Z. Liu and J. Zhai, Adv. Mater., 2018, 30, 1703323 CrossRef PubMed.
- M. R. Powell, L. Cleary, M. Davenport, K. J. Shea and Z. S. Siwy, Nat. Nanotechnol., 2011, 6, 798–802 CrossRef CAS PubMed.
- G. Xie, P. Li, Z. Zhao, Z. Zhu, X.-Y. Kong, Z. Zhang, K. Xiao, L. Wen and L. Jiang, J. Am. Chem. Soc., 2018, 140, 4552–4559 CrossRef CAS PubMed.
- Z. Zhang, X.-Y. Kong, K. Xiao, G. Xie, Q. Liu, Y. Tian, H. Zhang, J. Ma, L. Wen and L. Jiang, Adv. Mater., 2016, 28, 144–150 CrossRef CAS PubMed.
- X. Li, T. Zhai, P. Gao, H. Cheng, R. Hou, X. Lou and F. Xia, Nat. Commun., 2018, 9, 40 CrossRef PubMed.
- M. Zhao, W. Wu and B. Su, ACS Appl. Mater. Interfaces, 2018, 10, 33986–33992 CrossRef CAS PubMed.
- Y. Huang, L. Liu, C. Luo, W. Liu, X. Lou, L. Jiang and F. Xia, Chem. Soc. Rev., 2023, 52, 6270–6293 RSC.
- D. Ding, P. Gao, Q. Ma, D. Wang and F. Xia, Small, 2019, 15, 1804878 CrossRef PubMed.
- D. Zhang and X. Zhang, Nano Lett., 2022, 22, 3793–3800 CrossRef CAS PubMed.
- H. Zeng, S. Zhou, L. Xie, X. Zhang, J. Zeng, M. Yan, Q. Liang, T. Liu, K. Liang, L. Zhang, P. Chen, L. Jiang and B. Kong, Anal. Chem., 2022, 94, 2589–2596 CrossRef CAS PubMed.
- H. Zeng, S. Zhou, L. Xie, Q. Liang, X. Zhang, M. Yan, Y. Huang, T. Liu, P. Chen, L. Zhang, K. Liang, L. Jiang and B. Kong, Biosens. Bioelectron., 2023, 222, 114985 CrossRef CAS PubMed.
- S. Zhou, L. Zhang, L. Xie, J. Zeng, B. Qiu, M. Yan, Q. Liang, T. Liu, K. Liang, P. Chen and B. Kong, Anal. Chem., 2021, 93, 2982–2987 CrossRef CAS PubMed.
- Y. Chen and J. Shi, Adv. Mater., 2016, 28, 3235–3272 CrossRef CAS PubMed.
- C. Yuan, X. Wu, R. Gao, X. Han, Y. Liu, Y. Long and Y. Cui, J. Am. Chem. Soc., 2019, 141, 20187–20197 CrossRef CAS PubMed.
- Z. Teng, X. Su, Y. Zheng, J. Zhang, Y. Liu, S. Wang, J. Wu, G. Chen, J. Wang, D. Zhao and G. Lu, J. Am. Chem. Soc., 2015, 137, 7935–7944 CrossRef CAS PubMed.
- Z. Teng, W. Li, Y. Tang, A. Elzatahry, G. Lu and D. Zhao, Adv. Mater., 2019, 31, e1707612 CrossRef PubMed.
- X. Liu, Y. Maegawa, Y. Goto, K. Hara and S. Inagaki, Angew. Chem., Int. Ed., 2016, 55, 7943–7947 CrossRef CAS PubMed.
- T. Himiyama, M. Waki, Y. Maegawa and S. Inagaki, Angew. Chem., Int. Ed., 2019, 58, 9150–9154 CrossRef CAS PubMed.
- M. Kalantari, Y. Liu, E. Strounina, Y. Yang, H. Song and C. Yu, J. Mater. Chem. A, 2018, 6, 17579–17586 RSC.
- M. Liu, L. Chen, Z. Zhao, M. Liu, T. Zhao, Y. Ma, Q. Zhou, Y. S. Ibrahim, A. A. Elzatahry, X. Li and D. Zhao, J. Am. Chem. Soc., 2022, 144, 3892–3901 CrossRef CAS PubMed.
- B. Karimi, N. Ganji, O. Pourshiani and W. R. Thiel, Prog. Mater. Sci., 2022, 125, 100896 CrossRef CAS.
- Q. Ma, T. Liu, R. Xu, Q. Du, P. Gao and F. Xia, Anal. Chem., 2021, 93, 1984–1990 CrossRef CAS PubMed.
- Z. Teng, X. Su, B. Lee, C. Huang, Y. Liu, S. Wang, J. Wu, P. Xu, J. Sun, D. Shen, W. Li and G. Lu, Chem. Mater., 2014, 26, 5980–5987 CrossRef CAS.
- J. H. Kim, B. Fang, M. Y. Song and J.-S. Yu, Chem. Mater., 2012, 24, 2256–2264 CrossRef CAS.
- Z. Teng, S. Wang, X. Su, G. Chen, Y. Liu, Z. Luo, W. Luo, Y. Tang, H. Ju, D. Zhao and G. Lu, Adv. Mater., 2014, 26, 3741–3747 CrossRef CAS PubMed.
- J. Wang, Y. Liu, Q. Cai, A. Dong, D. Yang and D. Zhao, Adv. Mater., 2022, 34, 2107957 CrossRef CAS PubMed.
- M.-H. Sun, S.-Z. Huang, L.-H. Chen, Y. Li, X.-Y. Yang, Z.-Y. Yuan and B.-L. Su, Chem. Soc. Rev., 2016, 45, 3479–3563 RSC.
- Y. Huang, H. Zeng, L. Xie, R. Gao, S. Zhou, Q. Liang, X. Zhang, K. Liang, L. Jiang and B. Kong, J. Am. Chem. Soc., 2022, 144, 13794–13805 CrossRef CAS PubMed.
- Z. Sun, T. Liao, Y. Zhang, J. Shu, H. Zhang and G.-J. Zhang, Biosens. Bioelectron., 2016, 86, 194–201 CrossRef CAS PubMed.
- J. Gao, W. Guo, D. Feng, H. Wang, D. Zhao and L. Jiang, J. Am. Chem. Soc., 2014, 136, 12265–12272 CrossRef CAS PubMed.
- M. Pan, J. Cai, S. Li, L. Xu, W. Ma, C. Xu and H. Kuang, Anal. Chem., 2021, 93, 4825–4831 CrossRef CAS PubMed.
- B. Niu, Y. Zhou, K. Liao, T. Wen, S. Lao, G. Quan, X. Pan and C. Wu, Acta Pharm. Sin. B, 2022, 12, 2074–2088 CrossRef CAS PubMed.
- Q. Ma, R. Wang, P. Gao, Y. Dai and F. Xia, Anal. Chem., 2022, 94, 16411–16417 CrossRef CAS PubMed.
- H. Chen, L. Xu, W. Tuo, X. Chen, J. Huang, X. Zhang and Y. Sun, Anal. Chem., 2020, 92, 4131–4136 CrossRef CAS PubMed.
- Y. Zhang, S. Xia, S. Wan, T. E. Steenwinkel, T. Vohs, R. L. Luck, T. Werner and H. Liu, ChemBioChem, 2021, 22, 2282–2291 CrossRef CAS PubMed.
- C. Wei, X. Liu, Y. Gao, Y. Wu, X. Guo, Y. Ying, Y. Wen and H. Yang, Anal. Chem., 2018, 90, 11333–11339 CrossRef CAS PubMed.
- S. Mo, X. Zhang, S. Hameed, Y. Zhou and Z. Dai, Theranostics, 2020, 10, 2130–2140 CrossRef CAS PubMed.
|
This journal is © The Royal Society of Chemistry 2024 |