DOI:
10.1039/D4AN00170B
(Minireview)
Analyst, 2024,
149, 2498-2506
Impact electrochemistry for biosensing: advances and future directions
Received
31st January 2024
, Accepted 6th April 2024
First published on 15th April 2024
Abstract
Impact electrochemistry allows for the investigation of the properties of single entities, ranging from nanoparticles (NPs) to soft bio-particles. It has introduced a novel dimension in the field of biological analysis, enhancing researchers’ ability to comprehend biological heterogeneity and offering a new avenue for developing novel diagnostic devices for quantifying biological analytes. This review aims to summarize the recent advancements in impact electrochemistry-based biosensing over the past two to three years and provide insights into the future directions of this field.
Introduction
The specific detection of a single entity (e.g., a cell, a nanoparticle [NP], or single molecule) represents the highest level of sensitivity that can be achieved in electroanalysis.1 Aiming for this level of sensitivity is essential for advancing our understanding of nature because the study of single entities allows for the observation of phenomena that may be unnoticeable in ensemble measurements involving numerous entities.2 Particularly, impact electrochemistry is of great significance for the determination of a single entity owing to its unique advantages in single-entity electrochemical analysis.3–7 Impact electrochemistry is based on the measurement of an electrochemical signal that occurs when a single entity freely moving in a solution collides with a potentiostatted electrode surface, mainly due to faradaic charge transfer. The collision events are primarily quantified through chronoamperometry (i–t),8,9 as well as other electrochemical methods such as fast-scan cyclic voltammetry (CV)10 and open circuit potential (OCP) measurements.11 These measurements typically involve three different readout principles occurring in the individual collision events, namely, the obstruction of a reaction at an electrode,12,13 the direct electrolysis of colliding particles,14–16 and the electrochemical reactions catalyzed at the surfaces of the colliding particles.17–19 In addition to these, the disturbance of the double layer when particles collide with the electrode interface may induce non-faradaic responses.20–22 The detected signals manifest as sudden, discrete drops or rises of the otherwise smooth i–t background during the collision events. These are categorized as transient “spikes” or “staircases”, depending on the process occurring between the colliding particles and the electrode surface.23,24
Impact electrochemistry originally served as a powerful electroanalytical tool for the analysis and characterization of rigid inorganic nanoparticles, encompassing the acquisition of the physicochemical properties,7,25–37 reaction kinetics,38–42 and reaction mechanisms.43 Recently, impact electrochemistry has also been increasingly used for nanoparticle synthesis and screening purposes,44–47 as well as for studying entity-to-entity interactions48 and the transient electrochemical process at a confined interface.22,49,50 In addition to rigid nanoparticles, impact electrochemistry has also found applications in the detection of soft nanoparticles such as organic nanoparticles51 emulsion nanodroplet52–55 and liposomes.56 Furthermore, given its potential to reduce the limit of detection (LOD) to the single entity level and enable in situ detection of analytes one at a time with fast response and high throughput, impact electrochemistry has been applied to detect a wide range of biospecies, including nucleic acids,57–59 proteins,60 bacteria,61 viruses,62 vesicles,63 and cells.64
Impact electrochemistry-based biosensing mainly relies on three principles, including the use of labeled electroactive probes, direct electrolysis of individual bioentities, and diffusion blocking. So far, several review papers have addressed impact electrochemistry-based bioanalysis, including the detection of single soft bioentities,65 single enzymes1 and single bacteria.66 However, a comprehensive review covering all biospecies in the recent two to three years has not been available. In the present article, we focus on the recent advances in impact electrochemistry-based bioanalysis categorized by detection methods. We also highlight the strategies employed to enhance the performance of the analysis. Finally, we summarize the challenges in this filed and offer a perspective on its future direction.
Recent developments in impact electrochemistry-based biosensing
As most bioanalytes are electrochemically inactive, the use of electrochemical probes is typically required to transduce signals during collision events. For single bioentities possessing redox centers, detection can be achieved through direct electrolysis upon their collisions with the electrode. Additionally, diffusion blocking is a universal approach applicable to almost all inactive bioanalytes. The following section will discuss the analysis of various types of bioanalytes based on the aforementioned detection methods using impact electrochemistry.
Indirect analysis using redox probes
Indirect analysis of bioanalytes making use of redox probes for the electrochemical readouts is primarily applicable to proteins and nucleic acids, with silver nanoparticles (AgNPs) and platinum nanoparticles (PtNPs) being among the most commonly used probes. PtNPs exhibit high activity in catalyzing electrochemical reactions,67,68 while AgNPs can be readily oxidized in appropriate electrolytes,69 enabling rapid signal extraction during collision events. The obtained transient currents in the i–t curves contain abundant information, such as impact frequency, current amplitude, charge, and current lifetime. All of these parameters can be utilized in combination of various strategies for analyte quantification.
The use of PtNPs as electroactive probes originated from the electrocatalytic amplification (ECA) of hydrazine oxidation catalyzed by the surfaces of individual PtNPs upon colliding with the electrodes, as introduced by the Bard group.70 This concept was subsequently applied to DNA/RNA detection by observing changes in impact frequency before and after the hybridization of targets to ssDNA-modified PtNPs.71–73 This methodology opens new avenues of designing sensitive biosensing methods utilizing impact electrochemistry. However, the sensitivity is compromised in practical applications due to low impact frequency and susceptibility to interference from complex sample matrices.
To improve the sensitivity levels in real sample detection, recently, various strategies have been incorporated into the design of ECA-based biosensors. Wang et al. achieved the quantification of human immunodeficiency virus DNA (HIV-DNA) within complex systems by integrating DNA walker amplification into impact electrochemistry, resulting in a remarkable threefold increase in impact frequency.59 The limit of detection (LOD) can reach as low as 4.86 fM, representing a reduction by 1 to 4 orders of magnitude compared to traditional modified electrodes.59 Later on, the same authors combined impact electrochemistry with rolling circle amplification (RCA) and demonstrated the detection of cardiac troponin I (cTnI) (Fig. 1a).74 The presence of cTnI prompted the specific formation of magnetic immunocomplexes, initiating RCA and enzyme digestion reactions that subsequently led to the release of PtNPs for collision experiments. As a result, the LOD can then be lowered to 0.57 fg mL−1. The successful application of this method in clinical samples was also demonstrated. To further enhance the sensitivity to its limit, Zhang et al. introduced a one-to-many strategy for the detection of microRNA-21, a breast cancer marker.75 Their strategies involved the integration of satellite magnetic NP (MN)–DNA–PtNP conjugates with duplex-specific nuclease-assisted PtNP releasing. The resulting small-sized and nearly naked PtNPs significantly promote the collision frequency and remarkably lowed the LOD to 47 aM.58 This method even exhibited the ability to differentiate non-target microRNAs containing single-nucleotide mismatches.
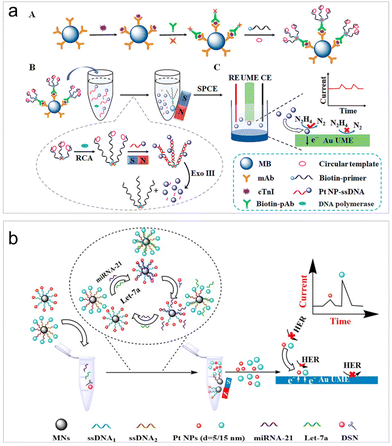 |
| Fig. 1 (a) Magnetic RCA-assisted immunosensor for cTnI detection based on impact electrochemistry. Reproduced with permission from ref. 74. Copyright American Chemical Society, 2022. (b) Schematic of the size-resolved single entity collision biosensing for the quantification of microRNA in a single run using 5 nm and 15 nm PtNPs as electrochemical probes. Reproduced with permission from ref. 76. Copyright American Chemical Society, 2021. | |
In addition to the impact frequency, the current lifetime in the collision events can also serve as signal output mode for target analysis.34 Leveraging differences in the current rise time resulting from the collisions of PtNPs of various sizes on an ultramicroelectrode (UME), Zhang et al. developed a size-resolved strategy for multiple microRNA detection assays using two sizes of PtNPs (5 and 15 nm) as detection probes (Fig. 1b).76 It is important to highlight that the new signal output strategy for the first time facilitated the simultaneous quantitative detection of two microRNAs.
The above work utilizes impact electrochemical methods with single PtNPs as electroactive probes. The transient current responses obtained are usually step-like, primarily due to collision adsorption of PtNPs. This would result in an increase in background current, making it difficult to distinguish the step-like transient signals from the background level, thereby lowering the detection sensitivity.
AgNPs are a type of redox labels employed to report signals through direct oxidation. Unlike in most impact electrochemistry-based bioanalysis using ECA strategies, where only surface atoms are available for the electrochemical readout, the direct oxidation of AgNPs utilizes all the atoms of an AgNP to transduce the signal. Compared with PtNPs of the same size, the detection sensitivity may be significantly enhanced when using current (and in most cases, charge) output mode of AgNPs. The direct electrolysis of AgNPs has been commonly used for the detection of various analytes, including viruses,77 bacteria,78 and tumor protein markers.79 The first study related to this detection principle was conducted by Compton et al. in 2015, where E. coli was labeled with a certain amount of AgNPs through electrostatic interaction.78 When individual E. coli arrived in the electron tunneling region of a UME surface, the adsorbed AgNPs were oxidized into Ag+, generating current spikes in the i–t curve. Later on, the same group extended this detection approach to the quantification of single influenza viruses at low concentrations.80 It is noteworthy that this type of detection lacks selectivity, as it does not involve a bio-recognition procedure.
Recently, Zhou et al. introduced a bio-recognition procedure into the impact electrochemistry-based detection and analyzed the changes in oxidative charge and impact frequency of AgNPs for the detection of cytokeratin 19 fragment (CYFRA21-1),79 achieving a LOD of 0.1 ng mL−1. Following this work, they devised an aggregation–collision strategy for quantifying alpha-fetoprotein (AFP) (Fig. 2a). This strategy relied on the aggregation of AgNPs triggered by molecular biorecognition between AFP and AgNP-anti-AFP probes. This led to an increase in AgNP size and a decrease in AgNP concentration, enabling an accurate, self-validated dual-mode immunoassay through impact analysis of AgNP oxidation.81 This biosensor enabled a LOD of 5 pg mL−1 for AFP analysis with remarkable specificity.
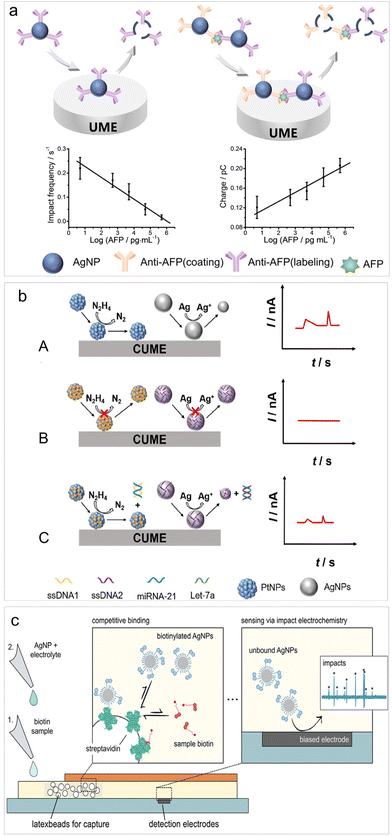 |
| Fig. 2 (a) Schematic of the AFP biosensing based on impact electrochemistry. Reproduced with permission from ref. 81. Copyright American Chemical Society, 2023. (b) Schematic diagram of signal-resolved biosensing for multiplex immunoassay for target microRNAs in a single run. Reproduced with permission from ref. 83. Copyright American Chemical Society, 2023. (c) Digital lateral flow sensor based on impact electrochemistry and competitive binding. Reproduced with permission from ref. 84. Copyright American Chemical Society, 2022. | |
By combining magnetic separation, liposome encapsulation release, and DNAzyme-assisted signal amplification, Wang et al. achieved the detection of the severe acute respiratory syndrome coronavirus 2 (SARS-CoV-2) through AgNP electrolysis on polysulfide (Ps)-functionalized Au ultramicroelectrodes (UMEs).82 The resulting biosensor detected the SARS-CoV-2 spike protein with a LOD of 6.78 fg mL−1 and has successfully been applied to real sample detection.
The utilization of both the catalytic properties of PtNPs and the electrolysis of AgNPs has been reported recently. By distinguishing between two types of current signals from impact electrochemistry, namely step-type current transients produced by PtNPs catalyzed hydrazine oxidation and peak-type current transients produced by AgNPs oxidation, a multiplex immunoassay for target microRNAs (microRNA-21 and Let-7a) have been established during single-particle impact electrochemistry in a single run (Fig. 2b).83 This multiplex immunoassay can also be applied for the detection of other DNAs/RNAs, proteins and various other biomolecules.
To enable point-of-care (POC) settings targeting small analytes, the lateral flow assay has been integrated into impact electrochemistry-based sensing. Wolfrum et al. introduced a digital lateral flow sensor based on direct AgNP electrolysis for detecting free biotin in a solution (Fig. 2c).84 This detection took place within a membrane-based microfluidic platform, where free biotin and biotinylated AgNPs competed for binding to streptavidin immobilized on embedded latex beads. Consequently, the concentration of free biotin could be determined through the electro-oxidative collision of excessive AgNPs at an array of detection electrodes. This research holds significant potential for future applications in POC testing of low concentrations of small analytes, such as neurotransmitters or organophosphates even at low concentrations.
It is important to note that multi-collision events85,86 may take place, adding difficulties in accurate data statistics. Therefore, before performing impact experiments, a set of experimental parameters should be designed according to Poisson approximation,87 including the concentration of the NP probes, the size of the UME, etc.
Direct analysis through single-entity electrolysis or redox mediating
For single bioentities intrinsically possessing redox centers, direct electrolysis upon collisions can enable the selective detection of single bio-entities without the need for electroactive probes. Such type of detection is mainly applicable to cells, including vesicles,88 red blood cells (RBCs),64 cancer cells,89 bacteria,90 and platelets.91 It is worth noting that the use of redox mediators is often involved in the detection of single bacteria based on their specific electrochemical activity with the mediator, which helps enhance detection sensitivity and selectivity.61,90
The direct electrolysis of single cells originated from the work of Ewing et al. to examine the electrochemical response of catecholamines hormones inside the vesicles,88 followed by the detection of a series of colliding vesicles or liposomes through their redox contents.56,92,93 In 2016, Compton et al. and Dick et al. reported side by side the impact analysis of RBCs and cancer cells. Compton's work focused on the catalytic activity of RBCs towards hydrogen peroxide,64 while Dick's study centered on the specific electroactive content belonging to cancer cells.89 Recently, similar detections have been applied to the investigation of the serotonin concentration within a single human platelet, which are well-known for storing serotonin.91 As depicted in Fig. 3a, when a single platelet collides with a UME, the serotonin inside the platelet is oxidized at the electrode surface, resulting in an anodic current peak during the collision. The concentration of serotonin can be quantified by integrating the peak current, and the concentration of platelets can be obtained according to the collision frequency. It is noteworthy that there are some limitations of impact electrochemistry for cell detection. Cells sometimes remain intact during impact, but other times experience adhesion, preventing the encapsulated redox molecules from being quantified. Therefore, it is essential to emphasize that the studies based on direct electrolysis principle usually rely on cell membrane rupture, which must occur during collision events to enable the release and detection of the electroactive content.
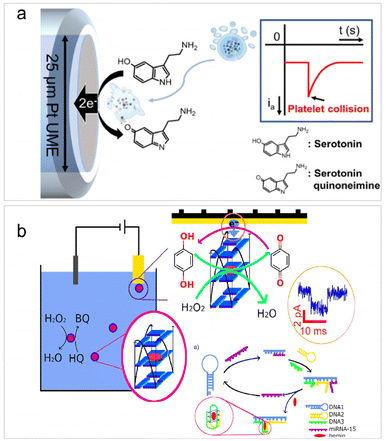 |
| Fig. 3 (a) Schematic of single platelet detection based on the oxidation of the encapsulated serotonin. Reproduced with permission from ref. 91. Copyright American Chemical Society, 2020. (b) Illustration of the detection of the catalytic current of a single GQH during a collision with the Au UME and HCR-based detection of microRNA-15. Reproduced with permission from ref. 94. Copyright American Chemical Society, 2021. | |
The analysis of single microorganisms, primarily bacteria, using impact electrochemistry is often in combination with redox mediating systems.61,90,95 The principle is based on the electron transfer of individual bacteria via the redox mediator, which is then detected at the potentiostatted microelectrode. For example, Zhi et al. successfully quantified the redox activity of two bacterial species: the Gram-negative Escherichia coli and the Gram-positive Bacillus subtilis.90 Following this work, they further reported a three-mediator combined method for selective identification of individual Saccharomyces cerevisiae within a mixed microbial consortium.61 Redox mediating was also employed in the detection of individual G-quadruplex/hemin (GQH) structures through their intrinsic peroxidase activities for catalyzing the reaction between hydrogen peroxide and hydroquinone.94 Since GQH can function as a DNAzyme for nucleic acid sensing, the authors further devised a hybridization chain reaction (HCR) strategy to detect microRNA-15 (Fig. 3b). In the presence of target microRNA-15, three DNA strands were hybridized to form a G-quadruplex subunit, triggering a collision-induced electrochemical signal from GQH within the i–t curve. This biosensor enabled the sensitive detection of microRNA-15 with a LOD of 50 fM.94
Direct analysis through diffusion blocking
The diffusion-blocking strategy involves impeding an ongoing electrode reaction on the electrode surface when inert entities collide with it. It is a widely employed label-free analysis approach for the direct detection of non-electroactive entities in impact electrochemistry. This detection principle was initially proposed by Lemay et al. who conducted both electrochemical and optical monitoring of insulating microbeads composed of different materials.13 Currently, the diffusion-blocking strategy is extensively utilized in the investigation of non-electroactive bio-entities, including cells,96 viruses,97 bacteria,98 platelets,99 and macromolecules such as proteins and nucleic acids.100
The detection principle of diffusion blocking suggests that a notable limitation of this method is its lack of selectivity. However, this method still proves useful for the biological reaction analysis, such as the detection and analysis of aggregation levels for the treatment and prevention of infectious diseases. Hemagglutination assays are essential for blood group typing, alloantibody screening, and biomarker detection. Li et al. examined the specific agglutination of red blood cells (RBCs) with and without the presence of antibodies by observing the RBC collision events on a Pt UME (Fig. 4a).96 In the presence of antibodies, various degrees of RBC agglutination occurred, resulting in larger current step magnitudes owing to the increased coverage of RBC clusters on the UME surface. Analysis of the collision frequency and the average current step magnitude allows for determining the concentration and extent of RBCs agglutination. Very recently, the nanopillar microarray electrode array electrode was fabricated for impact analysis of individual RBCs. The array electrode can significantly improve the diffusion movement of analytes in the electrolyte and the adsorption effect of analytes on the electrode surface, which achieved RBC detection in real blood samples at sub-picomolar concentrations, and the detection range covered physiological concentrations.101
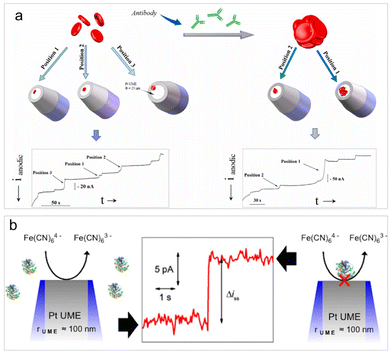 |
| Fig. 4 (a) Schematic of the detection and characterization of RBC agglutination. Reproduced with permission from ref. 96. Copyright Elsevier Science, 2021. (b) Schematic of the detection of single insulating macromolecules. Reproduced with permission from ref. 100. Copyright American Chemical Society, 2015. | |
It is worth highlighting the work of Bard et al. published in 2015.100 While it may not be the most recent study, it represents a significant contribution to the detection of single macromolecules, such as single proteins and DNA, through their collision events. This is likely the simplest technology to date capable of single-molecule resolution. The key parameter of the detection is the use of a UME with a radius smaller than 100 nm, allowing for the discrimination of signals from single molecules against a low background current. With this setup, discrete adsorption events of antibodies, enzymes, and DNA can be rendered detectable (Fig. 4b). Though this methodology is not selective, it may allow for the assessment of the quality and concentration of a molecular sample using a molecule's footprint (i.e., current step magnitude and collision frequency). This work conveys a message that extreme electrode manufacturing is crucial for pushing the limits of detection in impact electrochemistry.
Conclusion and future prospects
This review article presents a comprehensive summary of the advancements in biosensing based on impact electrochemistry, primarily over the past three years. Significant progress has been achieved in this field, particularly in enhancing detection sensitivity. For instance, the LOD for DNA detection has improved from nanomolar (nM) levels to femtomolar (fM) or even attomolar (aM) levels, while the LOD for protein detection has reduced from picomolar (pM) to femtomolar (fM) levels. Additionally, multiplex assay has been achieved through the utilization of multi-labeling technique. Furthermore, flow assays have been developed in conjunction with impact electrochemistry and are anticipated to be used for POC testing.
In the future, combining impact electrochemistry with microfluidics holds significant promise as a strategy for analyzing small volumes of analytes with low concentrations. This capability helps to conserve limited sample resources, minimize waste, and achieve higher sensitivity in detection, particularly in the fields such as clinical diagnostics. Single-molecule analysis represents highest level of sensitivity in detection; besides, it allows the understanding biological heterogeneity and revolutionize precision medicine. However, single-molecule analysis remains a challenging task, demanding ongoing efforts. While it has been achieved through diffusion blocking principle, such detection lacks selectivity and places high demands on electrode fabrication. Therefore, researchers should collaborate closely with material scientists to design conjugates that enable the detection of one molecule per probe (e.g., NPs). This approach, when combined with established bio-recognition or chemistry, can also be applied to the analysis of single small molecules. With the assistance of artificial intelligence for accurate data processing prediction, these advances may find potential applications in the fields of environmental monitoring, medical diagnostics, and pharmaceutical analysis.
Author contributions
Jian-Hua Zhang and Yi-Ge Zhou conceived the organization of this review. Jian-Hua Zhang and Dian-Mei Song drafted the manuscript, and Yi-Ge Zhou revised it.
Conflicts of interest
The authors declare that they have no known competing financial interests or personal relationships that could have appeared to influence the work reported in this paper.
Acknowledgements
This paper was supported by a grant from the Natural Science Foundation of Shandong Province (ZR2023QB129), the Startup Foundation for Advanced Talents (Doctoral) (Z6122054), the National Natural Science Foundation (22222404, 21972039), and the Science and Technology Project of Hunan Province (2020RC3021, 2021NK1020, 2021SK1020).
References
- K. J. Vannoy, A. Ryabykh, A. I. Chapoval and J. E. Dick, Single enzyme electroanalysis, Analyst, 2021, 146, 3413–3421 RSC
.
- B. Roehrich and L. Sepunaru, Nanoimpacts at active and partially active electrodes: Insights and limitations, Angew. Chem., Int. Ed., 2020, 59, 19184–19192 CrossRef CAS PubMed
.
- J. J. Gooding, Single entity electrochemistry progresses to cell counting, Angew. Chem., Int. Ed., 2016, 55, 11198–11202 CrossRef PubMed
.
- J.-H. Zhang and Y.-G. Zhou, Nano-impact electrochemistry: Analysis of single bioentities, TrAC, Trends Anal. Chem., 2020, 123, 115768 CrossRef CAS
.
- D. Song and Y.-G. Zhou, From ensemble electrochemistry to nanoparticle-impact electrochemistry: Altered reaction process and possible applications, Curr. Opin. Electrochem., 2022, 34, 101011 CrossRef CAS
.
- R. Zhong, X. Wang, Q. Tao, J. Zhang, C. Lin, H. Wei and Y. G. Zhou, From ensemble electrochemistry to nano-impact electrochemistry: Altered reaction selectivity, Angew. Chem., Int. Ed., 2022, 61, e202207270 CrossRef CAS PubMed
.
- W. Zhang, J. Li, X. H. Xia and Y. G. Zhou, Enhanced electrochemistry of single plasmonic nanoparticles, Angew. Chem., Int. Ed., 2022, 61, e202115819 CrossRef CAS PubMed
.
- H. J. Zhou, F. R. F. Fan and A. J. Bard, Observation of discrete Au nanoparticle collisions by electrocatalytic amplification using Pt ultramicroelectrode surface modification, J. Phys. Chem. Lett., 2010, 1, 2671–2674 CrossRef CAS
.
- X. H. Zhao and Y. G. Zhou, Rapid and accurate data processing for silver nanoparticle oxidation in nano-impact electrochemistry, Front. Chem., 2021, 9, 718000 CrossRef CAS PubMed
.
- S. J. Percival and B. Zhang, Fast-scan cyclic voltammetry allows determination of electron transfer kinetic constants in single nanoparticle collision, J. Phys. Chem. C, 2016, 120, 20536–20546 CrossRef CAS
.
- H. Zhou, J. H. Park, F. R. Fan and A. J. Bard, Observation of single metal nanoparticle collisions by open circuit (mixed) potential changes at an ultramicroelectrode, J. Am. Chem. Soc., 2012, 134, 13212–13215 CrossRef CAS PubMed
.
- Z. Deng, F. Maroun, J. E. Dick and C. Renault, Detection of individual conducting graphene nanoplatelet by electro-catalytic depression, Electrochim. Acta, 2020, 355, 136805 CrossRef CAS
.
- B. M. Quinn, P. van't Hof and S. G. Lemay, Time-Resolved Electrochemical Detection of Discrete Adsorption Events, J. Am. Chem. Soc., 2004, 126, 8360–8361 CrossRef CAS PubMed
.
- L. Dery, S. Dery, E. Gross and D. Mandler, Influence of Charged Self-Assembled Monolayers on Single Nanoparticle Collision, Anal. Chem., 2023, 95, 2789–2795 CrossRef CAS PubMed
.
- D. Hernandez, J. C. Vidal, F. Laborda, J. Perez-Arantegui, A. C. Gimenez-Ingalaturre and J. R. Castillo, Detection, size characterization and quantification of silver nanoparticles in consumer products by particle collision coulometry, Mikrochim. Acta, 2021, 188, 12 CrossRef CAS PubMed
.
- K. Tschulik, B. Haddou, D. Omanović, N. V. Rees and R. G. Compton, Coulometric sizing of nanoparticles: Cathodic and anodic impact experiments open two independent routes to electrochemical sizing of Fe3O4 nanoparticles, Nano Res., 2013, 6, 836–841 CrossRef CAS
.
- A. J. Bard, H. Zhou and S. J. Kwon, Electrochemistry of Single Nanoparticles via Electrocatalytic Amplification, Isr. J. Chem., 2010, 50, 267–276 CrossRef CAS
.
- F. Zhang, P. A. Defnet, Y. Fan, R. Hao and B. Zhang, Transient Electrocatalytic Water Oxidation in Single-Nanoparticle Collision, J. Phys. Chem. C, 2018, 122, 6447–6455 CrossRef CAS
.
- J. Lin, W. Zhao, S. Li, J. Wang, D. Zhang, Y. Zang and Q. Xin, Electrocatalytic performance of single nanoparticles for methanol oxidation reaction, J. Electroanal. Chem., 2023, 928, 117045 CrossRef CAS
.
- M. Azimzadeh Sani, N. G. Pavlopoulos, S. Pezzotti, A. Serva, P. Cignoni, J. Linnemann, M. Salanne, M. P. Gaigeot and K. Tschulik, Unexpectedly high capacitance of the metal nanoparticle/water interface: Molecular-level insights into the electrical double layer, Angew. Chem., 2022, 134, e202112679 CrossRef
.
- X. Liu, X. Chen, T. Chen, Y. Xu and X. Zeng, Time-resolved selective electrochemical sensing of carbon particles, Anal. Chem., 2020, 93, 761–768 CrossRef PubMed
.
- S. M. Lu, K. J. Vannoy, J. E. Dick and Y. T. Long, Multiphase chemistry under nanoconfinement: An electrochemical perspective, J. Am. Chem. Soc., 2023, 145, 25043–25055 CrossRef CAS PubMed
.
- H. Rudakemwa, K. J. Kim, T. E. Park, H. Son, J. Na and S. J. Kwon, Observation and analysis of staircase response of single palladium nanoparticle collision on gold ultramicroelectrodes, Nanomaterials, 2022, 12, 3095 CrossRef CAS PubMed
.
- Z. P. Xiang, H. Q. Deng, P. Peljo, Z. Y. Fu, S. L. Wang, D. Mandler, G. Q. Sun and Z. X. Liang, Electrochemical dynamics of a single platinum nanoparticle collision event for the hydrogen evolution reaction, Angew. Chem., Int. Ed., 2018, 57, 3522–3526 CrossRef
.
- Y. G. Zhou, N. V. Rees and R. G. Compton, The electrochemical detection and characterization of silver nanoparticles in aqueous solution, Angew. Chem., Int. Ed., 2011, 50, 4219–4221 CrossRef CAS PubMed
.
- B. J. Plowman, N. P. Young, C. Batchelor-McAuley and R. G. Compton, Nanorod aspect ratios determined by the nano-impact technique, Angew. Chem., Int. Ed., 2016, 55, 7002–7005 CrossRef CAS PubMed
.
- H. Ma, J. F. Chen, H. F. Wang, P. J. Hu, W. Ma and Y. T. Long, Exploring dynamic interactions of single nanoparticles at interfaces for surface-confined electrochemical behavior and size measurement, Nat. Commun., 2020, 11, 2307 CrossRef CAS PubMed
.
- S. Rano, C. Laberty-Robert, K. Ngo, C. M. Sanchez-Sanchez and V. Vivier, Characterization of LiCoO2 nanoparticle suspensions by single collision events, Phys. Chem. Chem. Phys., 2019, 21, 5416–5423 RSC
.
- X. Jiao, E. E. L. Tanner, S. V. Sokolov, R. G. Palgrave, N. P. Young and R. G. Compton, Understanding nanoparticle porosity via nanoimpacts and XPS: electro-oxidation of platinum nanoparticle aggregates, Phys. Chem. Chem. Phys., 2017, 19, 13547–13552 RSC
.
- F. Hosseini Narouei, D. Andreescu and S. Andreescu, Rapid characterization of arsenic adsorption on single magnetite nanoparticles by collisions at microelectrodes, Environ. Sci.: Nano, 2020, 7, 1999–2009 RSC
.
- L. J. K. Weiß, E. Music, P. Rinklin, L. Straumann, L. Grob, D. Mayer and B. Wolfrum, Engineering electrostatic repulsion of metal nanoparticles for reduced adsorption in single-impact electrochemical recordings, ACS Appl. Nano Mater., 2021, 4, 8314–8320 CrossRef
.
- L. Chen, E. E. L. Tanner, C. Lin and R. G. Compton, Impact electrochemistry reveals that graphene nanoplatelets catalyse the oxidation of dopamine via adsorption, Chem. Sci., 2018, 9, 152–159 RSC
.
- E. N. Saw, V. Grasmik, C. Rurainsky, M. Epple and K. Tschulik, Electrochemistry at single bimetallic nanoparticles – using nano impacts for sizing and compositional analysis of individual AgAu alloy nanoparticles, Faraday Discuss., 2016, 193, 327–338 RSC
.
- Y. Y. Bai, Y. J. Yang, Y. Xu, X. Y. Yang and Z. L. Zhang, Current lifetime of single-nanoparticle electrochemical collision for in situ monitoring nanoparticles agglomeration and aggregation, Anal. Chem., 2023, 95, 4429–4434 CrossRef CAS PubMed
.
- Y. Zhang, W. Ji, S. Zhang, N. Gao, T. Xu, X. Wang and M. Zhang, Vitamin D inhibits the early aggregation of α-synuclein and modulates exocytosis revealed by electrochemical measurements, Angew. Chem., Int. Ed., 2022, 61, e202111853 CrossRef CAS PubMed
.
- L. Ezra, Z. J. O'Dell, J. Hui and K. R. Riley, Emerging investigator series: quantifying silver nanoparticle aggregation kinetics in real-time using particle impact voltammetry coupled with UV-vis spectroscopy, Environ. Sci.: Nano, 2020, 7, 2509–2521 RSC
.
- E. E. Tanner, S. V. Sokolov, N. P. Young and R. G. Compton, DNA capping agent control of electron transfer from silver nanoparticles, Phys. Chem. Chem. Phys., 2017, 19, 9733–9738 RSC
.
- M. Chen, S. M. Lu, Y. Y. Peng, Z. Ding and Y. T. Long, Tracking the electrocatalytic activity of a single palladium nanoparticle for the hydrogen evolution reaction, Chem. – Eur. J., 2021, 27, 11799–11803 CrossRef CAS PubMed
.
- L. Chen, C. Lin and R. G. Compton, Single entity electrocatalysis: oxygen reduction mediated via methyl viologen doped Nafion nanoparticles, Phys. Chem. Chem. Phys., 2018, 20, 15795–15806 RSC
.
- J. M. Kahk, N. V. Rees, J. Pillay, R. Tshikhudo, S. Vilakazi and R. G. Compton, Electron transfer kinetics at single nanoparticles, Nano Today, 2012, 7, 174–179 CrossRef CAS
.
- Y. G. Zhou, B. Haddou, N. V. Rees and R. G. Compton, The charge transfer kinetics of the oxidation of silver and nickel nanoparticles via particle–electrode impact electrochemistry, Phys. Chem. Chem. Phys., 2012, 14, 14354–14357 RSC
.
- Y. G. Zhou, N. V. Rees and R. G. Compton, Electrochemistry of nickel nanoparticles is controlled by surface oxide layers, Phys. Chem. Chem. Phys., 2013, 15, 761–763 RSC
.
- W. Xu, J. J. Zheng, Y. A. Li, X. Gao, X. Ji and Y. G. Zhou, Nano-impact electrochemistry reveals kinetics information of metal-ion battery materials with multiple redox centers, Angew. Chem., Int. Ed., 2023, 62, e202306185 CrossRef CAS PubMed
.
- A. V. Oladeji, J. M. Courtney, M. Fernandez-Villamarin and N. V. Rees, Electrochemical metal recycling: recovery of palladium from solution and in situ fabrication of palladium-carbon catalysts via impact electrochemistry, J. Am. Chem. Soc., 2022, 144, 18562–18574 CrossRef CAS PubMed
.
- M. V. Evers, M. Bernal, B. Roldan Cuenya and K. Tschulik, Piece by piece—Electrochemical synthesis of individual nanoparticles and their performance in ORR electrocatalysis, Angew. Chem., Int. Ed., 2019, 58, 8221–8225 CrossRef CAS PubMed
.
- H. Li, X. Zhang, Z. Sun and W. Ma, Rapid screening of bimetallic electrocatalysts using single nanoparticle collision electrochemistry, J. Am. Chem. Soc., 2022, 144, 16480–16489 CrossRef CAS PubMed
.
- U. Zahra, D. A. Robinson and H. S. White, Self-terminating redox-reactivity and vacancy-induced void formation during electrochemical collision synthesis of hollow Ag/Ag2S nanoparticles, J. Phys. Chem. C, 2022, 126, 18343–18350 CrossRef CAS
.
- M. Chen, S. M. Lu, H. W. Wang and Y. T. Long, Monitoring photoinduced interparticle chemical communication in situ, Angew. Chem., 2023, 135, e202215631 CrossRef
.
- S. M. Lu, J. F. Chen, H. F. Wang, P. Hu and Y. T. Long, Mass transport and electron transfer at the electrochemical-confined interface, J. Phys. Chem. Lett., 2023, 14, 1113–1123 CrossRef CAS PubMed
.
- J. F. Lemineur, J. M. Noël, C. Combellas and F. Kanoufi, Revealing the sub-50 ms electrochemical conversion of silver halide nanocolloids by stochastic electrochemistry and optical microscopy, Nanoscale, 2020, 12, 15128–15136 RSC
.
- W. Cheng, X. F. Zhou and R. G. Compton, Electrochemical sizing of organic nanoparticles, Angew. Chem., Int. Ed., 2013, 52, 12980–12982 CrossRef CAS PubMed
.
- C. K. Terry Weatherly, M. W. Glasscott and J. E. Dick, Voltammetric analysis of redox reactions and ion transfer in water microdroplets, Langmuir, 2020, 36, 8231–8239 CrossRef CAS PubMed
.
- M. W. Glasscott, A. D. Pendergast, S. Goines, A. R. Bishop, A. T. Hoang, C. Renault and J. E. Dick, Electrosynthesis of high-entropy metallic glass nanoparticles for designer, multi-functional electrocatalysis, Nat. Commun., 2019, 10, 2650 CrossRef PubMed
.
- H. Moon and J. H. Park, In situ probing liquid/liquid interfacial kinetics through single nanodroplet electrochemistry, Anal. Chem., 2021, 93, 16915–16921 CrossRef CAS PubMed
.
- B. K. Kim, J. Kim and A. J. Bard, Electrochemistry of a single attoliter emulsion droplet in collisions, J. Am. Chem. Soc., 2015, 137, 2343–2349 CrossRef CAS PubMed
.
- E. Lebegue, F. Barriere and A. J. Bard, Lipid membrane permeability of synthetic redox DMPC liposomes investigated by single electrochemical collisions, Anal. Chem., 2020, 92, 2401–2408 CrossRef CAS PubMed
.
- J. H. Zhang and Y. G. Zhou, Single particle impact electrochemistry: Analysis of nanoparticles and biomolecules, J. Electrochem., 2019, 25, 374–385 CAS
.
- A. D. Castaneda, N. J. Brenes, A. Kondajji and R. M. Crooks, Detection of microRNA by electrocatalytic amplification: A general approach for single-particle biosensing, J. Am. Chem. Soc., 2017, 139, 7657–7664 CrossRef CAS PubMed
.
- F. Luo, F. Chen, Y. Xiong, Z. Wu, X. Zhang, W. Wen and S. Wang, Single-particle electrochemical biosensor with DNA walker amplification for ultrasensitive HIV-DNA counting, Anal. Chem., 2021, 93, 4506–4512 CrossRef CAS PubMed
.
- P. Pandey, N. Bhattarai, L. Su, X. Wang, F. Leng, B. Gerstman, P. P. Chapagain and J. He, Detecting individual proteins and their surface charge variations in solution by the potentiometric nanoimpact method, ACS Sens., 2022, 7, 555–563 CrossRef CAS PubMed
.
- Y. Chen, Y. Liu, D. Wang, G. Gao and J. Zhi, Three-mediator enhanced collisions on an ultramicroelectrode for selective identification of single Saccharomyces cerevisiae, Anal. Chem., 2022, 94, 12630–12637 CrossRef CAS PubMed
.
- J. Liu, C. Ma, S. Shi, H. Liu, W. Wen, X. Zhang, Z. Wu and S. Wang, A general controllable release amplification strategy of liposomes for single-particle collision electrochemical biosensing, Biosens. Bioelectron., 2022, 207, 114182 CrossRef CAS PubMed
.
- J. Dunevall, H. Fathali, N. Najafinobar, J. Lovric, J. Wigstrom, A. S. Cans and A. G. Ewing, Characterizing the catecholamine content of single mammalian vesicles by collision–adsorption events at an electrode, J. Am. Chem. Soc., 2015, 137, 4344–4346 CrossRef CAS PubMed
.
- L. Sepunaru, S. V. Sokolov, J. Holter, N. P. Young and R. G. Compton, Electrochemical red blood cell counting: One at a time, Angew. Chem., Int. Ed., 2016, 55, 9768–9771 CrossRef CAS PubMed
.
- T. H. T. Nguyen, J. Lee, H. Y. Kim, K. M. Nam and B. K. Kim, Current research on single-entity electrochemistry for soft nanoparticle detection: Introduction to detection methods and applications, Biosens. Bioelectron., 2020, 151, 111999 CrossRef CAS PubMed
.
- H. Smida, A. Langlard, D. Ameline, C. Thobie-Gautier, M. Boujtita and E. Lebegue, Trends in single-impact electrochemistry for bacteria analysis, Anal. Bioanal. Chem., 2023, 415, 3717–3725 CrossRef CAS PubMed
.
- C. M. Sánchez-Sánchez, Imaging structure sensitive catalysis on different shape-controlled platinum nanoparticles, J. Am. Chem. Soc., 2010, 132, 5622–5624 CrossRef PubMed
.
- L. Zhang, Q. Wang, L. Li, M. N. Banis, J. Li, K. Adair, Y. Sun, R. Li, Z.-J. Zhao, M. Gu and X. Sun, Single atom surface engineering: A new strategy to boost electrochemical activities of Pt catalysts, Nano Energy, 2022, 93, 106813 CrossRef CAS
.
- K. Ngamchuea, R. O. D. Clark, S. V. Sokolov, N. P. Young, C. Batchelor-McAuley and R. G. Compton, Single oxidative collision events of silver nanoparticles: understanding the rate-determining chemistry, Chem. – Eur. J., 2017, 23, 16085–16096 CrossRef CAS PubMed
.
- X. Xiao and A. J. Bard, Observing Single nanoparticle collisions at an ultramicroelectrode by electrocatalytic amplification, J. Am. Chem. Soc., 2007, 129, 9610–9612 CrossRef CAS PubMed
.
- Y. Zhang, J. Mao, W. Ji, T. Feng, Z. Fu, M. Zhang and L. Mao, Collision of aptamer/Pt nanoparticles enables label-free amperometric detection of protein in rat brain, Anal. Chem., 2019, 91, 5654–5659 CrossRef CAS PubMed
.
- X. Qiu, H. Tang, J. Dong, C. Wang and Y. Li, Stochastic collision electrochemistry from single Pt nanoparticles: electrocatalytic amplification and MicroRNA sensing, Anal. Chem., 2022, 94, 8202–8208 CrossRef CAS PubMed
.
- J. Li, X. Liang, R. Zhong, M. Liu, X. Liu, H. L. Yan and Y. G. Zhou, Clinically applicable homogeneous assay for serological diagnosis of alpha-fetoprotein by impact electrochemistry, ACS Sens., 2022, 10, 3216–3222 CrossRef PubMed
.
- J. Yang, J. He, L. Mi, F. Han, W. Wen, X. Zhang, S. Wang and Z. Wu, Magnetic rolling circle amplification-assisted single-particle collision immunosensor for ultrasensitive detection of cardiac troponin I, Anal. Chem., 2022, 94, 12514–12522 CrossRef CAS PubMed
.
- Y. Y. Bai, Z. Wu, C. M. Xu, L. Zhang, J. Feng, D. W. Pang and Z. L. Zhang, One-to-many single entity electrochemistry biosensing for ultrasensitive detection of microRNA, Anal. Chem., 2022, 92, 853–858 CrossRef PubMed
.
- Y. Y. Bai, Y. J. Yang, Z. Wu, X. Y. Yang, M. Lin, D. W. Pang and Z. L. Zhang, Size-resolved single entity collision biosensing for dual quantification of MicroRNAs in a single run, ACS Appl. Mater. Interfaces, 2021, 13, 22254–22261 CrossRef CAS PubMed
.
- Z. Bognar, M. I. de Jonge and R. E. Gyurcsanyi, In situ silver nanoparticle coating of virions for quantification at single virus level, Nanoscale, 2022, 14, 2296–2303 RSC
.
- L. Sepunaru, K. Tschulik, C. Batchelor-McAuley, R. Gavish and R. G. Compton, Electrochemical detection of single E. coli bacteria labeled with silver nanoparticles, Biomater. Sci., 2015, 3, 816–820 RSC
.
- J. H. Zhang, Q. Shen and Y. G. Zhou, Quantification of tumor protein biomarkers from lung patient serum using nanoimpact electrochemistry, ACS Sens., 2021, 6, 2320–2329 CrossRef CAS PubMed
.
- L. Sepunaru, B. J. Plowman, S. V. Sokolov, N. P. Young and R. G. Compton, Rapid electrochemical detection of single influenza viruses tagged with silver nanoparticles, Chem. Sci., 2016, 7, 3892–3899 RSC
.
- J.-H. Zhang, M. Liu, F. Zhou, H.-L. Yan and Y.-G. Zhou, Homogeneous electrochemical immunoassay using an aggregation-collision strategy for alpha-fetoprotein detection, Anal. Chem., 2023, 95, 3045–3053 CrossRef CAS PubMed
.
- J. Liu, Y. Jiang, W. Wen, X. Zhang, Z. Wu and S. Wang, Enhanced single-particle collision electrochemistry at polysulfide functionalized microelectrodes for SARS-CoV-2 detection, ACS Sens., 2023, 8, 2011–2020 CrossRef CAS PubMed
.
- X. Qiu, Q. Dai, H. Tang and Y. Li, Multiplex assays of MicroRNAs by using single particle electrochemical collision in a single run, Anal. Chem., 2023, 95, 13376–13384 CrossRef CAS PubMed
.
- L. J. K. Weiss, P. Rinklin, B. Thakur, E. Music, H. Url, I. Kopic, D. Hoven, M. Banzet, T. von Trotha, D. Mayer and B. Wolfrum, Prototype digital lateral flow sensor using impact electrochemistry in a competitive binding assay, ACS Sens., 2022, 7, 1967–1976 CrossRef CAS PubMed
.
- W. Ma, H. Ma, J. F. Chen, Y. Y. Peng, Z. Y. Yang, H. F. Wang, Y. L. Ying, H. Tian and Y. T. Long, Tracking motion trajectories of individual nanoparticles using time-resolved current traces, Chem. Sci., 2017, 8, 1854–1861 RSC
.
- W. Ma, H. Ma, Z. Y. Yang and Y. T. Long, Single Ag nanoparticle electro-oxidation: potential-dependent current traces and potential-independent electron transfer kinetic, J. Phys. Chem. Lett., 2018, 9, 1429–1433 CrossRef CAS PubMed
.
- S. M. Oja, D. A. Robinson, N. J. Vitti, M. A. Edwards, Y. Liu, H. S. White and B. Zhang, Observation of multipeak collision behavior during the electro-oxidation of single Ag nanoparticles, J. Am. Chem. Soc., 2017, 139, 708–718 CrossRef CAS PubMed
.
- X. Li, S. Majdi, J. Dunevall, H. Fathali and A. G. Ewing, Quantitative measurement of transmitters in individual vesicles in the cytoplasm of single cells with nanotip electrodes, Angew. Chem., Int. Ed., 2015, 54, 11978–11982 CrossRef CAS PubMed
.
- J. E. Dick, Electrochemical detection of single cancer and healthy cell collisions on a microelectrode, Chem. Commun., 2016, 52, 10906–10909 RSC
.
- Y. Chen, D. Wang, Y. Liu, G. Gao and J. Zhi, Redox activity of single bacteria revealed by electrochemical collision technique, Biosens. Bioelectron., 2021, 176, 112914 CrossRef CAS PubMed
.
- J. Lee, Y. Kang, J. Chang, J. Song and B. K. Kim, Determination of serotonin concentration in single human platelets through single-entity electrochemistry, ACS Sens., 2020, 5, 1943–1948 CrossRef CAS PubMed
.
- S. T. Barlow, B. Figueroa, D. Fu and B. Zhang, Membrane tension modifies redox loading and release in single liposome electroanalysis, Anal. Chem., 2021, 93, 3876–3882 CrossRef CAS PubMed
.
- L. Huang, J. Zhang, Z. Xiang, D. Wu, X. Huang, X. Huang, Z. Liang, Z. Y. Tang and H. Deng, Faradaic counter for liposomes loaded with potassium, sodium ions, or protonated dopamine, Anal. Chem., 2021, 93, 9495–9504 CrossRef CAS PubMed
.
- H. Wang, C. Yang, H. Tang and Y. Li, Stochastic collision electrochemistry from single G-quadruplex/hemin: electrochemical amplification and MicroRNA sensing, Anal. Chem., 2021, 93, 4593–4600 CrossRef CAS PubMed
.
- M. Peng and Y.-G. Zhou, Impact electrochemical analysis of soft bio-particles: A mini review, Electrochem. Commun., 2023, 150, 107490 CrossRef CAS
.
- N. Li, M. Du, Y. Meng, H. Xue, F. Cao, H.-Y. Hsu and F. Liu, Characterization of red blood cells agglutination based on stochastic collision electrochemical technique, Sens. Actuators, B, 2021, 349, 130817 CrossRef CAS
.
- J. E. Dick, A. T. Hilterbrand, L. M. Strawsine, J. W. Upton and A. J. Bard, Enzymatically enhanced collisions on ultramicroelectrodes for specific and rapid detection of individual viruses, Proc. Natl. Acad. Sci. U. S. A., 2016, 113, 6403–6408 CrossRef CAS PubMed
.
- H. Smida, F. X. Lefèvre, C. Thobie-Gautier, M. Boujtita, C. M. Paquete and E. Lebègue, Single electrochemical impacts of Shewanella oneidensis MR-1 bacteria for living cells adsorption onto a polarized ultramicroelectrode surface, ChemElectroChem, 2022, 10, e202200906 CrossRef
.
- J. Lee, Z. Gerelkhuu, J. Song, K. H. Seol, B. K. Kim and J. Chang, Stochastic electrochemical cytometry of human platelets via particle collision approach, ACS Sens., 2019, 4, 3248–3256 CrossRef CAS PubMed
.
- J. E. Dick, C. Renault and A. J. Bard, Observation of single-protein and DNA macromolecule collisions on ultramicroelectrodes, J. Am. Chem. Soc., 2015, 137, 8376–8379 CrossRef CAS PubMed
.
- P.-M. Chung, Y.-T. Lin and G.-J. Wang, Au/Ni coaxial nanopillar microarray electrodes for red
blood cell concentration detection, IEEE Sens. J., 2023, 23, 4694–4699 CAS
.
Footnote |
† These authors contributed equally. |
|
This journal is © The Royal Society of Chemistry 2024 |
Click here to see how this site uses Cookies. View our privacy policy here.