Metabolomics study on southern hard clams (Mercenaria campechiensis) response to diclofenac exposure†
Received
27th August 2022
, Accepted 4th November 2022
First published on 7th November 2022
Abstract
The exposure of ecologically critical invertebrates to pharmaceutically active compounds in aquatic environments has been one of the major concerns over the past decade, which also adds serious risk to the aquatic ecosystem. However, the metabolic level perturbations in invertebrates in response to sub-lethal doses of pharmaceuticals are still rarely studied, especially in the marine coastal environment. In this study, the diclofenac regulation of southern hard clam Mercenaria campechiensis metabolites at different time points and concentrations was investigated using NMR-based metabolomics. As a result, clam metabolic profile perturbations were observed under both low and high concentrations of diclofenac exposure in one week according to principal component analysis (PCA) and partial least squares-discriminant analysis (PLS-DA); however, the potential influenced metabolic pathways were distinctly different. The low-concentration group showed significant taurine upregulation, which indicated self-protection from osmotic stress. However, the metabolites succinate, alanine, and glutamate were significantly upregulated at the relatively high concentration of diclofenac, which was a sign of anaerobic activities. The metabolic profile perturbations in week 2 showed high similarity in both low- and high-concentration groups, and the osmotic protectants betaine and taurine were significantly downregulated. The study indicated the early markers of diclofenac exposure in M. campechiensis, which provided pioneering results for monitoring the toxicity of pharmaceuticals to marine coastal water.
Environmental significance
Pharmaceutically active compounds in the aquatic environment have drawn serious concerns to the aquatic ecosystem. However, the metabolic level perturbations in marine coastal invertebrates in response to pharmaceuticals are rarely studied. In this study, NMR-based metabolomics was applied to the southern hard clam M. campechiensis exposed to different sublethal doses of diclofenac for the targeted metabolic pathway analysis and early marker discovery. The clam metabolic profile perturbations observed under early-stage low concentrations of diclofenac exposure were distinctly different from the higher concentrations or relatively long-term groups. The low-concentration group showed significant taurine upregulation, which indicated self-protection from osmotic stress. However, the metabolites succinate, alanine, and glutamate were significantly upregulated at the relatively high concentration of diclofenac, which was a sign of anaerobic activities. The potential metabolic marker discovery is expected to provide powerful tools for the future prediction and control of diclofenac contamination of the marine environment.
|
1. Introduction
Pharmaceuticals and personal care products (PPCPs) have drawn increasing concern over the last decade due to their persistence in the environment and potential effects on non-target organisms.1–4 Nonsteroidal anti-inflammatory drugs (NSAIDs) are one of the major classes of PPCPs that have been used globally5 and have been constantly released to the aquatic environment due to universal consumption, low human metabolic capability, improper disposal, and incomplete removal from wastewater treatment plants.6,7 Diclofenac is one prescribed NSAID that has been widely detected in various aquatic ecosystems ranging from the ng L−1 to μg L−1 range in surface seawater8,9 and from ng g−1 to μg g−1 in aquatic organisms.10,11 Diclofenac has been added to the first European Union Water Framework Directive Watch List in 2015 (ref. 12) and for continuous monitoring.13 Diclofenac is biologically active at low doses to the targeted organism such as humans with a specific mechanism of action; therefore, its nontarget organisms in the aquatic environment are concerned by various studies. The adverse effect of diclofenac has been studied in several nontarget organisms such as marine mussels (Mytilus galloprovincialis),14–16 zebrafish (Danio rerio),17 rainbow trout (Oncorhynchus mykiss),18,19 freshwater invertebrates (Hyalella azteca),10 common carp (Cyprinus carpio),20 brown trout (Salmo trutta f. fario),21,22 and three-spined stickleback (Gasterosteus aculeatus).23
The increase of pharmaceutical pollutants in marine coastal water is mainly due to the increased population growth rates in coastal areas, increased human activities, and the low removal rates of pharmaceutics from wastewater treatment plants.24 However, current research is highly focused on freshwater species and acute toxicity with limited studies on pharmaceutical pollutant influences in the marine environment.25 Only a few studies have investigated diclofenac metabolism in marine aquatic organisms;14,26 however, early marker studies are rare. Marine invertebrates, especially bivalves, have special filter feeding and respiration capabilities and serve as the key elements of the marine aquatic food chain. They are one of the most threatened marine organisms by pharmaceutical contaminants in marine surface water systems.27 Southern hard clam Mercenaria campechiensis is distributed primarily along the Atlantic coast from North Carolina to Florida and in the Gulf of Mexico.28 Hard clams are commercially harvested and the most valuable commercially harvested clams in the United States.29 Therefore, the M. campechiensis selected in this study also plays a critical role in human health.
Metabolomics has been employed in environmental stressor studies; however, only limited metabolomics applications have been reported in marine biology studies.30–34 Recently, metabolomics has been applied to the organ-specific of giant clams Tridacna maxima affected by marine plastic ingestion in the Red Sea.35 The metabolomics approaches in analyzing the environmental stressors and ecotoxicology can measure the simultaneous and combinational metabolite changes and provide the biological functioning of the target organisms at the molecular level.36,37 Nuclear magnetic resonance (NMR) based metabolomics has advantages in the limited sample pre-treatment, non-destructive measurement, and high reproducibility,38–40 which is an excellent tool for early marker studies.41 In addition, recent developments in NMR have shown increased sensitivity42 and improved spectra resolution,43 which provide abilities for more comprehensive approaches in NMR-based metabolomics studies. Though metabolomic profiling of the diclofenac effects on freshwater invertebrate Hyalella Azteca,10 and marine mussels Mytilus galloprovincialis15 has been reported, the early marker and dose study is still in the very early stage. In this study, the potential effects of diclofenac exposure under sub-lethal doses at different time points were evaluated using the southern hard clam M. campechiensis, which will provide pioneering results for ecotoxicological studies of marine invertebrates under pharmaceutical exposures.
2. Materials and methods
2.1 Experimental design and sample collection
Live adult southern hard clams M. campechiensis were purchased from Bay Shellfish Co., Florida. All the clams were cleaned and cultured in fresh seawater under 12 h light/dark photoperiods in aerated glass aquaria for two months before the experiment. All the experiments were carried out under 26.5 ± 0.5 °C with the dissolved oxygen (DO) ranges from 6.24 to 6.34 mg L−1 and 33.62 to 33.85 ppt salinity. The chemicals applied in the experiment including diclofenac, methanol, and chloroform were purchased from Fisher Scientific.
Three groups were designed including one control group with no diclofenac and two experiment groups with 1 mg L−1 and 5 mg per L diclofenac. Fresh seawater and the ones with the corresponding concentrations of diclofenac in seawater were changed daily. Adult clams with lengths ranging from 4.8 to 5.4 cm were spread out in 6 aquariums with 6–7 clams in each aquarium. The clams were fed with phytoplankton daily. Two experiment time points were designed, and the clam samples were collected on day 7 (1 week) and day 14 (2 weeks). All samples were immediately frozen in liquid nitrogen to stop any enzymatic or chemical reactions for further usage. The frozen samples were stored in a −80 °C freezer before further analysis.
2.2 Sample preparation and 1H NMR acquisition
All the frozen clam samples were pre-thawed on ice before the sample preparation. The digestive gland samples (0.5 ± 0.05 g) from the dissection of the clams were collected and transferred into homogenization tubes. The sample was extracted using a previously reported method44 using water, methanol, and chloroform with slight changes. The polar metabolites in the methanol/water phase were freeze-dried by using a Savant SpeedVac SPD 120 vacuum concentrator. The dried samples were resuspended using phosphate buffer with deuterium water (D2O), and the final samples contained 10% of D2O with 0.1 M phosphate buffer (pH = 7.4) and 0.5 mM trimethylsilylpropanoic acid (TSP). All the NMR data were acquired using presaturation experiments using a Joel 400 MHz high-resolution NMR.
2.3 Data analysis
All the NMR data were preprocessed using Delta 5.3.3 including calibration, phasing, and baseline correction. The NMR data were exported to text files for further analysis. All the text files were imported to Matlab (MathWorks) for peak bucketing calculations. The peak patterns were determined using a previously reported approach45 with slight changes. The NMR peak identification was carried out using Chenomx 8.6 (Chenomx Inc.), and a representative spectrum with the assignment is listed in Fig. S1.† PCA and PLS-DA with cross-validation were carried out using PLS-Toolbox (Eigenvector Research), and the boxplots were carried out using MetaboAnalyst 5.0. The Matthews correlation coefficient was used to evaluate the cross-validation of the PLS-DA model and a high score indicates good results in combined confusion matrix factors including true positives, false negatives, true negatives, and false positives.46
3. Results
3.1 Clam physical observation
No clam sample was dead during the week 1 experiment in all six groups. On day 14, only one dead clam was noticed in the high-concentration diclofenac tank. All the other clams were alive during the harvesting time.
3.2 Metabolic profiling changes in week 1
The metabolomic level perturbation was observed at week 1 in both 1 mg L−1 and 5 mg L−1 groups of diclofenac treatment according to the PCA study; however, the PCA score plot showed that the major metabolite changes were different (Fig. 1). PLS-DA studies have been applied between control and 1 mg L−1, and control and 5 mg per L diclofenac to show the changes. Both models showed relatively reliable results after cross-validation (Fig. 3), which also indicated the metabolite level changes.
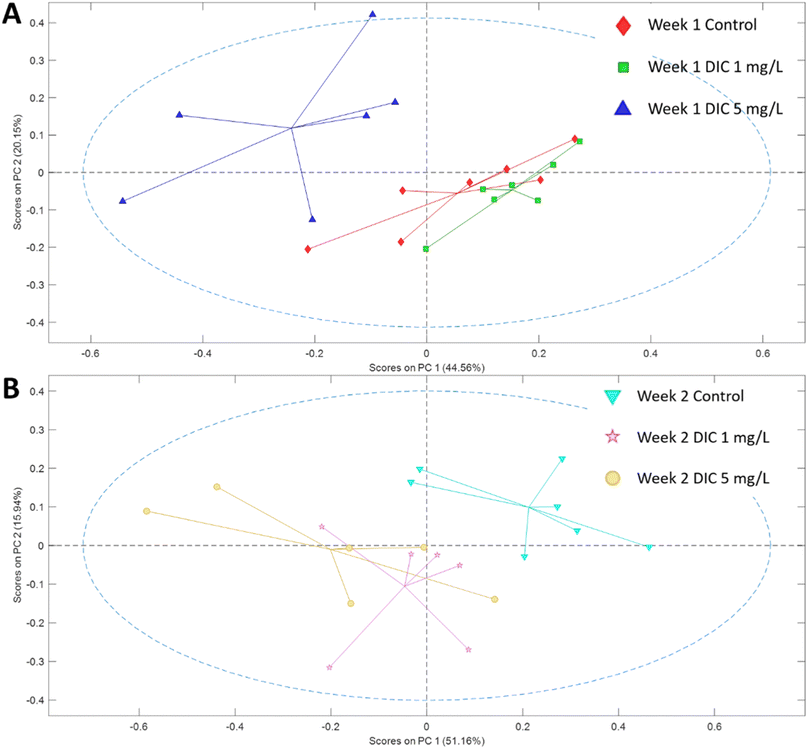 |
| Fig. 1 PCA score plot of the metabolites for control and two treatment groups in (A) week 1 and (B) week 2. The markers in one group were connected to the center of the group data. | |
The low concentration diclofenac group (1 mg L−1) showed significant upregulation of amino acids isoleucine, leucine, valine, taurine, lysine, and tyrosine; however, these amino acids except leucine were not significantly changed in the higher concentration diclofenac group (5 mg L−1). In a contrast, the high-concentration diclofenac group showed significant upregulation of succinate, leucine, glutamate, and alanine and significantly low regulation of betaine (Table 1 and Fig. 3).
Table 1 Metabolite fold change (FC) and p values based on the Student's t-test. FC was calculated using the experimental group with respect to the control group. The metabolites are listed in the alphabetical order
ppm |
Metabolites |
Week 1 |
Week 2 |
Case 1 mg L−1vs. control |
Case 5 mg L−1vs. control |
Case 1 mg L−1vs. control |
Case 5 mg L−1vs. control |
P values |
FC |
P values |
FC |
P values |
FC |
P values |
FC |
2.27 |
Acetoacetate |
1.12 × 10−1 |
0.88 |
2.55 × 10−1 |
0.92 |
7.59 × 10−1 |
0.98 |
6.09 × 10−1 |
0.98 |
1.5 |
Alanine |
2.95 × 10−1 |
0.93 |
3.15 × 10−2 |
1.33 |
2.77 × 10−1 |
1.19 |
3.93 × 10−3 |
1.52 |
8.6 |
AMP |
6.02 × 10−2 |
1.05 |
4.92 × 10−1 |
0.98 |
2.88 × 10−1 |
0.96 |
8.61 × 10−1 |
1.01 |
2.78 |
Aspartate |
2.12 × 10−1 |
1.06 |
7.23 × 10−2 |
0.92 |
6.34 × 10−2 |
0.86 |
5.75 × 10−1 |
0.96 |
8.53 |
ATP |
8.27 × 10−1 |
1.01 |
1.15 × 10−2 |
0.93 |
5.90 × 10−2 |
0.92 |
1.28 × 10−1 |
0.95 |
6.15 |
ATP/AMP |
2.72 × 10−1 |
1.02 |
1.36 × 10−1 |
0.97 |
2.55 × 10−1 |
0.97 |
6.80 × 10−1 |
0.99 |
3.91 |
Betaine |
2.48 × 10−1 |
1.02 |
2.94 × 10−2 |
0.95 |
8.02 × 10−3 |
0.91 |
5.98 × 10−3 |
0.87 |
3.2 |
Choline |
5.39 × 10−3 |
0.88 |
1.01 × 10−1 |
0.92 |
1.37 × 10−1 |
0.92 |
1.85 × 10−1 |
0.95 |
1.94 |
D-Arginine |
1.66 × 10−1 |
1.04 |
7.41 × 10−1 |
1.01 |
9.92 × 10−1 |
1.00 |
4.79 × 10−1 |
1.03 |
2.74 |
Dimethylamine |
1.35 × 10−2 |
1.15 |
8.75 × 10−2 |
0.94 |
1.35 × 10−1 |
0.92 |
4.51 × 10−1 |
0.96 |
4.64 |
Glucose |
5.72 × 10−2 |
1.06 |
4.54 × 10−1 |
0.98 |
2.96 × 10−1 |
0.96 |
7.78 × 10−1 |
1.01 |
2.35 |
Glutamate |
1.20 × 10−1 |
0.95 |
1.40 × 10−2 |
1.11 |
5.98 × 10−1 |
0.96 |
2.80 × 10−2 |
1.11 |
3.57 |
Glycine |
1.10 × 10−1 |
1.20 |
4.59 × 10−1 |
1.06 |
5.29 × 10−4 |
2.36 |
6.22 × 10−2 |
1.52 |
5.42 |
Glycogen |
1.89 × 10−2 |
1.17 |
2.36 × 10−1 |
0.92 |
6.72 × 10−1 |
1.04 |
4.19 × 10−1 |
1.11 |
7.05 |
Histidine |
4.67 × 10−1 |
0.60 |
8.90 × 10−1 |
1.03 |
2.34 × 10−1 |
2.09 |
5.53 × 10−1 |
1.38 |
2.63 |
Hypotaurine |
2.92 × 10−2 |
0.85 |
9.01 × 10−1 |
0.99 |
2.93 × 10−1 |
1.11 |
1.39 × 10−1 |
1.14 |
0.94 |
Isoleucine |
3.37 × 10−3 |
1.09 |
4.26 × 10−1 |
1.02 |
9.15 × 10−1 |
1.00 |
1.92 × 10−1 |
1.08 |
1.35 |
Lactate |
3.93 × 10−2 |
1.06 |
7.95 × 10−1 |
0.98 |
5.48 × 10−1 |
0.97 |
2.96 × 10−1 |
1.06 |
0.97 |
Leucine |
5.67 × 10−3 |
1.09 |
5.15 × 10−3 |
1.09 |
4.46 × 10−1 |
1.03 |
2.14 × 10−2 |
1.16 |
3.01 |
Lysine |
3.92 × 10−2 |
1.07 |
4.42 × 10−1 |
0.97 |
4.65 × 10−1 |
0.95 |
4.64 × 10−1 |
1.05 |
3.12 |
Malonate |
1.26 × 10−2 |
0.85 |
4.02 × 10−1 |
0.94 |
1.70 × 10−1 |
0.91 |
3.14 × 10−1 |
1.07 |
2.14 |
Methionine |
2.34 × 10−1 |
1.02 |
1.74 × 10−1 |
1.02 |
2.05 × 10−1 |
0.96 |
3.07 × 10−1 |
1.04 |
4.07 |
Myo-inositol |
8.33 × 10−1 |
0.99 |
4.35 × 10−1 |
1.05 |
6.67 × 10−1 |
0.98 |
6.97 × 10−2 |
1.15 |
2.93 |
N,N-Dimethylglycine |
8.85 × 10−3 |
1.10 |
2.17 × 10−1 |
0.95 |
2.08 × 10−1 |
0.91 |
7.57 × 10−1 |
0.98 |
3.21 |
O-Phosphocholine |
6.21 × 10−1 |
0.98 |
1.02 × 10−1 |
0.91 |
1.75 × 10−1 |
0.90 |
5.29 × 10−1 |
0.97 |
7.32 |
Phenylalanine |
6.00 × 10−2 |
1.05 |
5.93 × 10−1 |
0.99 |
2.09 × 10−1 |
0.96 |
8.03 × 10−1 |
1.01 |
8.86 |
Pyrimidine |
8.43 × 10−2 |
1.04 |
5.80 × 10−1 |
0.99 |
2.04 × 10−1 |
0.95 |
7.78 × 10−1 |
1.01 |
3.23 |
sn-Glycero-3-phosphocholine |
9.07 × 10−1 |
0.99 |
8.35 × 10−2 |
0.88 |
8.56 × 10−1 |
0.98 |
4.12 × 10−1 |
0.93 |
2.4 |
Succinate |
6.19 × 10−1 |
0.95 |
2.49 × 10−3 |
4.70 |
3.32 × 10−1 |
1.41 |
4.63 × 10−4 |
3.00 |
4.37 |
Tartrate |
7.23 × 10−1 |
1.02 |
7.25 × 10−1 |
1.04 |
8.08 × 10−2 |
0.82 |
2.61 × 10−1 |
0.87 |
3.45 |
Taurine |
3.77 × 10−2 |
1.07 |
5.60 × 10−1 |
0.99 |
4.10 × 10−2 |
0.93 |
1.26 × 10−2 |
0.93 |
4.45 |
Trigonelline |
2.20 × 10−1 |
1.03 |
3.79 × 10−1 |
1.01 |
4.93 × 10−1 |
0.98 |
2.81 × 10−1 |
1.04 |
6.9 |
Tyrosine |
4.65 × 10−2 |
1.05 |
9.55 × 10−1 |
1.00 |
2.69 × 10−1 |
0.96 |
4.19 × 10−1 |
1.03 |
1.04 |
Valine |
3.74 × 10−2 |
1.06 |
2.50 × 10−1 |
1.03 |
4.68 × 10−1 |
0.97 |
1.86 × 10−1 |
1.07 |
3.3 Metabolite changes in week 2
The week 2 data showed that both diclofenac concentrations have high perturbations in the metabolomic level according to the PCA study; however, different from week 1, the low concentration diclofenac group showed very similarly changing patterns with the high concentration diclofenac group. Both diclofenac treatment groups showed metabolite level perturbation according to the PLS-DA study and the cross-validation (Fig. 2 and 3). The PLS-DA loadings showed very similar important features including taurine, betaine, succinate, and glycine. Similarly, the t-test showed significant upregulation of glycine (p < 0.06) and downregulation of betaine and taurine (p < 0.05). The succinate was highly upregulated in both groups but was only significant in the high-concentration group. In addition, alanine, leucine, and glutamate showed upregulation only in the high-concentration group (Table 1).
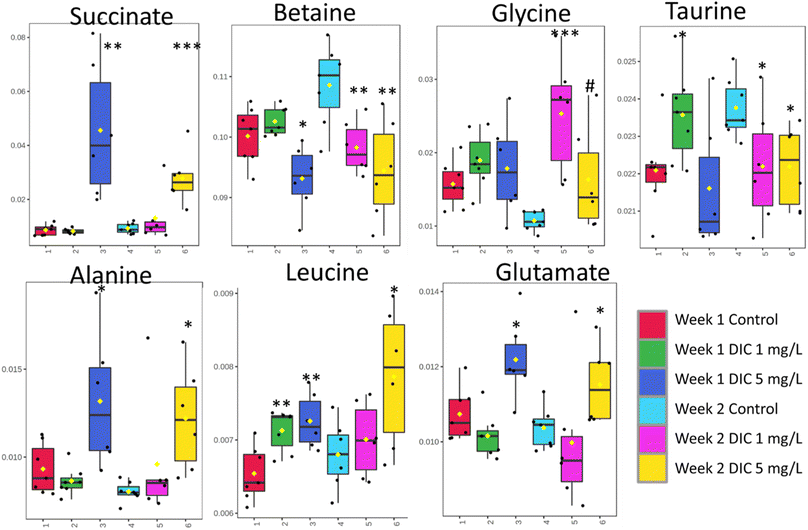 |
| Fig. 2 The box plots of the selected metabolites showed high separation in all the study groups. Student's t-tests were carried out at each time point and were performed using the experiment samples vs. their control group, repetitively. #p < 0.1, *p < 0.05, **p < 0.01, and ***p < 0.001. | |
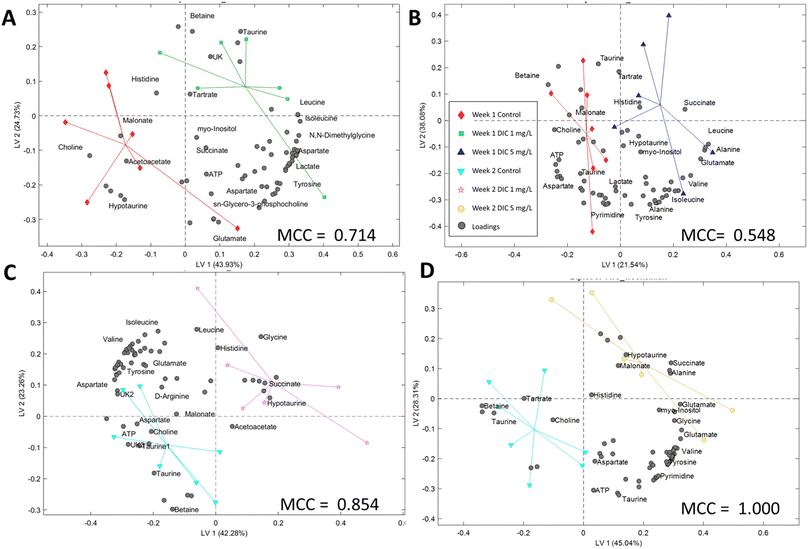 |
| Fig. 3 The PLS-DA study for all the treatments vs. the control. (A) Week 1 1 mg L−1vs. control, (B) week 1 5 mg L−1vs. control, (C) week 2 1 mg L−1vs. control, and (D) week 2 5 mg L−1vs. control. High Matthews correlation coefficients (MCCs) indicate the high reliability of the models. | |
4. Discussion
4.1 Diclofenac concentration effect on clam metabolites
Succinate is one critical tricarboxylic acid (TCA) cycle intermediate, which was significantly and dramatically upregulated only in the high concentration diclofenac treatment (FC = 4.70 and p = 0.003 for week 1 and FC = 0.87 and p = 0.0005 for week 2). The phenomenon was only observed in the high concentration diclofenac treatment and the low concentration diclofenac treatment has no significant succinate changes in both week 1 (FC = 0.95 and p = 0.619) and week 2 (FC = 1.41 and p = 0.332) groups. The accumulation of succinate in clams were reported as an anaerobic activity,47 which indicated that the high concentration of succinate in the tissue was potentially due to the anaerobic activity. Similarly, alanine and glutamate where both are close metabolites to the TCA pathway also showed a similar result to succinate. Both metabolites were significantly upregulated in the high-concentration diclofenac group, which further illustrated the potential anaerobic activity.48 The results indicated that the higher concentration of diclofenac is critical in contributing to the potential anaerobic activities.
4.2 Metabolic perturbations under different exposure times
Betaine showed a significant downregulation after one week in both low concentration (FC = 0.91 and p = 0.008) and high concentration (FC = 0.87 and p = 0.006) diclofenac groups. Betaine was considered to serve as one of the key organic osmolytes in marine animal tissues and extracellular fluid to balance the high osmolality of seawater.49 The depletion of betaine indicated that a high concentration of diclofenac or longer exposure to a low concentration of diclofenac inhibited the potential cytoprotective effect of betaine and reduced cellular impacts of environmental stress.50
Similarly, another important osmolyte taurine showed a very similar behavior to betaine, which is further evidence that diclofenac has potential osmolyte stress on clams. Unlike the anaerobic activity, which was only observed in the 5 mg L−1 groups in week 1, the betaine and taurine downregulation were observed in week 2 for both concentrations. The low concentration and short-term group (1 week) showed neglectable changes of betaine (FC = 1.02 and p = 0.248) and a significantly higher concentration of taurine (FC = 1.07 and p = 0.038). The phenomena indicated that taurine is a potential sign of self-protective response in clam osmotic regulation to diclofenac exposure in the early-stage and relatively low concentration; however, the protection cannot last long or under a relatively high concentration, which could likely break despite the protection effect.
Glucose levels were significantly upregulated (FC = 1.06 and p = 0.057) only in the low concentration diclofenac (1 mg L−1) group at week 1, which indicated the potential energy uptake under the initial low concentration of diclofenac stressor. This can be further evidenced in the amino acids including isoleucine (FC = 1.09 and p = 0.003), valine (FC = 1.06 and p = 0.0037), and tyrosine (FC = 1.05 and p = 0.047), which only showed significant upregulation in the low concentration diclofenac group at week 1. The upregulation at an early-stage low concentration of diclofenac was also observed in lactate (FC = 1.06 and p = 0.039), which is potentially due to the initial stage of anaerobic activity after taking an increased amount of glucose.
In the PLS-DA study, models were successfully built for the classification of the experimental groups and control groups, which indicated the potential classification using the metabolic profiling data. However, the model accuracy was different according to the Matthews correlation coefficient (MCC) values. The second week MCC values are higher than 0.85, which indicates high accuracy in cross-validation. The PLS-DA model can be potentially used to classify the diclofenac exposure using the whole metabolic profile instead of a single metabolite in the two week treatment. The first-week model is solid but less accurate (MCC > 0.50), which also indicates that the metabolic level changes were mainly in the targeted pathways rather than the whole profile. The PLS-DA important loadings (metabolites) have high similarity to the t-test results,51 and can be found in the t-test study sections.
In summary, the early stage upregulations of taurine, glucose, lactate and amino acids indicated that the potential self-protecting adjustment over low concentrations of diclofenac stress is likely from the anaerobic and osmotic regulations, and the self-protection is only effective at a relatively low concentration and short term diclofenac exposure.
5. Conclusion
Diclofenac is one of the most commonly used NSAID and has been constantly monitored in aquatic environments and creatures. Though diclofenac has shown adverse effects on various freshwater creatures, studies about its toxic effects on metabolite perturbations in marine organisms are rare. Both the concentration and exposure time study can reveal the marine animal potential biomarkers under the stress of diclofenac. In our study, 1H NMR-based metabolomics was performed to investigate the response of clams under sublethal dose exposure of diclofenac and study the regulation of clam metabolites in a short term (1 week) and relatively long term (2 weeks). As a result, though the metabolic profiles were both perturbated in the low-concentration week 1 and week 2 groups, the low concentration groups metabolic profiles were changed differently compared to the high-concentration group according to both PCA and PLS-DA studies, which indicated the potential early-stage self-protective response to low concentrations and short term exposure of diclofenac. While the short-term and low concentration of diclofenac groups indicated significantly upregulated taurine, tyrosine, isoleucine, and lactate, the other groups showed significant upregulation of succinate, alanine, and glutamate. Moreover, the key organic osmolytes betaine and taurine showed significant downregulation in the long-term low concentration of the diclofenac group and the two high-concentration groups. In summary, the study showed that M. campechiensis was able to show short-term resistance to relatively low concentrations of diclofenac exposure by self-protection in clam osmotic regulation and anaerobic activities; however, long-term or relatively high concentrations of diclofenac could significantly perturb the osmotic mechanism. The study indicated that the metabolomics of the digestive gland of M. campechiensis is a feasible method for monitoring the toxicity of pharmaceuticals in the marine environment.
Conflicts of interest
There are no conflicts to declare.
Acknowledgements
We would like to thank the New College of Florida student Lexi Fox for helping us in culturing the clams. LJ would like to acknowledge the financial support of the New College of Florida. BW's research material is based upon work supported in part by the National Science Foundation under Grant No. CHE-2245530.
References
- F. S. Freyria, F. Geobaldo and B. Bonelli, Nanomaterials for the Abatement of Pharmaceuticals and Personal Care Products from Wastewater, Appl. Sci., 2018, 8(2), 16 CrossRef.
- K. Patterson, K. Howlett, K. Patterson, B. Wang and L. Jiang, Photodegradation of ibuprofen and four other pharmaceutical pollutants on natural pigments sensitized TiO2 nanoparticles, Water Environ. Res., 2020, 92(8), 1152–1161 CrossRef.
- A. B. Boxall, M. A. Rudd, B. W. Brooks, D. J. Caldwell, K. Choi and S. Hickmann,
et al., Pharmaceuticals and personal care products in the environment: what are the big questions?, Environ. Health Perspect., 2012, 120(9), 1221–1229 CrossRef PubMed.
- Z. Wang, G. W. Walker, D. C. Muir and K. Nagatani-Yoshida, Toward a global understanding of chemical pollution: a first comprehensive analysis of national and regional chemical inventories, Environ. Sci. Technol., 2020, 54(5), 2575–2584 CrossRef PubMed.
- S. C. Monteiro and A. Boxall, Occurrence and fate of human pharmaceuticals in the environment, Rev. Environ. Contam. Toxicol., 2010, 53–154 Search PubMed.
- X. Yang, R. C. Flowers, H. S. Weinberg and P. C. Singer, Occurrence and removal of pharmaceuticals and personal care products (PPCPs) in an advanced wastewater reclamation plant, Water Res., 2011, 45(16), 5218–5228 CrossRef.
- S. Anliker, M. Patrick, K. Fenner and H. Singer, Quantification of Active Ingredient Losses from Formulating Pharmaceutical Industries and Contribution to Wastewater Treatment Plant Emissions, Environ. Sci. Technol., 2020, 54(23), 15046–15056 CrossRef.
- R. Loos, R. Carvalho, D. C. António, S. Comero, G. Locoro and S. Tavazzi,
et al., EU-wide monitoring survey on emerging polar organic contaminants in wastewater treatment plant effluents, Water Res., 2013, 47(17), 6475–6487 CrossRef.
- N. A. Munz, Q. Fu, C. Stamm and J. Hollender, Internal concentrations in gammarids reveal increased risk of organic micropollutants in wastewater-impacted streams, Environ. Sci. Technol., 2018, 52(18), 10347–10358 CrossRef PubMed.
- Q. Fu, A. Scheidegger, E. Laczko and J. Hollender, Metabolomic profiling and toxicokinetics modeling to assess the effects of the pharmaceutical diclofenac in the aquatic invertebrate Hyalella azteca, Environ. Sci. Technol., 2021, 55(12), 7920–7929 CrossRef.
- M. Capolupo, S. Franzellitti, A. Kiwan, P. Valbonesi, E. Dinelli and E. Pignotti,
et al., A comprehensive evaluation of the environmental quality of a coastal lagoon (Ravenna, Italy): Integrating chemical and physiological analyses in mussels as a biomonitoring strategy, Sci. Total Environ., 2017, 598, 146–159 CrossRef.
-
N. Nugent and M. Rhinard, The European Commission, Bloomsbury Publishing, 2015 Search PubMed.
- E. Simon, A. Duffek, C. Stahl, M. Frey, M. Scheurer and J. Tuerk,
et al., Biological effect and chemical monitoring of Watch List substances in European surface waters: steroidal estrogens and diclofenac–effect-based methods for monitoring frameworks, Environ. Int., 2022, 159, 107033 CrossRef PubMed.
- B. Bonnefille, L. Arpin-Pont, E. Gomez, H. Fenet and F. Courant, Metabolic profiling identification of metabolites formed in Mediterranean mussels (Mytilus galloprovincialis) after diclofenac exposure, Sci. Total Environ., 2017, 583, 257–268 CrossRef CAS PubMed.
- B. Bonnefille, E. Gomez, M. Alali, D. Rosain, H. Fenet and F. Courant, Metabolomics assessment of the effects of diclofenac exposure on Mytilus galloprovincialis: potential effects on osmoregulation and reproduction, Sci. Total Environ., 2018, 613, 611–618 CrossRef.
- F. Courant, L. Arpin-Pont, B. Bonnefille, S. Vacher, M. Picot-Groz and E. Gomez,
et al., Exposure of marine mussels to diclofenac: modulation of prostaglandin biosynthesis, Environ. Sci. Pollut. Res., 2018, 25(7), 6087–6094 CrossRef.
- N. Klüver, K. Bittermann and B. I. Escher, QSAR for baseline toxicity and classification of specific modes of action of ionizable organic chemicals in the zebrafish embryo toxicity test, Aquat. Toxicol., 2019, 207, 110–119 CrossRef.
- A. C. Mehinto, E. M. Hill and C. R. Tyler, Uptake and biological effects of environmentally relevant concentrations of the nonsteroidal anti-inflammatory pharmaceutical diclofenac in rainbow trout (Oncorhynchus mykiss), Environ. Sci. Technol., 2010, 44(6), 2176–2182 CrossRef.
- J. Schwaiger, H. Ferling, U. Mallow, H. Wintermayr and R. Negele, Toxic effects of the non-steroidal anti-inflammatory drug diclofenac: part I: histopathological alterations and bioaccumulation in rainbow trout, Aquat. Toxicol., 2004, 68(2), 141–150 CrossRef.
- H. Islas-Flores, L. M. Gómez-Oliván, M. Galar-Martínez, A. Colín-Cruz, N. Neri-Cruz and S. García-Medina, Diclofenac-induced oxidative stress in brain, liver, gill and blood of common carp (Cyprinus carpio), Ecotoxicol. Environ. Saf., 2013, 92, 32–38 CrossRef.
- B. Hoeger, B. Köllner, D. R. Dietrich and B. Hitzfeld, Water-borne diclofenac affects kidney and gill integrity and selected immune parameters in brown trout (Salmo trutta f. fario), Aquat. Toxicol., 2005, 75(1), 53–64 CrossRef.
- S. Schwarz, H. Schmieg, M. Scheurer, H.-R. Köhler and R. Triebskorn, Impact of the NSAID diclofenac on survival, development, behaviour and health of embryonic and juvenile stages of brown trout, Salmo trutta f. fario, Sci. Total Environ., 2017, 607, 1026–1036 CrossRef.
- J. Näslund, J. Fick, N. Asker, E. Ekman, D. J. Larsson and L. Norrgren, Diclofenac affects kidney histology in the three-spined stickleback (Gasterosteus aculeatus) at low μg/L concentrations, Aquat. Toxicol., 2017, 189, 87–96 CrossRef.
- H. Fenet, L. Arpin-Pont, A. Vanhoutte-Brunier, D. Munaron, A. Fiandrino and M.-J. M. Bueno,
et al., Reducing PEC uncertainty in coastal zones: a case study on carbamazepine, oxcarbazepine and their metabolites, Environ. Int., 2014, 68, 177–184 CrossRef.
- G. Aguirre-Martínez, T. Del Valls and M. Martín-Díaz, Early responses measured in the brachyuran crab Carcinus maenas exposed to carbamazepine and novobiocin: application of a 2-tier approach, Ecotoxicol. Environ. Saf., 2013, 97, 47–58 CrossRef.
- C. Trombini, M. Hampel and J. Blasco, Assessing the effect of human pharmaceuticals (carbamazepine, diclofenac and ibuprofen) on the marine clam Ruditapes philippinarum: an integrative and multibiomarker approach, Aquat. Toxicol., 2019, 208, 146–156 CrossRef PubMed.
- F. Gagné, C. Blaise, M. Fournier and P. Hansen, Effects of selected pharmaceutical products on phagocytic activity in Elliptio complanata mussels, Comp. Biochem. Physiol., Part C: Toxicol. Pharmacol., 2006, 143(2), 179–186 Search PubMed.
- R. T. Dillon Jr and J. Manzi, Genetics and shell morphology of hard clams (genus Mercenaria) from Laguna Madre, Nautilus, 1989, 103(2), 73–77 Search PubMed.
-
E. Pritchard, Fisheries of the United States 2003, National Marine Fisheries Service, Office of Science and Technology, Fisheries Statistics Division, Silver Spring, MD, 2004 Search PubMed.
- R. J. Van Meter, D. A. Glinski, S. T. Purucker and W. M. Henderson, Influence of exposure to pesticide mixtures on the metabolomic profile in post-metamorphic green frogs (Lithobates clamitans), Sci. Total Environ., 2018, 624, 1348–1359 CrossRef PubMed.
- M. G. Miller, Environmental metabolomics: a SWOT analysis (strengths, weaknesses, opportunities, and threats), J. Proteome Res., 2007, 6(2), 540–545 CrossRef PubMed.
- V. Aru, G. Sarais, F. Savorani, S. B. Engelsen and F. C. Marincola, Metabolic responses of clams, Ruditapes decussatus and Ruditapes philippinarum, to short-term exposure to lead and zinc, Mar. Pollut. Bull., 2016, 107(1), 292–299 CrossRef PubMed.
- T. Cappello, A. Giannetto, V. Parrino, M. Maisano, S. Oliva and G. De Marco,
et al., Baseline levels of metabolites in different tissues of mussel Mytilus galloprovincialis (Bivalvia: Mytilidae), Comp. Biochem. Physiol.–D: Genom. Proteom., 2018, 26, 32–39 Search PubMed.
- V. Aru, M. B. Pisano, F. Savorani, S. B. Engelsen, S. Cosentino and F. C. Marincola, Metabolomics analysis of shucked mussels' freshness, Food Chem., 2016, 205, 58–65 CrossRef PubMed.
- F. Almulhim, S. Rossbach, A.-H. Emwas, N. M. Kharbatia, L. Jaremko and M. Jaremko,
et al., Metabolomic Study on Tridacna maxima Giant Clams Reveals Metabolic Fingerprint of Environmental Pollutants, Front. Mar. Sci., 2022, 9, 813404 CrossRef.
- B. Wang, C. Habermehl and L. Jiang, Metabolomic analysis of honey bee (Apis mellifera L.) response to glyphosate exposure, Mol. Omics, 2022, 18(7), 635–642 RSC.
- A.-H. Emwas, K. Szczepski, B. G. Poulson, K. Chandra, R. T. McKay and M. Dhahri,
et al., NMR as a “gold standard” method in drug design and discovery, Molecules, 2020, 25(20), 4597 CrossRef PubMed.
- B. Wang, S. Sheriff, A. Balasubramaniam and M. A. Kennedy, NMR based metabolomics study of Y2 receptor activation by neuropeptide Y in the SK-N-BE2 human neuroblastoma cell line, Metabolomics, 2015, 11(5), 1243–1252 CrossRef.
- B. Wang, A. M. Goodpaster and M. A. Kennedy, Coefficient of variation, signal-to-noise ratio, and effects of normalization in validation of biomarkers from NMR-based metabonomics studies, Chemom. Intell. Lab. Syst., 2013, 128, 9–16 CrossRef PubMed.
- L. Jiang, H. Sullivan and B. Wang, Principal component analysis (PCA) loading and statistical tests for nuclear magnetic resonance (NMR) metabolomics involving multiple study groups, Anal. Lett., 2022, 55(10), 1648–1662 CrossRef.
- A. Emwas, R. Roy, R. McKay, L. Tenori, E. Saccenti and G. Gowda,
et al.
, Metabolites, 2019, 9, 123 CrossRef PubMed.
- K. Chandra, S. Al-Harthi, S. Sukumaran, F. Almulhim, A.-H. Emwas and H. S. Atreya,
et al., NMR-based metabolomics with enhanced sensitivity, RSC Adv., 2021, 11(15), 8694–8700 RSC.
- K. Bingol, D.-W. Li, B. Zhang and R. Brüschweiler, Comprehensive metabolite identification strategy using multiple two-dimensional NMR spectra of a complex mixture implemented in the COLMARm web server, Anal. Chem., 2016, 88(24), 12411–12418 CrossRef PubMed.
- L. Jiang, H. Sullivan, C. Seligman, S. Gilchrist and B. Wang, An NMR-based metabolomics study on sea anemones Exaiptasia diaphana (Rapp, 1829) with atrazine exposure, Mol. Omics, 2021, 17(6), 1012–1020 RSC.
- B. Wang, A. M. Maldonado-Devincci and L. Jiang, Evaluating line-broadening factors on a reference spectrum as a bucketing method for NMR based metabolomics, Anal. Biochem., 2020, 606, 113872 CrossRef.
- D. Chicco and G. Jurman, The advantages of the Matthews correlation coefficient (MCC) over F1 score and accuracy in binary classification evaluation, BMC Genomics, 2020, 21(1), 1–13 CrossRef.
- C. Ortmann and M. K. Grieshaber, Energy metabolism and valve closure behaviour in the Asian clam Corbicula fluminea, J. Exp. Biol., 2003, 206(22), 4167–4178 CrossRef.
- A. C. Lee, Y. C. Lee and T. S. Chin, Effects of low dissolved oxygen on the digging behaviour and metabolism of the hard clam (Meretrix lusoria), Aquacult. Res., 2012, 43(1), 1–13 CrossRef.
- M. B. Burg and J. D. Ferraris, Intracellular organic osmolytes: function and regulation, J. Biol. Chem., 2008, 283(12), 7309–7313 CrossRef.
- H. Hanana, G. Simon, N. Kervarec and S. Cérantola, Evaluation of toxicological effects induced by tributyltin in clam Ruditapes decussatus using high-resolution magic angle spinning nuclear magnetic resonance spectroscopy: study of metabolic responses in heart tissue and detection of a novel metabolite, Toxicol. Rep., 2014, 1, 777–786 CrossRef.
- B. Wang, Z. Shi, G. Weber and M. Kennedy, Introduction of a new critical p value correction method for statistical significance analysis of metabonomics
data, Anal. Bioanal. Chem., 2013, 405(26), 8419–8429 CrossRef PubMed.
|
This journal is © The Royal Society of Chemistry 2023 |