DOI:
10.1039/D3TC02375C
(Paper)
J. Mater. Chem. C, 2023,
11, 11671-11680
Supramolecular polymerization of chiral platinum(II) complexes: transformable nanoassemblies and their amplified circularly polarized luminescence†
Received
6th July 2023
, Accepted 3rd August 2023
First published on 3rd August 2023
Abstract
Herein, we report four pairs of enantiomeric platinum(II) complexes D/L-Pt-1–Pt-4 with minor structural variations that have been found to lead to distinct self-assembly behaviors and form various well-defined nanostructures. Notably, Pt-1 exhibits polymorphic self-assembly with well-defined nanostructures, such as nanorings, nanotwists, dendritic twists, and microbelts, in various conditions through the interplay of different non-covalent interactions. Importantly, two pairs of enantiomers D/L-Pt-1–Pt-2 show significantly amplified effects for circular dichroism (CD) and circularly polarized luminescence (CPL) in their corresponding nanoassemblies. Moreover, the self-assembly process can be governed by the Pt···Pt/π–π stacking, the solvophobic interactions, and electrostatic repulsion provided by the positively charged tridentate cyclometalated platinum(II) moieties. The detailed supramolecular polymerization pathway was systematically investigated by spectroscopy, X-ray single-crystal diffraction, and electron microscopy studies.
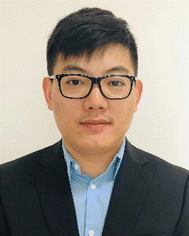
Xiaolin Zhu
| Dr Xiaolin Zhu is an Associate Professor at the Shaanxi Normal University from Dec. 2019. He received his BSc degree and PhD degree from Nanjing Tech University. After graduation, he worked as a postdoc fellow at North Dakota State University, New Jersey Institute of Technology, and San Diego State University. His research interests focus on the study of optoelectronic properties of supramolecular photo-functional materials and their applications in photocatalysis. |
Introduction
The spontaneous self-assembly of molecules through non-covalent interactions into dynamically elongated nanoarchitectures has attracted considerable interest over the past few decades owing to their potential applications in the fields of materials and life sciences.1–11 Supramolecular polymerizations have been mainly built from purely organic building blocks since the first report in 1990.11 There has been a notable advance in the understanding of the formation process of supramolecular polymerizations,8–10 which are primarily driven by the combination of various non-covalent interactions, including π–π stacking, hydrogen bonding, electrostatic, and hydrophobic–hydrophobic interactions.10–13 Inspired by the coordination polymers, the incorporation of metal centers, in particular, d8 and d10 transition metals, into systems has enabled the self-assemblies novel topological structures and interesting luminescence properties due to the presence of additional metal–metal interactions in transition metal complexes.14,15
Among the d8 and d10 transition metal complexes, square-planar d8 platinum(II) complexes have been extensively explored owing to their propensity to self-assemble into ordered nanostructures, driven by metal–metal and/or π–π stacking interactions.14–18 In addition, the Pt(II) complexes possess rich photophysical properties, such as intense absorption in the visible region and drastic color and emission changes during the process of self-assembly.18–20 These unique properties make Pt(II) complexes promising candidates for a variety of optoelectronic and biomedical applications, including organic light-emitting devices (OLEDs), photocatalysis, molecular recognition, biological imaging, and nonlinear optics.15–22 In this regard, Pt(II) complexes have been recognized as ideal small molecules to construct a wide variety of supramolecular nanomaterials. More importantly, controlling the topological structures of biological polymers, such as protein folding/unfolded process, plays a crucial role in a biological system.8,12,13 However, the ability of multiple assembly pathways for the same monomer may lead to the formation of various aggregates with distinct molecular packing, optical properties, and thermodynamic stability, depending closely on the self-assembly conditions, which is a fundamental challenge for researchers.2,14 To obtain the desired self-assemblies, mechanistic insights into the complex pathways and control of the kinetic effects are essential.23 It has been demonstrated that the formation of assembly aggregates can be affected by kinetically trapped or metastable aggregates that compete with thermodynamically stable states.24–27 For example, Würthner et al. have developed a series of fluorescent supramolecular systems of perylene bisimide-based J-aggregates, in which kinetically trapped states are directed by imide hydrogen-bonding interactions.2,28 Recently, Fernández et al. have demonstrated the strategy of N–H⋯Cl–MII interactions in controlling kinetic pathways for the self-assembly of imide-substituted bispyridyldihalogen PtII/PdII complexes.14,29–31 We recently reported two pyrazole-based kinetically trapped BODIPY dyes that exhibited self-assembly behavior and competing pathways to form metastable J-aggregates and thermodynamically stable H-aggregates.32 Nevertheless, well-documented examples of “kinetically trapped” supramolecular polymerization for Pt(II) complexes are scarce. Thus, the development of a single Pt(II) complex that involves more than two different assemblies with distinct optical and morphological properties is highly required.
Our previous studies reported a series of Pt(II) isocyanide complexes bearing amide linkers that exhibited cooperative supramolecular polymerization in non-polar solvents, whereas isodesmic self-assembly for Pt(II) complexes with ester linkers.33 Based on these results, the class of Pt(II) complexes might have the possibility to self-assemble two or even more different assemblies with distinct properties by controlling kinetic effects. To explore this point, herein we have designed two enantiomeric pairs of chiral organoplatinum(II) complexes D/L-Pt-1, and D/L-Pt-2, which bear two different chiral isocyanide ligands (Scheme 1). To minimize the structural variations, two similar chiral camphorone-based isocyanides with considerable steric hindrance were chosen as the auxiliary ligands, with two different linking groups, i.e. amide or methylene sulfonamide. Their supramolecular polymerization behaviors, associated with photophysical changes in various solvents and their other well-defined nanostructures, have been systematically investigated.
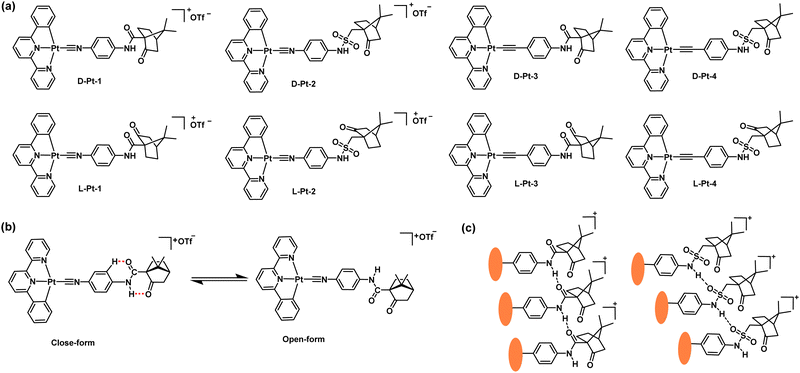 |
| Scheme 1 (a) Molecular structures of platinum(II) complexes D/L-Pt-1–Pt-4; (b) the chemical equilibrium between the open and close conformations; (c) the proposed intermolecular hydrogen bonding packing mode for Pt-1 and Pt-2. | |
The motivations of the molecular design are as follows: (1) the pincer C^N^N ligands and substituted aromatic isocyanide ligands not only can promote the self-assembly process by intermolecular π–π interactions, but also are beneficial for the spectroscopic analysis of the self-assembled process. (2) The type of the linker seems to determine the pathway complexity: for complex Pt-2 with methylene sulfonamide as the linker, intermolecular hydrogen bonding between the carboxyl groups and N–H can efficiently occur for complex Pt-2 in a nonpolar solvent, resulting in thermodynamically controlled assemblies. In contrast, the utilization of an amide group as the linker, together with a carbonyl group on camphorone, allows the formation of an intramolecularly H-bonded pseudocycle for complex Pt-1 (Scheme 1), giving an equilibrium co-existence of the “closed-form” and “open-form” monomeric species in the solution. Initially, the solvophobic interactions together with Pt···Pt and π–π interactions drive the self-assembly of complex Pt-1 into metastable nanotoroids. Over time, steric hindrance effects induce a molecular rearrangement into flexible nanofibers, including nanotwists and nanohelixes, that are stabilized by intermolecular hydrogen bonding of amide and carbonyl groups. The final formation of microbelts indicates the occurrence of highly-ordered self-assembly. In contrast, complex Pt-2 is found to assemble into well-defined nanospheres and microcubes in non-polar solvents. (3) The hydrophobic chiral camphorone groups not only increase the colloidal stability of the aggregates but also help to modulate the configuration of molecular packing via the large steric hindrance. Through changing the linker of the chiral camphorone motif, the transfer of chirality and regioisomerism can be effectively adjusted, which influences their self-assembly capability and resultant nanostructures in mixed solvents. As a result, remarkable amplification of circular dichroism (CD) and CPL signals from small molecules to nanotwists was observed for both pairs of complexes Pt-1 and Pt-2. For comparison, two enantiomeric pairs of neutral Pt(II) complexes Pt-3–Pt-4 with similar structures have been synthesized and investigated.
Results and discussion
Synthesis and characterization
The synthetic routes of the desired ligands L1–L4 and complexes Pt-1–Pt-4 are illustrated in Schemes S1 and S2, and the detailed procedures and characterization data are given in the ESI.† The cationic Pt(II) complexes Pt-1–Pt-2 and neutral complexes Pt-3–Pt-4 were prepared in moderate to high yields by the reaction of the chloroplatinum(II) precursor with the corresponding chiral isocyanide ligands or the chiral acetylene ligands, respectively. All new ligands and complexes were fully confirmed by 1H NMR, 13C NMR, and high-resolution mass spectroscopy (HRMS) as well as Fourier transform infrared (FT-IR), as shown in Fig. S1–S25 (ESI†). Intense absorption bands of ν(N
C) were observed at higher frequency (ca. 2185 and 2188 cm−1) for complexes Pt-1 and Pt-2, comparing to the corresponding acetylene ligands based Pt-3 and Pt-4 at ca. 2100 and 2098 cm−1 for the absorption bands of ν(C
C), respectively, further confirming the successful synthesis of the complexes (Fig. S25, ESI†).
X-Ray crystallography
The successful synthesis of ligands was also confirmed by single-crystal X-ray analyses. Suitable single crystals of ligands L2–L4 were obtained by slow evaporation in ethyl acetate solutions and their crystal structures were determined. The molecular structures of ligands L2–L4 are shown in Fig. S26–S28 (ESI†), and the crystal structure determination data are summarized in Table S1 (ESI†). D-L2 crystallizes in the triclinic P
space group, while L-L3 and L-L4 crystallize in the orthorhombic P212121 space groups (Table S1, ESI†). Crystal structural analysis of ligand L2 revealed that the unit cell contained two independent molecules with distinct conformations (referred to as L2a and L2b, see Fig. S26a, ESI†). For the ligands L2 and L4, the benzene ring is nearly perpendicular to the sulfonamide plane with dihedral angles of 80.48° (L2a), 73.65° (L2b), and 78.6° (L4), indicating the presence of dihedral angles intramolecular N–H⋯O
C hydrogen bonding, together with intramolecular N–H⋯O
C hydrogen bonding (Fig. S27, ESI†). Ligand L2a displays a C
O⋯H–N distance of 2.915 Å, which is quite similar to that of ligand L4 with 2.886 Å. Because of loose intramolecular seven-membered rings hydrogen bonding, the formation of intermolecular hydrogen bonds can be assumed for ligands L2 and L4 (2.232 Å and 2.213 Å for ligand L2, 2.250 Å for ligand L4, Fig. S28, ESI†). Interestingly, the amide torsion angle and dihedral angle between the amide plane and the benzene ring groups of ligand L3 are 4.51° and 4.37°, respectively, which is almost planar in the crystal. Ligand L3 displays a C
O⋯H–N distance of 1.970 Å and a C
O⋯H distance of 2.275 Å, in the formation of two fused six-member rings. Unfortunately, intensive efforts to grow single crystals for all these complexes were unsuccessful. Thus, the geometries of Pt-1 and Pt-2 were optimized in CH3CN using density functional theory (DFT) (Fig. S29, ESI†). In stark contrast to Pt-2, the optimized geometry of complex Pt-1 displayed that the benzene ring, amide group, and carbonyl group on the camphorone motif are almost coplanar, which is consistent with the single crystal structure of L3.
Spectroscopic studies in solution
Before studying the self-assembly behaviors, the photophysical properties of Pt-1–Pt-4 were investigated systematically in solution (Fig. 1 and Fig. S30–S33, ESI†). The simulated UV-vis absorption spectra via TDDFT calculation and the comparison to those of the experimental spectra are provided in Fig. S34 (ESI†). The theoretical spectra could reproduce the experimental spectra features very well.
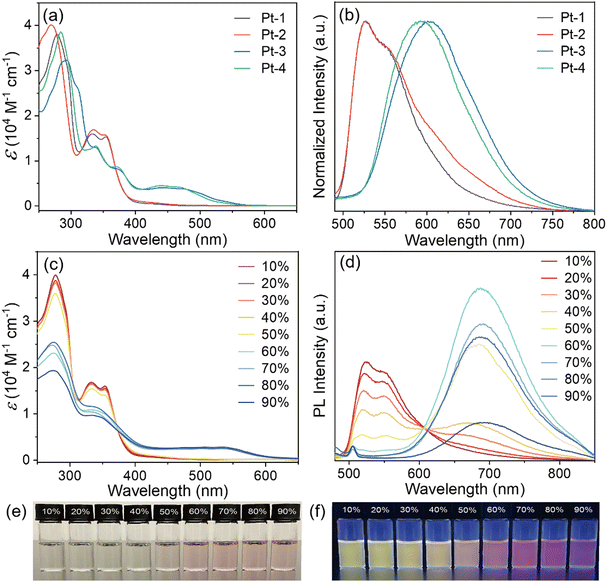 |
| Fig. 1 (a) UV-Vis absorption and (b) normalized PL spectra excited at 420 nm of complexes Pt-1, Pt-2, Pt-3, and Pt-4 in dilute CHCl3; (c) UV-Vis and (d) emission spectra of Pt-1 in CHCl3-MCH with different MCH fractions (vol, 10–90%); and photographs of complex Pt-1 in the CHCl3-MCH mixture excited at 420 nm under visible light (e) and 365 nm UV light (f) irradiation. | |
As shown in Fig. 1a, the absorption spectra of complexes Pt-1–Pt-2 in dilute chloroform (10−5 M) possessed moderate intense absorption bands below ca. 340 nm, and weak absorption bands between 390 and 450 nm. Based on the molar extinction coefficients, the calculated natural transition orbitals (NTO, Tables S2 and S3, ESI†), we attributed the high-energy absorption bands to the intra-ligand (1IL) 1π–π* transitions of the C^N^N ligand and the low-energy absorption bands to 1MLCT/1LLCT of the C^N^N ligand transitions.34
The absorption spectra of Pt-3–Pt-4 in CHCl3 solution show high-energy absorption bands in the range of 290–400 nm, and broad low-energy absorption bands between ca. 410 and 500 nm. The former can be attributed to intraligand charge-transfer (1ILCT) transitions of the (C^N^N) ligand, while the latter can be assigned mainly to 1MLCT transitions.35 This character of transition was further confirmed by NTOs (Tables S2 and S3, ESI†).
Complexes Pt-1 and Pt-2 are soluble in CHCl3, THF, and CH3CN, whereas their solubility in nonpolar solvents such as hexane and methylcyclohexane (MCH) is very poor. Thus, the self-assembly behaviors of these complexes were examined in the mixed solvents of CHCl3 and MCH with different ratios. For complex Pt-1, when the MCH content in CHCl3 reaches ca. 40%, the slightly reduced absorption band intensity indicates the formation of well-dispersed nanoaggregates of Pt-1agg-I. Upon the increase of MCH content to ca. 60%, complex Pt-1 showed a dramatic high-energy absorbance decrease, along with the emergence of a new absorption shoulder at ca. 400–600 nm. On the basis of the other related studies,15,36 the newly formed absorption band should be originating from the metal–metal-to-ligand charge transfer (1MMLCT) transition, indicating the formation of new aggregate species, namely Pt-1agg-II. Thus, it is very promising to construct controllable nanoscale structures with various morphologies for complex Pt-1. Similarly, upon increasing the MCH content in chloroform solutions for Pt-2 (Fig. S35, ESI†), an additional absorption band appears at ca. 566 nm, which is also assigned as the 1MMLCT band. However, the growth of an apparent new absorption band was not observed in the cases of Pt-3 and Pt-4 (Fig. S36 and S37, ESI†).
The emission spectra on the solvent effect of complexes Pt-1–Pt-4 in CHCl3/MCH mixtures were also recorded (Fig. 1d and Fig. S35–S37, ESI†). Complex Pt-1 in CHCl3 exhibited an emission maximum at ca. 528 nm with a quantum yield of 4.0% and a lifetime (τ) of 537 ns (Table S4, ESI†). It is anticipated that the presence of transition metal Pt atoms possessed large spin–orbit coupling constants that can assist in facile intersystem crossing from single excited states to triplet excited states.15 Considering the large Stokes shifts, relatively long lifetimes, and TDDFT calculations, we assigned the emission band for Pt-1 to the triplet excited state 3MLCT/3LLCT character.34 The relatively low emission efficiency may partially be caused by the quenching of molecular oxygen in the solution. Upon increasing the MCH content in the CHCl3/MCH mixture, the emission band at 525 nm decreased gradually, along with the appearance of a new emission band at around 687 nm with a quantum yield of 9.0% and lifetime (τ) of 229 ns, which can be ascribed to 3MMLCT character.15,36 A similar phenomenon has been observed for complex Pt-2 (Fig. S35, ESI†). On the contrary, the emission-quenching phenomena of Pt-3 and Pt-4 in non-polar solvents were observed (Fig. S36-S37, ESI†). Such emission enhancement in high MCH content for complex Pt-1 could be attributed to the formation of aggregates Pt-1agg-II induced by solvophobic interactions and Pt⋯Pt/π–π interactions with the increasing of non-polar solvent.
CD and CPL properties of self-assemblies
To further clarify the chirality transfer and amplification, the circular dichroism (CD) and CPL spectra of complexes Pt-1–Pt-4 were carried out (Fig. 2 and Fig. S38–S41, ESI†). As shown in Fig. 2a, a pair of weak mirror-image CD spectra of complexes D/L-Pt-1 appeared at ca. 300 nm in the diluted chloroform solution (50 μM). The weak Cotton effect at the band of 300 nm revealed that the chirality was transmitted from the camphorone group to the originally achiral pincer C^N^N ligand. Remarkably, a bisignated CD signal with a positive Cotton effect at 283 nm and a negative Cotton effect at 395 nm was observed for D-Pt-1 in the solution of CHCl3/MCH (2/8, v/v, 50 μM), with a CDmax amplified by ca. 70-fold. A similar mirror-image CD spectrum was obtained for L-Pt-1 assemblies, indicating the induction of chirality with opposite handedness from enantiomers. Surprisingly, a CDmax amplified by ca. 150-fold was found for Pt-2 in the solution of CHCl3/MCH (2/8, v/v, 50 μM) compared to the monomeric species. More intriguingly, both Pt-1 and Pt-2 assemblies showed intense bisignated CPL signals with relatively high |glum| values of 0.013 and 0.023, which may benefit from the effective exciton coupling of assemblies at excited states. These observations suggest that the chirality for complexes Pt-1 and Pt-2 is not only transferred from the molecules into the supramolecular polymers but also significantly amplified due to the strong intermolecular π–π interaction and hydrogen bonds. Furthermore, the chirality is amplified from the molecular level to the higher-ordered chiral assemblies through π–π and strong intermolecular hydrogen bond interactions. In sharp contrast, no prominent chirality amplification for complexes Pt-3 and Pt-4 was observed during the supramolecular polymerization process (Fig. S40 and S41, ESI†). Such dramatic spectroscopic behaviors for cationic and neutral complexes may be attributed to their solubility difference in nonpolar solvents and hence assembly performance variations, suggesting the importance of the solvophobic interactions in the self-assembly process.
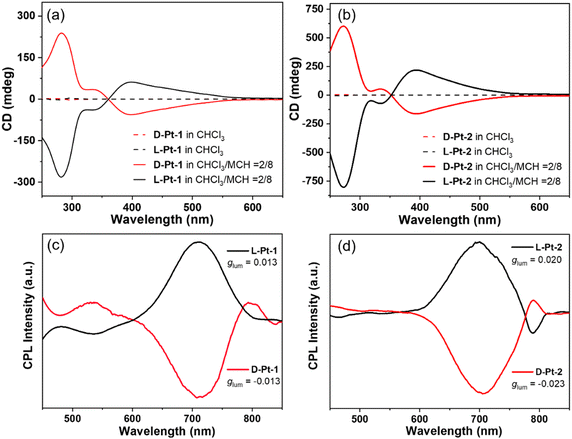 |
| Fig. 2 CD spectra of complexes D/L-Pt-1 (a) and D/L-Pt-2 (b) in CHCl3 and in the MCH-CHCl3 mixture (2/8, v/v); CPL spectra excited at 420 nm of complexes D/L-Pt-1 (c) and D/L-Pt-2 (d) in the MCH-CHCl3 mixture (2/8, v/v) (c = 50 μM). | |
Morphology studies of self-assemblies
To gain deep insight into the self-assembly behaviors of Pt-1–Pt-4, scanning electron microscopy (SEM), transmission electron microscopy (TEM), the aberration-corrected high-angle annular dark-field scanning TEM (HAADF-STEM), and atomic force microscopy (AFM) were further employed for morphology studies. Delightedly, well-defined morphologies for complex Pt-1, including nanotwists, nanorings, dendritic twists, and nanorods were formed (Fig. 3, and Fig. S42–S46, ESI†) through the screening of simple parameters, i.e., temperatures, mixed solvent ratios, and concentrations. In general, when 80% MCH was added to the CHCl3 solution of complex Pt-1, the morphologies of uniform short fibers with lengths of a few hundred nanometers were observed (Fig. S42, ESI†). With the MCH content decreasing to 60%, these molecules formed longer nanofibers with an average ca. 40 nm in width and tended to form bent twisted nanostructures. More interestingly, the SEM images of Pt-1 in 40% MCH in CHCl3 solution showed unique toroidal nanostructures with an average of external diameters ca. 230 nm (Fig. 3a). Smaller toroidal nanostructures were further confirmed from TEM, HAADF-STEM, and AFM images (Fig. 3b–d and Fig. S43, S44, ESI†), which indicated the height of the nanorings to be ca. 8 nm (Fig. S44, ESI†). The HAADF-STEM images and corresponding EDS mapping analyses (Fig. 3c and Fig. S44, S45, ESI†) not only confirmed the self-assemblies but also provided evidence for Pt⋯Pt interactions by measuring the distance between the neighboring Pt atoms.37 The ring closure of the supramolecular polymers requires both the bending and cyclization processes of twisted fibers, which can be facilitated by kinetic control of gentle sonication.
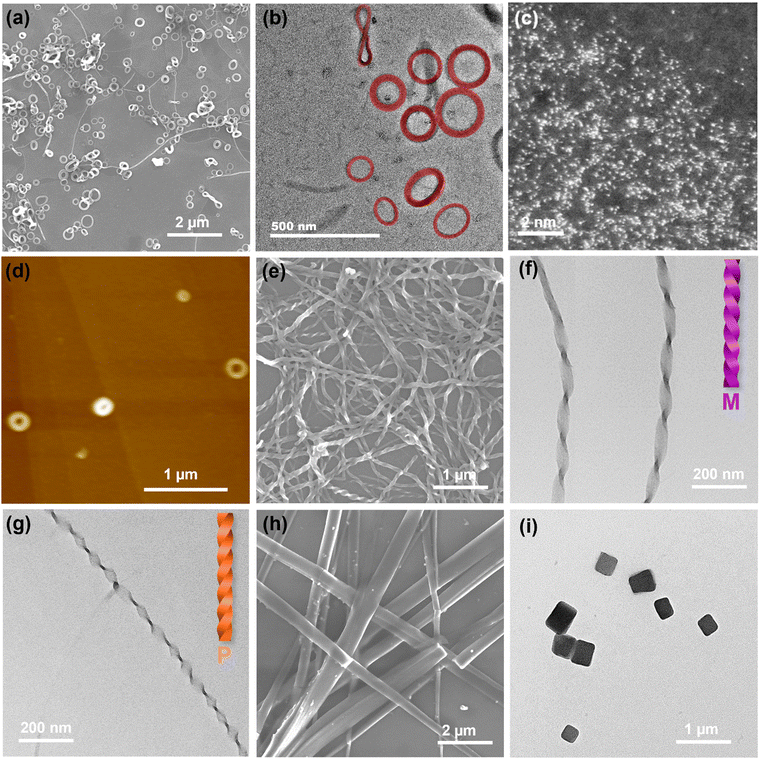 |
| Fig. 3 (a–d) SEM, TEM, HAADF-STEM and AFM images of D-Pt-1 self-assemblies prepared in 40% MCH-CHCl3 solution at R.T. without aging (the nanorings highlighted as red); (e and f) SEM and TEM images of D-Pt-1 self-assemblies prepared in 40% MCH-CHCl3 solution after aging for 2 h at 288 K; (g) TEM image of L-Pt-1 self-assemblies prepared in 40% MCH-CHCl3 solution after aging for 2 h at 288 K; (h) SEM image of D-Pt-1 self-assemblies prepared in 40% MCH-CHCl3 solution after aging for 3 days; (i) TEM images of D-Pt-2 self-assemblies prepared in 40% MCH-CHCl3 solution after aging for 16 h at R.T. | |
When cooling the temperature to 288 K, essentially long nanotwists with an average width of about 90 nm were observed for both complexes D-Pt-1 and L-Pt-1 (Fig. 3e and Fig. S45, ESI†). The supramolecular chirality of the assemblies was also observed using SEM. Interestingly, left-handed (M) twists appeared from the solution of complex D-Pt-1 while the opposite right-handed (P) twists were formed using the L-enantiomer, which is consistent with the results from the CD spectra studies mentioned above. With the prolongation of the self-assembly time to 12 h, the initially formed uniform twist nanostructures subsequently entangled with others to grow into higher-order dendritic twist structures (Fig. S46a–c, ESI†). After 3 days, precipitates were dominated by microbelt structures with lengths of a few hundred micrometers as revealed in the corresponding SEM images (Fig. 3h and Fig. S46f, ESI†). This indicated that upon aging the CHCl3-MCH (60/40, v/v, 50 μM) of complex Pt-1 at 288 K, the initially assembled nanofibers grew gradually into the twists, dendritic twists, and finally microbelts. Overall, we found that the nanotwists or nanoring structures were formed in hours, while the microbelts were generated after days of aging.
In sharp contrast, complex Pt-2 linked with the sulfamide group showed different aggregated species under similar conditions. Nanospheres with diameters of ca. 100 nm have been observed in a CHCl3-MCH (60/40, v/v, 50 μM) mixture (Fig. S47, ESI†). Prolonged standing time results in forming cubic nanostructures (Fig. 3i and Fig. S48, ESI†), which can be promoted by reducing the content of MCH to 20% (Fig. S47, ESI†). Such differences in the self-assembly behaviour and morphologies of complex Pt-2 can be attributed to a different packing conformation caused by the slight structural modification, suggesting the significance of the “kinetically trapped” design in Pt-1. Whereas for Pt-3 and Pt-4, only monodispersed nanospheres were observed (Fig. S49 and S50, ESI†).
Structural elucidation of the assemblies
To further understand the self-assembly process, variable-temperature UV-vis absorption was performed in the mixed solvent of 1,1,2,2-tetrachloroethane (TCE), and MCH (3
:
7, v/v, TCE was employed as a higher boiling point than CHCl3) at a concentration of 20 μM. As shown in Fig. 4a, upon slow heating (1 K min−1) of the mixture of Pt-1agg-II from 298 K to 363 K, the absorption bands at ca. 280 nm and 335 nm gradually increased, along with the disappearance of the shoulder at ca. 540 nm. The UV-vis profile of complex Pt-1 at 363 K is very similar to that in pure CHCl3, suggesting predominant molecular monomeric species at high temperatures, indicating the depolymerization of aggregates Pt-1agg-II of complex Pt-1 (Fig. 4a). By plotting the degree of aggregation (αagg)38 against temperature from UV-vis absorption at 290 nm, a non-sigmoidal heating curve with a very high elongation temperature (ca. 358 K) can be obtained, which evidenced that the formation of Pt-1agg-II proceeds via a cooperative nucleation-elongation mechanism.38–40 The negative enthalpy polymerization process (Table S5, ESI†) is consistent with aggregation arising from the involvement of intermolecular Pt⋯Pt/π–π interactions and multiple hydrogen bonds as discussed above. Nevertheless, the current variable-temperature data fitting model is not suitable for understanding the complex assembly process of this complex quite well. To better understand the assembly process of this complex, a more detailed variable-temperature data fitting model is needed. However, Pt-1agg-II will transform into more thermodynamically stable micro-belt aggregates at high temperatures.
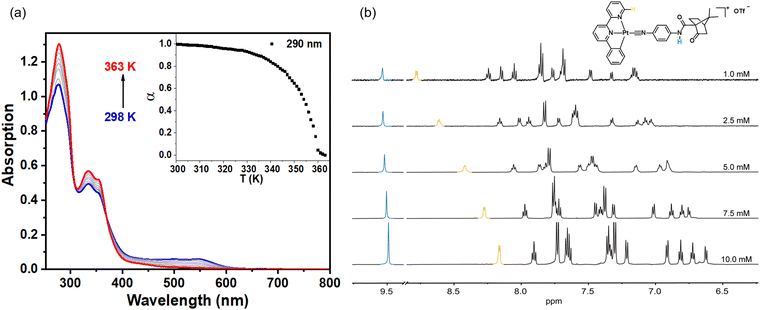 |
| Fig. 4 (a) Temperature-dependent UV-Vis absorption spectra of complex Pt-1 in a mixture of TCE/MCH (v/v, 3/7, c = 50 μM) upon heating from 298 K (red line) to 363 K (blue line) with a rate of 1 K min−1 showing from the transformation from Pt-1agg-II to Pt-1mono. Inset: Degree of Pt-1agg-II (αagg) monitored at 290 nm vs. temperature. (b) Concentration-dependent 1H NMR spectra of Pt-1 in CD3CN at 298 K. | |
In order to gain insights into the interaction patterns dictating during the supramolecular polymerization, concentration-dependent and temperature-dependent 1H NMR experiments of Pt-1–Pt-4 were carried out (Fig. 4b and Fig. S51–S55, ESI†). Increasing the concentration results in pronounced up-field shifts for the protons of complex Pt-1 in the aromatic region, indicative of the strong shielding effect induced by the formation of aggregates through intermolecular π–π/Pt⋯Pt interactions. Simultaneously, only slight shifts for the amide N–H proton were observed from 9.53–9.49 ppm. Meanwhile, the variable-temperature 1H NMR spectra of Pt-1 in CD3CN showed a very similar phenomenon upon cooling the temperature from 343 K to 283 K (Fig. S51, ESI†). A plausible explanation would be the formation of the closed-form monomeric species caused by the highly stable intramolecular hydrogen bonding between the amide N–H group and the camphorone carbonyl group (Scheme 1). Notably, the open-form monomeric species was not visible even at high temperatures, most likely due to the very strong strength of intramolecular hydrogen bonding, which is consistent with the formation of two fused six-membered rings as mentioned above.
Upon increasing the concentration for Pt-2, the aromatic proton signals also undergo apparent up-field shifts, whereas the amide N–H signal was found to down-field shift (Fig. S52, ESI†). A similar phenomenon was observed for complex Pt-2 in the temperature-dependent 1H NMR experiments (Fig. S53, ESI†). These observations indicate the formation of intermolecular hydrogen bonds from the monomeric species for Pt-2. On the basis of the concentration-dependent 1H NMR spectra, Pt-3 and Pt-4 were found to exhibit similar variation tendency but much fewer changes than those of Pt-1 and Pt-2, indicating negligible intermolecular π–π/Pt⋯Pt interactions for Pt-3 and Pt-4. It is worth noting that the protons ascribable to camphorone rings experience almost no shift for all these complexes, which indicates no aggregation formation for camphorone rings. Taking all the above spectroscopic evidence into account, it is possible to shed some light on the aggregation mode of all these complexes, particularly the potential role of the linker units to participate in competing intra- vs. intermolecular hydrogen bonding and π–π/Pt⋯Pt interactions during the process of self-assembly. Among them, we infer that the equilibrium between intramolecular hydrogen bonding (closed conformation) and non-hydrogen bonding states (extended conformation) of complex Pt-1 affords the expected kinetically trapped assembling system.
Proposed self-assembly pathway
On the basis of the photophysical data, electronic microscopic studies as well as NMR data, a proposed polymorphic assembly pathway for Pt-1 is suggested as depicted in Fig. 5. The chiral camphorone moieties are considered to have higher solubility towards non-polar solvent, whereas the platinum cores tightly pack in the inner core of the assembly. Upon adding a small fraction of MCH (20–40%) to the CHCl3 solution of Pt-1, the positively charged tridentate cyclometalated platinum(II) isocyanide moiety became less solvated, indicating that the solvophobic interactions serve as the predominant driving force that would bring the Pt(II) moieties into close proximity for the formation of weak π–π/Pt⋯Pt interactions. Furthermore, the supramolecular Pt⋯Pt chains are perturbed by the electrostatic repulsion between two neighboring Pt(II) cations, which subsequently induces the distortion and undergoes self-assembly into toroidal nanostructures Pt-1agg-I, appended with the “close-form” amide substituted camphorone as the exterior. In such mixture compositions, no MMLCT band can be observed in the UV-vis spectra, although new 3MMLCT emission bands can be observed upon increasing the MCH content, indicating the formation of well-dispersed oligomers in the solution. With the increasing MCH content to ca. 60%, complex Pt-1 tends to form nanotwist structures Pt-1agg-II with Pt⋯Pt/π–π and intermolecular hydrogen bonding interactions induced by stronger solvophobic interactions, evidenced from the MMLCT absorption shoulders, the 3MMLCT emission bands, and the HAADF-STEM images. Upon hours of aging, the nanotwists tended to grow into higher-order structures (Fig. S46, ESI†), i.e., dendritic twists. With prolonged aging time to days, such formation of solvophobic interactions together with strong intermolecular hydrogen bonding and π–π/Pt⋯Pt interactions would restrict the assemblies in alignment, resulting in linear growth and formation of thermodynamically stable microbelts with orderly and tightly arrangement finally (Fig. 5), which is further confirmed by the PXRD data (Fig. S56, ESI†). Due to the energy barrier for the formation, the transformation from nanotwists Pt-1agg-II to microbelts Pt-1agg-III is relatively slow without any perturbation (several days). However, this transformation can be facilitated by heating the Pt-1agg-II solution to 313 K, thus, the appearance of microbelts is significantly shortened. The reason can be rationalized that heating the solution could accelerate the transformation of intramolecular hydrogen bonds to intermolecular ones, which provides additional driving forces for molecular self-assembly. Once the nuclei are formed, they can act as seeds to promote the transformation of metastable Pt-1agg-II into thermodynamically stable Pt-1agg-III.
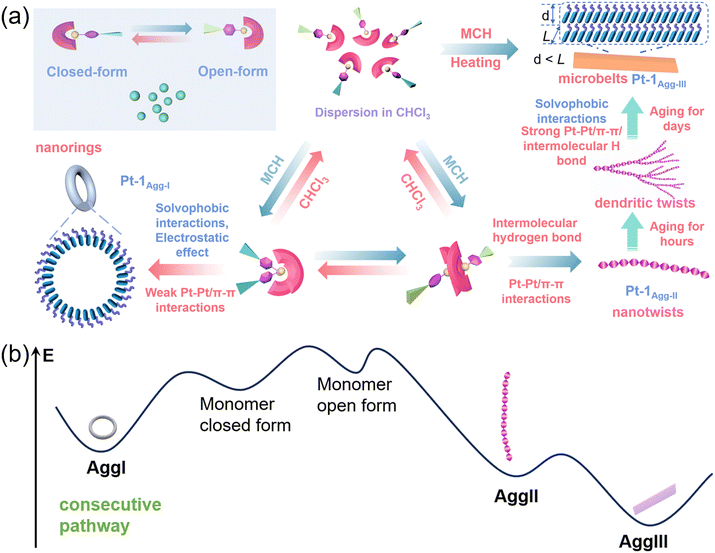 |
| Fig. 5 (a) Cartoon representation of the supramolecular polymerization pathways for Pt-1. (b) The energy landscape of the consecutive supramolecular polymerization for Pt-1. | |
On the other side, molecules of complex Pt-2 would assemble into nanospheres with the transition metal center Pt part as the core while loosely packing camphorone motif as a shell, due to the steric hindrance of the bridgehead methyl moiety in methylene sulfonamide substituted camphorone. With the alignment of the metallophilic interactions, the complex would gradually transform from nanospheres into nanocubes upon the prolongation of standing time.
The distinct mechanisms during the self-assembly of amide-bearing Pt-1 and ester-bearing Pt-2 should probably lie in the modulation of the hydrogen bonding interaction, which leads to different organizations of the complex. Moreover, the formation of two six-member rings via intramolecular hydrogen bonds in complex Pt-1 increased the rigidity of the isocyanide ligand, accordingly resulting in closer packing. Whereas Pt-3 and Pt-4 are less tightly assembled into nanospheres to reduce the surface energy.
Conclusions
In summary, four pairs of chiral camphorone-functionalized enantiomeric platinum complexes D/L-Pt-1–Pt-4 are reported, and they have shown the ability to form a variety of distinct morphologies. Such a self-assembly process is governed by the Pt⋯Pt/π–π stacking and the solvophobic interactions as well as electrostatic repulsion provided by the positively charged tridentate cyclometalated platinum(II) moieties. The polymorphic self-assembly with well-defined nanostructures, i.e. nanorings, nanotwists, dendritic twists, and microbelts in various conditions, accompanied by dramatic spectroscopic changes, were systematically investigated by photophysical studies, and electron microscopy. Two pairs of enantiomers D/L-Pt-1–Pt-2 showed significantly amplified effects for chirality and circularly polarized luminescence in their corresponding nanoassemblies. The current work demonstrates that the incorporation of chiral auxiliary ligands into Pt(II) complexes would make them desirable for the creation of various functional supramolecular materials with outstanding chirality and circularly polarized luminescent properties.
Experimental section
All chemical reagents were purchased from commercial sources (Aldrich, Alfa Aesar, Energy Chemical, and Adamas) and were used as received unless otherwise noted. 1H NMR and 13C NMR spectra were recorded on a 600 MHz BRUKER spectrometer at room temperature using tetramethylsilane (TMS) as the internal standard. High-resolution mass spectrometry (HRMS) data were collected on a Bruker maxis UHR-TOF mass spectrometer in the ESI-positive mode. Fourier transform infrared (FT-IR) measurements were performed using a Bruker Tensor27 spectrophotometer. Powder X-ray diffraction (PXRD) patterns were recorded on a Bruker D8 Advance instrument. X-ray single crystal diffraction data were collected on a Nonius Kappa CCD diffractometer using Mo Kα radiation (λ) 0.71073 Å (graphite monochromator). UV-vis spectra were recorded on an Agilent Technologies Cary 3500 spectrometer. Emission spectra and the absolute quantum yields were recorded on a Horiba FluoroMax Plus spectrofluorometer. CD experiments were performed using a Chirascan CD spectrometer. PL lifetimes were measured using an FLS1000 fluorescence spectrometer (Edinburgh) at room temperature. CPL spectra and subsequently derived glum values were recorded using an OLIS CPL Solo spectrofluorometer and the globalworks software suite. Scanning electron microscopy (SEM) was performed using a Hitachi SU8220 instrument. Transmission electron microscopy (TEM) was performed using a Hitachi TEM HT 7800 instrument operating at 80
kV acceleration voltage. The high-angle annular dark-field (HAADF) imaging and EDX images were obtained using an FEI Titan cubed Themis G2 300 microscope operated at 300
kV and equipped with a probe aberration corrector and a monochromator. The samples were dropped onto a carbon-coated copper grid and dried. Atomic force microscopy (AFM) experiments were performed on an Asylum Research Cypher VRS (Oxford instruments) atomic force microscope equipped with a Scan Asyst-HR fast scanning module and a Scan Asyst Air-HR probe (tip radius, 2 nm), utilizing peak force feedback control. Density functional theory (DFT) and TDDFT calculations of these complexes were performed using Gaussian 09 software using the B3LYP, and the LANL2DZ basis set was assigned for the Pt atom and 6-31G* basis set for all the remaining atoms. Multiwfn software was used for NTO analyses.41 Detailed synthesis and experimental procedures are described in the ESI.†
Author contributions
X. Zhu: investigation, conceptualization, data curation, methodology, visualization, writing – original draft, review, and editing, funding acquisition. Z. Wang: investigation, visualization, writing – original draft. Y. Jia: investigation. F. Yang: methodology. Y. Zhang: methodology. Shirui Zhao: investigation. X. He: supervision, conceptualization, funding acquisition.
Conflicts of interest
There are no conflicts to declare.
Acknowledgements
This study was supported by the National Natural Science Foundation of China (22202128), Fundamental Research Funds for the Central Universities (GK202205016, GK202201006, and 2021CSLY010), China Postdoctoral Science Foundation (No. 2020M683417), and the Natural Science Basic Research Plan in Shaanxi Province of China (2021JQ-302).
Notes and references
- S. S. Babu, V. K. Praveen and A. Ajayaghosh, Chem. Rev., 2014, 114, 1973–2129 CrossRef CAS PubMed.
- F. Wurthner, Acc. Chem. Res., 2016, 49, 868–876 CrossRef PubMed.
- J. D. Tovar, Acc. Chem. Res., 2013, 46, 1527–1537 CrossRef CAS PubMed.
- D. B. Amabilino, D. K. Smith and J. W. Steed, Chem. Soc. Rev., 2017, 46, 2404–2420 RSC.
- J. Li, J. Wang, H. Li, N. Song, D. Wang and B. Z. Tang, Chem. Soc. Rev., 2020, 49, 1144–1172 RSC.
- L. Zhang, H. X. Wang, S. Li and M. Liu, Chem. Soc. Rev., 2020, 49, 9095–9120 RSC.
- S. Yagai, Y. Kitamoto, S. Datta and B. Adhikari, Acc. Chem. Res., 2019, 52, 1325–1335 CrossRef CAS PubMed.
- P. K. Hashim, J. Bergueiro, E. W. Meijer and T. Aida, Prog. Polym. Sci., 2020, 105, 101250 CrossRef CAS.
- P. A. Korevaar, S. J. George, A. J. Markvoort, M. M. Smulders, P. A. Hilbers, A. P. Schenning, T. F. De Greef and E. W. Meijer, Nature, 2012, 481, 492–496 CrossRef CAS PubMed.
- A. Sorrenti, J. Leira-Iglesias, A. J. Markvoort, T. F. A. de Greef and T. M. Hermans, Chem. Soc. Rev., 2017, 46, 5476–5490 RSC.
- C. Fouquey, J.-M. Lehn and A.-M. Levelut, Adv. Mater., 1990, 2, 254–257 CrossRef CAS.
- S. W. Englander and L. Mayne, Proc. Natl. Acad. Sci. U. S. A., 2014, 111, 15873–15880 CrossRef CAS PubMed.
- Y. Tezuka, Acc. Chem. Res., 2017, 50, 2661–2672 CrossRef CAS PubMed.
- N. Baumer, J. Matern and G. Fernandez, Chem. Sci., 2021, 12, 12248–12265 RSC.
- V. W. Yam, V. K. Au and S. Y. Leung, Chem. Rev., 2015, 115, 7589–7728 CrossRef CAS PubMed.
- M. C. Yeung and V. W. Yam, Chem. Soc. Rev., 2015, 44, 4192–4202 RSC.
- Z. Gao, Y. Han, Z. Gao and F. Wang, Acc. Chem. Res., 2018, 51, 2719–2729 CrossRef CAS PubMed.
- A. Aliprandi, D. Genovese, M. Mauro and L. De Cola, Chem. Lett., 2015, 44, 1152–1169 CrossRef CAS.
- Y. Chi and P. T. Chou, Chem. Soc. Rev., 2010, 39, 638–655 RSC.
- J. Herberger and R. F. Winter, Coord. Chem. Rev., 2019, 400, 213048 CrossRef.
- R. Chakrabarty, P. S. Mukherjee and P. J. Stang, Chem. Rev., 2011, 111, 6810–6918 CrossRef CAS PubMed.
- V. W.-W. Yam, A. K.-W. Chan and E. Y.-H. Hong, Nat. Rev. Chem., 2020, 4, 528–541 CrossRef CAS.
- M. Wehner and F. Würthner, Nat. Rev. Chem., 2019, 4, 38–53 CrossRef.
- J. Matern, Y. Dorca, L. Sanchez and G. Fernandez, Angew. Chem., Int. Ed., 2019, 58, 16730–16740 CrossRef CAS PubMed.
- X. Wang, G. Guerin, H. Wang, Y. Wang, I. Manners and M. A. Winnik, Science, 2007, 317, 644–647 CrossRef CAS PubMed.
- J. S. Valera, R. Gomez and L. Sanchez, Angew. Chem., Int. Ed., 2019, 58, 510–514 CrossRef CAS PubMed.
- H. Chen, Z. Huang, H. Wu, J. F. Xu and X. Zhang, Angew. Chem., Int. Ed., 2017, 56, 16575–16578 CrossRef CAS PubMed.
- T. E. Kaiser, H. Wang, V. Stepanenko and F. Wurthner, Angew. Chem., Int. Ed., 2007, 46, 5541–5544 CrossRef CAS PubMed.
- M. J. Mayoral, C. Rest, V. Stepanenko, J. Schellheimer, R. Q. Albuquerque and G. Fernandez, J. Am. Chem. Soc., 2013, 135, 2148–2151 CrossRef CAS PubMed.
- A. Langenstroer, K. K. Kartha, Y. Dorca, J. Droste, V. Stepanenko, R. Q. Albuquerque, M. R. Hansen, L. Sanchez and G. Fernandez, J. Am. Chem. Soc., 2019, 141, 5192–5200 CrossRef CAS PubMed.
- J. Matern, K. K. Kartha, L. Sanchez and G. Fernandez, Chem. Sci., 2020, 11, 6780–6788 RSC.
- Y. Zhang, N. Zhou, X. Zhu and X. He, Polym. Chem., 2021, 12, 5535–5541 RSC.
- L. Li, N. Zhou, H. Kong and X. He, Polym. Chem., 2019, 10, 5465–5472 RSC.
- S.-W. Lai, H.-W. Lam, W. Lu, K.-K. Cheung and C.-M. Che, Organometallics, 2002, 21, 226–234 CrossRef CAS.
- W. Liu, B.-X. Mi, M. C. W. Chan, Z. Hui, C.-M. Che, N. Zhu and S.-T. Lee, J. Am. Chem. Soc., 2004, 126, 4958–4971 CrossRef PubMed.
- K. M.-C. Wong and V. W.-W. Yam, Acc. Chem. Res., 2011, 44, 424–434 CrossRef CAS PubMed.
- HAADF-STEM images provide direct and valuable information such as atomic distance, and relative height differences between adjacent Pt atoms according to the different brightness of various atoms. For more information, see ref: J. Shan, C. Ye, Y. Jiang, M. Jaroniec, Y. Zheng and S.-Z. Qiao, Sci. Adv., 2022, 8, eabo0762 CrossRef CAS PubMed.
- M. M. J. Smulders, M. M. L. Nieuwenhuizen, T. F. A. de Greef, P. van der Schoot, A. P. H. Schenning and E. W. Meijer, Chem. – Eur. J., 2010, 16, 362–367 CrossRef CAS PubMed.
- D. Zhao and J. S. Moore, Org. Biomol. Chem., 2003, 1, 3471–3491 RSC.
- T. F. A. De Greef, M. M. J. Smulders, M. Wolffs, A. P. H. Schenning, R. P. Sijbesma and E. W. Meijer, Chem. Rev., 2009, 109, 5687–5754 CrossRef CAS PubMed.
- T. Lu and F. Chen, J. Comput. Chem., 2012, 33, 580–592 CrossRef CAS PubMed.
Footnotes |
† Electronic supplementary information (ESI) available: Detailed experimental procedures; crystals data for ligands; detailed structural characterization data, crystal data, DFT calculations, and photophysical studies. CCDC 2209733, 2209735 and 2209736. For ESI and crystallographic data in CIF or other electronic format see DOI: https://doi.org/10.1039/d3tc02375c |
‡ These authors contributed equally. |
|
This journal is © The Royal Society of Chemistry 2023 |