DOI:
10.1039/D3TC02243A
(Paper)
J. Mater. Chem. C, 2023,
11, 11905-11911
Dithiene-fused quinoxalineimide-based all-acceptor polymers for n-type organic semiconductors†
Received
27th June 2023
, Accepted 5th July 2023
First published on 18th August 2023
Abstract
Introduction of strong electron-deficient building blocks into the polymer backbone is an efficient strategy to design n-type organic semiconductors. In this work, a strong electron-deficient building block, dithiene-fused quinoxalineimide (DTQI), containing electron-withdrawing quinoxaline and imide units, was synthesized and incorporated into a polymer backbone. The frontier molecular orbital (FMO) energy levels downshifted with the incorporation of the quinoxalineimide (QI) unit onto the framework. Owing to the high electron-affinity of DTQI, the DTQI-based acceptor–acceptor (all-acceptor, A–A) type polymer P(DTQI-BTI) showed deep-lying highest occupied molecular orbital (HOMO) and lowest unoccupied molecular orbital (LUMO) energy levels, thus yielding unipolar n-type transport character with electron mobility of 0.25 cm2 V−1 s−1 in organic thin-film transistors. These results demonstrate that DTQI is a promising building block for constructing n-type polymer semiconductors and can be extended to the design of other novel electron-deficient building blocks.
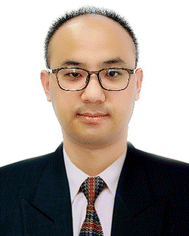
Yongqiang Shi
| Yongqiang Shi is a Professor at School of Chemistry and Materials Science, Anhui Normal University. Before that, he carried out research as a research assistant at Southern University of Science and Technology in 2015–2020 under the supervision of Prof. Xugang Guo. He received his PhD degree from Southwest Petroleum University in 2020. He worked as a “Hong Kong Scholars Program” postdoctoral fellow in the group of Prof. Qichun Zhang at City University of Hong Kong. His research focuses on the design and synthesis of n-type polymers for organic thin-film transistors, polymer solar cells, perovskite solar cells, and organic thermoelectrics. |
Introduction
n-Type organic semiconductors are an excellent important class of active materials in organic optoelectronic devices, such as organic thin-film transistors (OTFTs),1–13 organic photovoltaics (OPVs),14–18 perovskite solar cells (PVSCs),19–21 and organic thermoelectrics (OTEs).22–29 In the past few decades, tremendous efforts have been made to develop p-type (hole transport) and ambipolar (both hole and electron transport) semiconducting polymers.30–34 However, the development of n-type (electron transport) polymer semiconductors lags behind their p-type counterparts due to the limited electron-deficient building blocks.35–37 To realize practical organic electron devices, both n-type and p-type organic semiconductors are required. Therefore, it is necessary and urgent to design and synthesize n-type polymer semiconductors.
To realize unipolar n-type electron transport in polymer semiconductors, both the highest occupied molecular orbital (HOMO) and lowest unoccupied molecular orbital (LUMO) energy levels should be sufficiently low. One of the efficient strategies to design n-type polymer semiconductors is to introduce strong electron-deficient building blocks into the backbone, such as imide,38–42 amide,4,43–46 cyano,47 or B ← N groups.48–52 Among them, imide functionalization has become one of the widely investigated acceptor building blocks for constructing n-type organic semiconductors, which exhibits intriguing optoelectronic properties due to the strong electron-withdrawing character of the imide group and good solubility enabled by the N-alkyl side chain. As typical representatives of imide building blocks, naphthalene diimide (NDI),1,53 perylene diimide (PDI),8,54,55 naphthodithiophene diimide (NDTI),56,57 dithienophtalimide (DTP),58,59 and bithiophene imide (BTI),5 have been widely incorporated into n-type polymer semiconductors. NDI and PDI containing diimide groups are beneficial to realizing the low-lying LUMO energy levels. However, the geometries of NDI and PDI result in sizable steric hindrance with neighboring units and lead to a distorted polymer backbone, which limits electron transport. Recently, BTI (Fig. 1) has shown remarkable success in developing high-performance n-type polymer semiconductors and the BTI-based A–A type polymers have yielded promising device performance in OTFTs, PVSCs, and OTEs.19,23,35
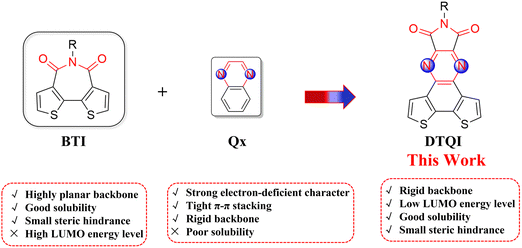 |
| Fig. 1 Molecular design strategy to develop electron-deficient building block DTQI, which combines the advantages of both BTI and Qx. | |
Among various acceptor units, a pyrazine-fused electron-deficient unit is also a promising building block to construct n-type polymer semiconductors due to its strong electron-withdrawing ability, easy functionalization, and tunable LUMO levels.60,61 For example, pyrazine derivatives, such as quinoxaline (Qx) (Fig. 1), fluorinated quinoxaline, thiadiazoloquinoxaline, and dithienobenzoquinoxaline, have been widely developed as high mobility semiconductors for OTFTs.62,63 By proper modification of pyrazine-based acceptor units, the energy levels and charge carrier mobility can be easily tuned by the following methods: (i) changing the quinoxaline-based polymer backbone from acceptor–donor to acceptor–acceptor; (ii) introduction of different side chains to tune the solubility, aggregation behavior and intermolecular interactions of the molecule, which in turn affects the device performance of the polymer; (iii) introduction of functional groups onto the quinoxaline backbones, such as F atoms, Cl atoms, and cyano and ester groups, to lower the LUMO energy levels of the polymers. Therefore, it is highly desired to design other novel pyrazine-based acceptor units and investigate their structure–property relationship.
Inspired by the excellent performance of both BTI- and pyrazine-based organic semiconductors, herein, a dithiene fused quinoxalineimide (DTQI) (Fig. 1) electron-deficient building block, containing electron-withdrawing quinoxaline and imide units, was synthesized and incorporated into polymer semiconductors. DTQI-based A–A type polymers PDTQI and P(DTQI-BTI) exhibit a highly planar backbone and low LUMO energy levels. The low-lying LUMOs (<−3.50 eV) of the polymers could effectively facilitate electron injection in OTFTs. P(DTQI-BTI) showed a high μe of >0.25 cm2 V−1 s−1. The results demonstrate that dithiene-fused quinoxalineimide is a promising electron-deficient building block for constructing n-type polymer semiconductors.
Results and discussion
Materials synthesis and characterization
The synthetic route to monomer DTQI and its corresponding A–A type polymers PDTQI and P(DTQI-BTI) is depicted in Scheme 1, and the detailed synthetic procedures are provided in the ESI.† Compound 1 was synthesized according to the reported procedure.64,65 Key intermediate compound 2 was obtained via the condensation of 1 with diethyl 2,3-dioxosuccinate. 2 was then dibrominated with NBS to yield 3. Carboxylic acid compound 4 was obtained by hydrolysis with sodium hydroxide and successive hydrochloric acid treatment, and then 4 was treated with acetyl chloride to yield acid anhydride 5. The imide molecule DTQI-2Br was successfully obtained by amidation of compound 5 with the corresponding alkylamine and successive imidization by using acetic anhydride. The monomer and key intermediates were fully characterized by 1H NMR and 13C NMR (Fig. S1–S8, ESI†).
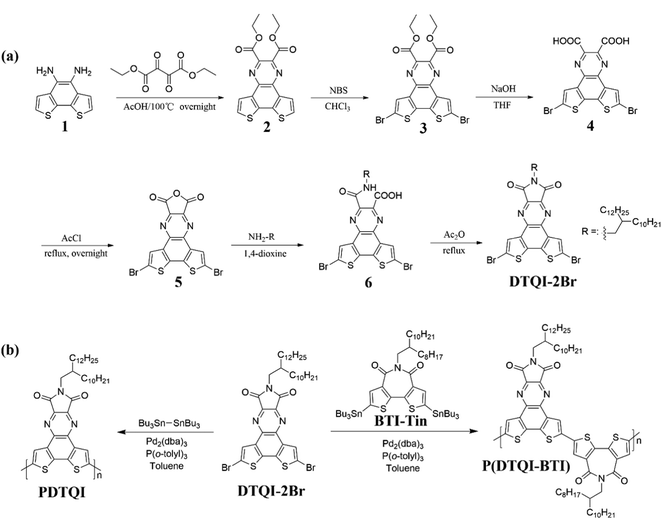 |
| Scheme 1 Synthetic route to (a) the dibrominated dithiene-fused quinoxalineimide monomer DTQI-2Br and (b) DTQI-based A–A type polymers PDTQI and P(DTQI-BTI). | |
The polymerization of DTQI-2Br was carried out with comonomers bis(tributyltin) and distannylated BTI (BTI-Tin) via the Stille coupling reaction using Pd2(dba)3/P(o-tol)3 as the catalyst system to afford A–A type polymers PDTQI and P(DTQI-BTI), respectively (Scheme 1b). BTI-Tin was chosen as the comonomer due to its high planar backbone, good solubility and high reactivity.12 Additionally, incorporation of BTI into the polymer backbone would increase the content of acceptor unit and allow us to tune the frontier molecular orbital levels of the conjugated polymers. After polymerization, the polymers were purified by successive Soxhlet extractions with methanol, acetone, hexane, dichloromethane and chloroform. The final chloroform fractions were collected and reprecipitated into methanol to afford the A–A type polymers, which were used for characterization and device fabrication. The number-average molecular weights (Mns) of the polymers were measured by high-temperature gel permeation chromatography (HT-GPC) with 1,2,4-trichlorobenzene as the eluent at 150 °C. As summarized in Table 1, PDTQI has an Mn of 54 kDa with a polydispersity index (PDI) of 1.8, and P(DTQI-BTI) shows an Mn of 65 kDa with a PDI of 1.7.
Table 1 Molecular weights, and optical and electrochemical properties of the polymers
Polymer |
M
n
(KDa) |
PDI |
λ
filmmax b (nm) |
λ
filmonset (nm) |
E
optg c (eV) |
E
LUMO
(eV) |
E
HOMO
(eV) |
GPC versus polystyrene standard, trichlorobenzene as the eluent at 150 °C.
Absorption of the as-cast film in chloroform solution.
Estimated from the absorption onset of the as-cast polymer film using the equation: Eoptg = 1240/λonset (eV).
E
LUMO = −e(Eonsetred + 4.80) eV, Eonsetred determined using the Fc/Fc+ external standard.
E
HOMO = ELUMO -− Eoptg.
|
PDTQI |
54 |
1.8 |
345 |
627 |
1.98 |
−3.60 |
−5.58 |
P(DTQI-BTI) |
65 |
1.7 |
586 |
627 |
1.98 |
−3.63 |
−5.61 |
Polymer thermal properties
The thermal properties of the two A–A type polymers were investigated by thermogravimetric analysis (TGA) and differential scanning calorimetry (DSC). As shown in Fig. S9 (ESI†), both polymers exhibited excellent thermal stability with the decomposition temperature (5% weight loss) over 400 °C. DSC shows clearly distinct thermal properties of PDTQI, and a glass transition temperature (Tg) of 153 °C was detected during the heating scan. The exothermic peak at ≈250 °C corresponded to the phase transition temperature of PDTQI, while for P(DTQI-BTI), no endothermic or exothermic peak was observed during the heating or cooling process, revealing that no phase transitions were observed within the temperature range (25–350 °C).
Theoretical calculations
To evaluate the electronic structure and optimized geometry of DTQI, density functional theory (DFT) calculations were performed at the B3LYP/6-31G(d) level using the Gaussian 09 program. The optimized molecular geometry of DTQI is a completely planar structure (Fig. S10, ESI†). Compared with BTI, the new DTQI shows a low-lying FMO energy level, which should facilitate electron injection and block hole accumulation, thus resulting in n-type transport character. In comparison with BTzI,33 fusing a bithiophene unit into the quinoxalineimide core can improve the reaction activity between the DTQI monomer and other acceptor comonomers, thus obtaining a polymer with high Mn. Therefore, a DTQI building block is favorable for constructing high-performance n-type polymer semiconductors. The π-electron density distributions of the LUMO levels are widely delocalized over the π-framework, while the HOMO level is mostly localized around the central phenyl ring and thiophene rings of the DTQI core (Fig. 2). The calculated HOMO and LUMO levels are −6.21 and −2.60 eV for DTQI. The LUMO level of the DTQI is 0.3 eV lower than the calculated LUMO level of the BTI. The relatively lower LUMO level of the DTQI is beneficial for the electron transport. These results confirm that integrating electron-deficient BTI and quinoxaline units into a new building block is an effective strategy for achieving polymer semiconductors with high planar backbones and deep-lying LUMO levels.
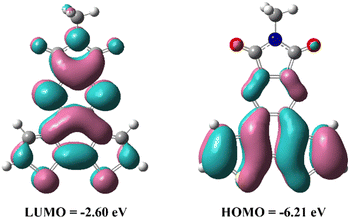 |
| Fig. 2 Frontier molecular orbital energy levels of DTQI. | |
Optical and electrochemical properties
The optical and electrochemical properties of DTQI-based polymers have been evaluated by UV-via absorption spectra and cyclic voltammetry (CV), and the corresponding data are summarized in Table 1. As shown in Fig. 3a, homopolymer PDTQI showed nearly the same absorption spectra in both film and solution. However, P(DTQI-BTI) exhibited two main peaks at 500–600 nm (Fig. 3b), and the long wavelength absorption band of P(DTQI-BTI) is ascribed to the intramolecular charge transfer (ICT). By incorporating a BTI acceptor unit into the P(DTQI-BTI) backbone, the maximum absorption peak of P(DTQI-BTI) is larger than that of PDTQI, suggesting the stronger π–π intermolecular aggregation of P(DTQI-BTI). The optical band gaps (Eoptgs) of PDTQI and P(DTQI-BTI) are 1.98 eV, determined from the polymer film absorption onsets.
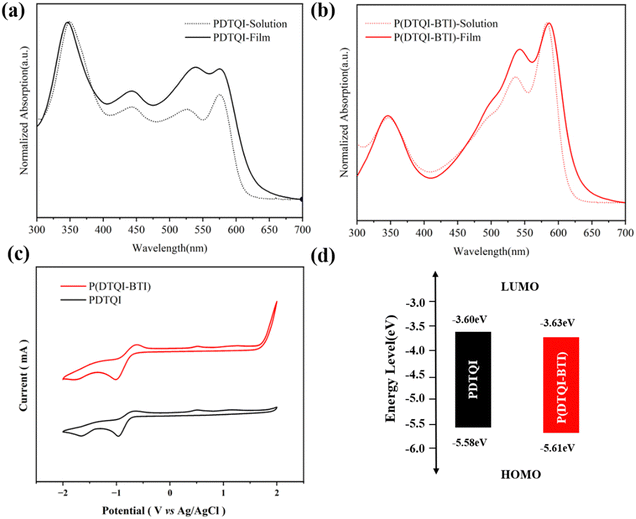 |
| Fig. 3 Normalized UV-vis absorption spectra of the DTQI-based polymers (a) PDTQI and (b) P(DTQI-BTI). (c) Cyclic voltammograms of polymer thin films measured in 0.1 M tetrabutylammonium hexafluorophosphate acetonitrile solution with the Fc/Fc+ redox couple as an external standard at a scanning rate of 0.05 V s−1. (d) FMO level diagram of the DTQI-based polymer films. | |
The electrochemical properties of DTQI-based polymers were evaluated using CV (Fig. 3c). These two polymers exhibit distinctive reduction peaks, indicating their n-type characteristics. According to the onset of reduction potentials, the LUMO energy levels were −3.60 and −3.63 eV for PDTQI and P(DTQI-BTI), respectively. Due to the weak oxidation peaks, the HOMO levels were calculated based on the LUMO and Eoptg using the equation: EHOMO = ELUMO − Eoptg, which were −5.58 and −5.61 eV for PDTQI and P(DTQI-BTI), respectively. Therefore, it is found that the DTQI building block containing the electron-withdrawing quinoxaline and imide units, provides the DTQI-based polymers with deep LUMO and HOMO energy levels. Such low-lying FMOs should facilitate electron injection and suppress hole accumulation, thus leading to n-type transport characteristics in OTFTs.
Charge transport properties of the n-type polymers
To investigate the charge transport properties of PDTQI and P(DTQI-BTI), OTFTs with a top-gate/bottom contact (TGBC) device structure were fabricated. Polymethyl methacrylate (PMMA) is used as the dielectric and Ag is used as the gate electrode. Chloroform is chosen as the processing solvent and the concentration of organic semiconductors is controlled at 5 mg mL−1. Before evaluation, an annealing process at 100 °C for 20 min was conducted to achieve better morphology. The saturation mobility of OTFTs is calculated according to the square root of drain current (IDS) dependence on gate voltage (VG). The detailed experimental procedures for OTFTs are summarized in the ESI.†Fig. 4a and b show the typical transfer curves and Fig. 4c and d show the corresponding output curves. The related parameters are listed in Table 2. The homopolymer PDTQI exhibits ambipolar charge transport in OTFT. The PDTQI-based device showed μh and μe of 0.005 and 0.012 cm2 V−1 s−1, respectively, while the copolymer P(DTQI-BTI) showed unipolar n-type charge transport and exhibited one order higher electron mobility of 0.25 cm2 V−1 s−1 than PDTQI. Besides, a high on/off current (Ion/Ioff) ratio of around 105 and a relatively low threshold voltage (Vth) of 10 V are also achieved by P(DTQI-BTI).
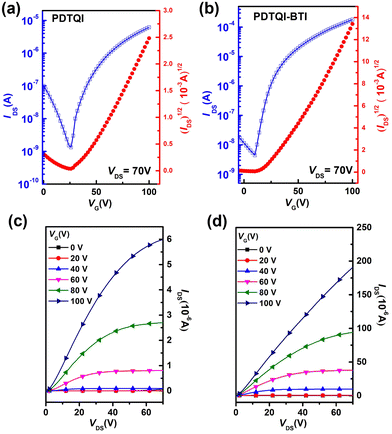 |
| Fig. 4 The transfer (a and b) and output (c and d) curves: (a and c) PDTQI and (b and d) P(DTQI-BTI). | |
Table 2 The OTFT characteristics based on PDTQI and P(DTQI-BTI)
Polymers |
μ
e (cm2 V−1 s−1) |
V
th (V) |
I
on/Ioff ratios |
The average mobility is obtained from 10 devices. |
PDTQI |
0.012 (0.01 ± 0.005) |
∼30 |
∼104 |
P(DTQI-BTI) |
0.25 (0.21 ± 0.005) |
∼10 |
∼105 |
Film morphology of the n-type polymers
The thin-film morphology of PDTQI and P(DTQI-BTI) is studied by atomic force microscopy (AFM) and the corresponding topography images are shown in Fig. 5. According to the topography images, the PDTQI-based film shows relatively amorphous morphology with the root-mean-square (RMS) roughness value of 0.408 nm. For P(DTQI-BTI), its surfaces are rougher with higher RMS values of 0.515 nm, indicating higher crystallinity.66,67 As a result, the higher electron mobility of P(DTQI-BTI) might be due to its stronger aggregation of molecules to form films with higher crystallinity. X-Ray diffraction (XRD) analysis was performed to investigate the crystallinity of PDTQI and P(DTQI-BTI) (Fig. S13, ESI†) under the optimal annealing process (100 °C for 20 min).
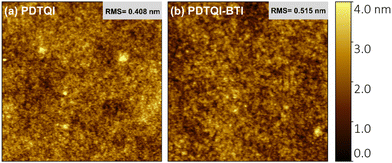 |
| Fig. 5 Tapping-mode AFM topography images of the polymer thin films: (a) PDTQI film and (b) P(DTQI-BTI) film. | |
Conclusions
In summary, a planar and strong electron-deficient building block, DTQI, containing quinoxaline and imide units has been successfully synthesized and incorporated into a π-conjugated polymer backbone. DTQI combines the advantages of both imide and quinoxaline functionalities, showing a highly planar backbone, good solubility, and small steric hindrance. Due to the strong electron-withdrawing ability of the imide and quinoxaline groups, DTQI has significantly enhanced electron-deficient characteristics. As a consequence, DTQI-based acceptor–acceptor type polymers PDTQI and P(DTQI-BTI) showed deep FMO energy levels, which is beneficial for electron transport in OTFTs. The resulting OTFT device based on P(DTQI-BTI) shows unipolar n-type behavior with μe of 0.25 cm2 V−1 s−1. These results demonstrate that a dithiene-fused quinoxalineimide (DTQI) electron-deficient unit is a promising building block for constructing n-type polymer semiconductors.
Conflicts of interest
There are no conflicts to declare.
Acknowledgements
Y. Shi thanks the National Natural Science Foundation of China (No. 22105004).
References
- H. Yan, Z. Chen, Y. Zheng, C. Newman, J. R. Quinn, F. Dötz, M. Kastler and A. Facchetti, Nature, 2009, 457, 679–686 CrossRef CAS PubMed.
- Y. Zhao, Y. Guo and Y. Liu, Adv. Mater., 2013, 25, 5372–5391 CrossRef CAS PubMed.
- C. Zhu, Z. Zhao, H. Chen, L. Zheng, X. Li, J. Chen, Y. Sun, F. Liu, Y. Guo and Y. Liu, J. Am. Chem. Soc., 2017, 139, 17735–17738 CrossRef CAS PubMed.
- J. Yang, Z. Zhao, S. Wang, Y. Guo and Y. Liu, Chem, 2018, 4, 2748–2785 CAS.
- K. Feng, H. Guo, H. Sun and X. Guo, Acc. Chem. Res., 2021, 54, 3804–3817 CrossRef CAS PubMed.
- G. Zhang, H. Yu, Y. Sun, W. Wang, Y. Zhao, L. Wang, L. Qiu and Y. Ding, J. Mater. Chem. C, 2021, 9, 633–639 RSC.
- Y. Sui, Y. Shi, Y. Deng, R. Li, J. Bai, Z. Wang, Y. Dang, Y. Han, N. Kirby, L. Ye and Y. Geng, Macromolecules, 2020, 53, 10147–10154 CrossRef CAS.
- N. Liang, D. Meng and Z. Wang, Acc. Chem. Res., 2021, 54, 961–975 CrossRef CAS PubMed.
- D. Qu, T. Qi and H. Huang, J. Energy Chem., 2021, 59, 364–387 CrossRef CAS.
- W. Yue, M. Nikolka, M. Xiao, A. Sadhanala, C. B. Nielsen, A. J. P. White, H.-Y. Chen, A. Onwubiko, H. Sirringhaus and I. McCulloch, J. Mater. Chem. C, 2016, 4, 9704–9710 RSC.
- D. Patra, J. Lee, S. Dey, J. Lee, A. J. Kalin, A. Putta, Z. Fei, T. McCarthy-Ward, H. S. Bazzi, L. Fang, M. Heeney, M.-H. Yoon and M. Al-Hashimi, Macromolecules, 2018, 51, 6076–6084 CrossRef CAS.
- H. F. Iqbal, M. Waldrip, H. Chen, I. McCulloch and O. D. Jurchescu, Adv. Electron. Mater., 2021, 7, 2100393 CrossRef CAS.
- H. F. Iqbal, Q. Ai, K. J. Thorley, H. Chen, I. McCulloch, C. Risko, J. E. Anthony and O. D. Jurchescu, Nat. Commun., 2021, 12, 2352 CrossRef CAS PubMed.
- Y. Shi, H. Guo, J. Huang, X. Zhang, Z. Wu, K. Yang, Y. Zhang, K. Feng, H. Y. Woo, R. Ortiz, M. Zhou and X. Guo, Angew. Chem., Int. Ed., 2020, 59, 14449–14457 CrossRef CAS PubMed.
- G. Wang, F. S. Melkonyan, A. Facchetti and T. J. Marks, Angew. Chem., Int. Ed., 2019, 58, 4129–4142 CrossRef CAS PubMed.
- Z.-G. Zhang and Y. Li, Angew. Chem., Int. Ed., 2021, 60, 4422–4433 CrossRef CAS PubMed.
- N. Su, R. Ma, G. Li, T. Liu, L.-W. Feng, C. Lin, J. Chen, J. Song, Y. Xiao, J. Qu, X. Lu, V. K. Sangwan, M. C. Hersam, H. Yan, A. Facchetti and T. J. Marks, ACS Energy Lett., 2021, 6, 728–738 CrossRef CAS.
- Y. Shi, R. Ma, X. Wang, T. Liu, Y. Li, S. Fu, K. Yang, Y. Wang, C. Yu, L. Jiao, X. Wei, J. Fang, D. Xue and H. Yan, ACS Appl. Mater. Interfaces, 2022, 14, 5470 Search PubMed.
- Y. Shi, W. Chen, Z. Wu, Y. Wang, W. Sun, K. Yang, Y. Tang, H. Y. Woo, M. Zhou, A. Djurisic, Z. He and X. Guo, J. Mater. Chem. A, 2020, 8, 13754–13762 RSC.
- W. Chen, Y. Shi, Y. Wang, X. Feng, A. B. Djurišić, H. Y. Woo, X. Guo and Z. He, Nano Energy, 2019, 68, 104363 CrossRef.
- A. A. Said, J. Xie and Q. Zhang, Small, 2019, 15, 1900854 CrossRef PubMed.
- J. Liu, Y. Shi, J. Dong, M. I. Nugraha, X. Qiu, M. Su, R. C. Chiechi, D. Baran, G. Portale, X. Guo and L. J. A. Koster, ACS Energy Lett., 2019, 4, 1556–1564 CrossRef CAS.
- Y. Shi, J. Li, H. Sun, Y. Li, Y. Wang, Z. Wu, S. Y. Jeong, H. Y. Woo, S. Fabiano and X. Guo, Angew. Chem., Int. Ed., 2022, 61, e202214192 CAS.
- H. Guo, C.-Y. Yang, X. Zhang, A. Motta, K. Feng, Y. Xia, Y. Shi, Z. Wu, K. Yang, J. Chen, Q. Liao, Y. Tang, H. Sun, H. Y. Woo, S. Fabiano, A. Facchetti and X. Guo, Nature, 2021, 599, 67–73 CrossRef CAS PubMed.
- Y. Lu, J.-Y. Wang and J. Pei, Chem. Mater., 2019, 31, 6412–6423 CrossRef CAS.
- Y. Lu, Z.-D. Yu, R.-Z. Zhang, Z.-F. Yao, H.-Y. You, L. Jiang, H.-I. Un, B.-W. Dong, M. Xiong, J.-Y. Wang and J. Pei, Angew. Chem., Int. Ed., 2019, 58, 11390–11394 CrossRef CAS PubMed.
- A. Tripathi, Y. Lee, S. Lee and H. Y. Woo, J. Mater. Chem. C, 2022, 10, 6114–6140 RSC.
- X. Yan, M. Xiong, J.-T. Li, S. Zhang, Z. Ahmad, Y. Lu, Z.-Y. Wang, Z.-F. Yao, J.-Y. Wang, X. Gu and T. Lei, J. Am. Chem. Soc., 2019, 141, 20215–20221 CrossRef CAS PubMed.
- M. Xiong, X. Yan, J.-T. Li, S. Zhang, Z. Cao, N. Prine, Y. Lu, J.-Y. Wang, X. Gu and T. Lei, Angew. Chem., Int. Ed., 2021, 60, 8189–8197 CrossRef CAS PubMed.
- B. Zhang, C. Ge, C. Xie, K. Lin, W. Yang, B. Liu, X. Gao, Y. Zhou and Q. Zhang, J. Mater. Chem. C, 2022, 10, 17530–17538 RSC.
- M. Comí, D. Patra, R. Yang, Z. Chen, A. Harbuzaru, Y. Wubulikasimu, S. Banerjee, R. Ponce Ortiz, Y. Liu and M. Al-Hashimi, J. Mater. Chem. C, 2021, 9, 5113–5123 RSC.
- Q. Che, W. Zhang, X. Wei, Y. Zhou, H. Luo, J. Wei, L. Wang and G. Yu, CCS Chem., 2023 DOI:10.31635/ccschem.023.202202458.
- Q. Liu, W. He, Y. Shi, S. Otep, W. L. Tan, S. Manzhos, C. R. McNeill, X. Guo, P. Sonar, T. Michinobu and A. K. K. Kyaw, Chem. Mater., 2022, 34, 3140–3151 CrossRef CAS.
- J. Yang, L. Zhao, Z. Yin, J. Wang, Y. Zhao, H. Chen and Y. Liu, Macromolecules, 2021, 54, 3120–3129 CrossRef CAS.
- Y. Shi, H. Guo, M. Qin, J. Zhao, Y. Wang, H. Wang, Y. Wang, A. Facchetti, X. Lu and X. Guo, Adv. Mater., 2018, 30, 1705745 CrossRef PubMed.
- Y. Shi, H. Guo, M. Qin, Y. Wang, J. Zhao, H. Sun, H. Wang, Y. Wang, X. Zhou, A. Facchetti, X. Lu, M. Zhou and X. Guo, Chem. Mater., 2018, 30, 7988–8001 CrossRef CAS.
- Y. Shi, W. Li, X. Wang, L. Tu, M. Li, Y. Zhao, Y. Wang and Y. Liu, Chem. Mater., 2022, 34, 1403–1413 CrossRef CAS.
- X. Guo, A. Facchetti and T. J. Marks, Chem. Rev., 2014, 114, 8943–9021 CrossRef CAS PubMed.
- Y. Wang, H. Guo, S. Ling, I. Arrechea-Marcos, Y. Wang, J. T. López Navarrete, R. P. Ortiz and X. Guo, Angew. Chem., Int. Ed., 2017, 56, 9924–9929 CrossRef CAS PubMed.
- Y. Wang, H. Guo, A. Harbuzaru, M. A. Uddin, I. Arrechea-Marcos, S. Ling, J. Yu, Y. Tang, H. Sun, J. T. López Navarrete, R. P. Ortiz, H. Y. Woo and X. Guo, J. Am. Chem. Soc., 2018, 140, 6095–6108 CrossRef CAS PubMed.
- C. Duan, J. Zhang, J. Xiang, X. Yang and X. Gao, Angew. Chem., Int. Ed., 2022, 61, e202201494 CAS.
- H. Xin, B. Hou and X. Gao, Acc. Chem. Res., 2021, 54, 1737–1753 CrossRef CAS PubMed.
- T. Shen, W. Li, Y. Zhao, Y. Wang and Y. Liu, Adv. Mater., 2023, 35, 2210093 CrossRef CAS PubMed.
- Y. Sui, Y. Deng, T. Du, Y. Shi and Y. Geng, Mater. Chem. Front., 2019, 3, 1932–1951 RSC.
- Y. Wang, E. Zeglio, H. Liao, J. Xu, F. Liu, Z. Li, I. P. Maria, D. Mawad, A. Herland, I. McCulloch and W. Yue, Chem. Mater., 2019, 31, 9797–9806 CrossRef CAS.
- T. Lei, J.-H. Dou, X.-Y. Cao, J.-Y. Wang and J. Pei, J. Am. Chem. Soc., 2013, 135, 12168–12171 CrossRef CAS PubMed.
- K. Feng, H. Guo, J. Wang, Y. Shi, Z. Wu, M. Su, X. Zhang, J. H. Son, H. Y. Woo and X. Guo, J. Am. Chem. Soc., 2021, 143, 1539–1552 CrossRef CAS PubMed.
- Y. Min, C. Dou, D. Liu, H. Dong and J. Liu, J. Am. Chem. Soc., 2019, 141, 17015–17021 CrossRef CAS PubMed.
- X. Shao, M. Liu, J. Liu and L. Wang, Angew. Chem., Int. Ed., 2022, 61, e202205893 CrossRef CAS PubMed.
- K. Zhao, Z.-F. Yao, Z.-Y. Wang, J.-C. Zeng, L. Ding, M. Xiong, J.-Y. Wang and J. Pei, J. Am. Chem. Soc., 2022, 144, 3091–3098 CrossRef CAS PubMed.
- J. Miao, Y. Wang, J. Liu and L. Wang, Chem. Soc. Rev., 2021, 51, 153–187 RSC.
- R. Zhao, J. Liu and L. Wang, Acc. Chem. Res., 2020, 53, 1557–1567 CrossRef CAS PubMed.
- X. Guo and M. D. Watson, Org. Lett., 2008, 10, 5333–5336 CrossRef CAS PubMed.
- B. Liu, M. Böckmann, W. Jiang, N. L. Doltsinis and Z. Wang, J. Am. Chem. Soc., 2020, 142, 7092–7099 CrossRef CAS PubMed.
- W. Jiang, Y. Li and Z. Wang, Acc. Chem. Res., 2014, 47, 3135–3147 CrossRef CAS PubMed.
- Y. Wang, M. Nakano, T. Michinobu, Y. Kiyota, T. Mori and K. Takimiya, Macromolecules, 2017, 50, 857–864 CrossRef CAS.
- Y. Wang and K. Takimiya, Adv. Mater., 2020, 32, 2002060 CrossRef PubMed.
- H. Wang, Q. Shi, Y. Lin, H. Fan, P. Cheng, X. Zhan, Y. Li and D. Zhu, Macromolecules, 2011, 44, 4213–4221 CrossRef CAS.
- M. Comí, M. U. Ocheje, S. Attar, A. U. Mu, B. K. Philips, A. J. Kalin, K. E. Kakosimos, L. Fang, S. Rondeau-Gagné and M. Al-Hashimi, Macromolecules, 2020, 54, 665–672 CrossRef.
- T. Hasegawa, M. Ashizawa, K. Aoyagi, H. Masunaga, T. Hikima and H. Matsumoto, Org. Lett., 2017, 19, 3275–3278 CrossRef CAS PubMed.
- Q. Shi, W.-Q. Chen, J. Xiang, X.-M. Duan and X. Zhan, Macromolecules, 2011, 44, 3759–3765 CrossRef CAS.
- T. Hasegawa, M. Ashizawa, S. Kawauchi, H. Masunaga, N. Ohta and H. Matsumoto, RSC Adv., 2019, 9, 10807–10813 RSC.
- Y. Wang and T. Michinobu, J. Mater. Chem. C, 2016, 4, 6200–6214 RSC.
- Y. Xie, T. Fujimoto, S. Dalgleish, Y. Shuku, M. M. Matsushita and K. Awaga, J. Mater. Chem. C, 2013, 1, 3467 RSC.
- F. A. Arroyave, C. A. Richard and J. R. Reynolds, Org. Lett., 2012, 14, 6138–6141 CrossRef CAS PubMed.
- Y. Wang, A. T. R. Tan, T. Mori and T. Michinobu, J. Mater. Chem. C, 2018, 6, 3593–3603 RSC.
- Y. H. Zhao, W. Li, T. Shen, Y. Zhao, Y. Liu and Y. Wang, Sci. China: Chem., 2023, 66, 548–561 CrossRef CAS.
|
This journal is © The Royal Society of Chemistry 2023 |
Click here to see how this site uses Cookies. View our privacy policy here.