DOI:
10.1039/D3TC01712E
(Paper)
J. Mater. Chem. C, 2023,
11, 10221-10229
Multifunctional optical sensing platform of temperature, pressure (vacuum) and laser power density: NaYF4: Gd3+, Yb3+, Er3+ nanomaterial as luminescent thermometer, manometer and power meter†
Received
17th May 2023
, Accepted 22nd June 2023
First published on 5th July 2023
Abstract
Temperature and pressure are crucial physical parameters in materials science, hence their monitoring is especially important for scientists and engineers. Moreover, the on-target power density parameter is often underestimated, and in various real applications it is difficult to determine it correctly using conventional contact power meters. We developed for the first time, a multi-functional sensing platform allowing ratiometric optical detection of pressure (vacuum), temperature and excitation power density, using a sensor based on the upconverting inorganic nanomaterial (NaYF4: Gd3+, Yb3+, Er3+) emitting in the visible range upon NIR laser excitation. The monotonic dependency between the temperature and the luminescence intensity ratio of the Er3+ thermally-coupled levels (TCLs), i.e. the intensity ratio of the band (525/550 nm), allows to monitor the temperature and laser-induced heating observed under vacuum conditions in the sample, so it is possible to use as a remote vacuum sensor. Moreover, the temperature elevation induced by increasing of the laser power also allows to use it as an optical power density meter. We believe that this work can inspire new research directions that will enable the development of the multi-functional sensor materials.
Introduction
There are many crucial physical parameters in materials science, among them, temperature and pressure are of great importance in a wide range of scientific research and industrial operations.1–9 For this reason, fast, accurate and remote measurements have increasingly attracted the attention of researchers.3–7,10,11 For measurements of these two parameters remotely, is usually used the optical techniques based on organic complexes, d-block metal ions and on the luminescence of lanthanides are usually used.3–7,10–14 In addition, working with variable laser power involves an additional difficulty in various non-linear optical measurements, trying to get the exact spot size and determine the on-target power density.15
The great interest in lanthanide (Ln) ions for optical methods is due to their wide variety of useful spectroscopic properties, among them wide-range multicolor emission, long short Stokes shift, luminescence lifetimes, narrow emission lines, etc., all this properties are related with the 4f–4f electronic transitions.3–7,16–20 Other advantage that have the inorganic materials doped with lanthanide ions is the good responses to pressure and temperature, so they can function as luminescent manometers and thermometers.3–7,10–13 Although, there are currently not many pumping power sensors, so their use as power meters is limited, for the moment.20,21 Materials containing Nd3+, Er3+ and Tm3+ are commonly used as luminescent thermometers for temperature sensing. This is due to the presence of thermally coupled levels (TCL) that are separated by a small energy difference (approximately 50–2000 cm−1). As a result, the emission from these materials follows a Boltzmann-type behavior.3,4,6,7,10–13,22–25 On the other hand, to measure the laser (pump) power density, one already reported approach is to use materials containing Cr3+ ions, which exhibit well-defined laser-induced self-heating behaviour, directly affecting the luminescence intensity ratio (local variation of temperature) that occurs between the 4T2(g) level and the 2E(g) level.21 For pressure sensing, inorganic materials are typically used, mainly thanks to their high structural stability and resistance to compression–decompression cycles. For the optical monitoring of high pressure systems, the characteristic emissions of the Eu2+ and Sm2+ ions embedded in different inorganic matrices are very sensitive to pressure changes.26–29 These materials are good alternative to the fluorescent ruby sensor (Al2O3:Cr3+), whose emission is highly temperature dependent.30–32 On the other hand, for low pressure (vacuum), it is possible to employ inorganic materials that exhibit a light-to-heat conversion by pressure-modulated or organic complexes (dyes) that demonstrate oxygen-dependent quenching of emission, in which luminescence thermometry controls heating–cooling processes.10,11,33,34 However, oxygen-sensitive organic dyes have a limited range of pressure where they detect, typically from ≈0.05 to 2 bar,34 so the latter ones seem to be a better alternative for vacuum sensing in a broader range (≈10−5–1 bar).
In luminescence thermometry, there are several approaches which allow the development of optical thermometers of micrometric or nanometric size, working in a wide temperature range.6,12,13,22–25,35–37 They typically exploit changes in luminescence lifetimes or band intensity ratio of two excited levels to determine temperature.6,12,13,20,23,35–41 In addition, optical pressure sensors have the ability to operate over a wide range from vacuum to high pressure.3–5,7,10,11,41 Because their operating mechanisms are different, i.e. high-pressure sensors are based on material compression, which directly affects the spectroscopic properties of optically active ions, such as changes in lifetimes, emission line width, luminescence band intensity ratios or line shift.2–5,7,41 On the other hand, low-pressure luminescent sensors (operating below 1 bar) utilize the loss of concentration of gas molecules, which directly affects the local temperature rise or the quenching of luminescence (emission intensity variations), this discovery enables the design of vacuum sensors using the luminescent thermometers/heaters, this view is based on the effect of laser heating of materials, which is enhanced in vacuum conditions.10,11,33,34
In this work, we show that NaYF4: Gd3+, Yb3+, Er3+, nanomaterial can be used as a multifunctional detector for temperature and pressure using the vacuum-enhanced light-to-heat conversion (see Fig. 1(a)).42 The developed sensor can operate in a temperature range from 300 to 600 K and in a pressure range from ≈10−4 to 1 bar. In addition, this material can be used as a power density detector using two different approaches, i.e. the change in the band intensity ratio of Er3+ TCLs is due to the local temperature change induced with laser heating, as well as by utilizing the well-defined and non-linear variation of upconversion (UC) emissions, which depends on the laser power density used. Please note, that the first strategy is valid in the high-power range (≈5–850 W cm−2) and the second method can be used operating in a low-power density regime (≈0.2–200 W cm−2).
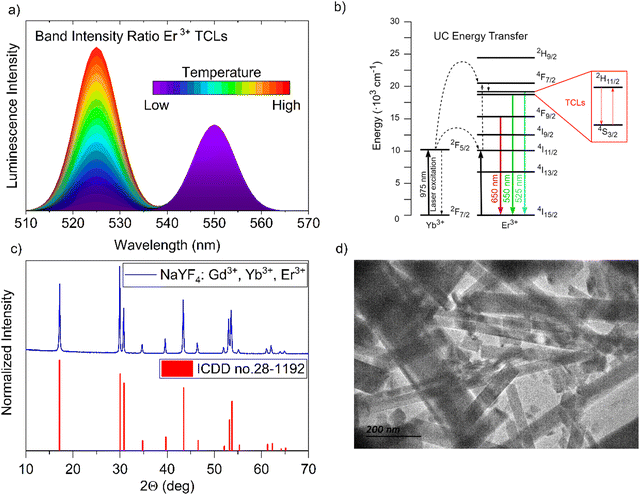 |
| Fig. 1 (a) Concept of temperature-dependent emission bands in Er3+-doped luminescent materials. (b) Er3+ and Yb3+ ion energy level diagram illustrating the principal processes occurring in the system, focusing on Er3+ TCLs 2H11/2 and 4S3/2. (c) comparison of the XRD pattern of the synthesized NaYF4:Gd3+, Yb3+, Er3+ nanomaterial with the reference data. (d) representative TEM image of the obtained NaYF4:Gd3+, Yb3+, Er3+ nanoparticles crystallizing in a hexagonal structure. | |
Results and discussion
Characteristics of the sensor material
The luminescent sensor material based on the nano-sized NaYF4 particles, co-doped with 78% of Y3+ and doped with a 10% of Yb3+, 10% of Gd3+ and 2% of Er3+ ions, was obtained through the modified hydrothermal synthesis method described by Zhang et al.43 The investigated fluoride nanomaterial is optically active and exhibits non-linear response to the excitation light, i.e. the anti-Stokes emission of Er3+, that is commonly observed in material with Yb3+–Er3+ ions, can generate UC luminescence when the sample is excitated by laser irradiation (≈975 nm). In such systems, the Yb3+ (“light-harvesting ions”) absorbs the radiation of the incident laser and transfer it to the neighboring Er3+ ions (emitters), causing an UC energy transfer process (energy level diagram in Fig. 1(b)).4,10,38 The use of Gd3+ ions allows lowering the reaction temperature during the synthesis process, ensuring the formation of the desired crystal structure.44 The synthesized nanomaterial crystallizes in a hexagonal crystal system, P
space group, and its XRD pattern agrees well with the reference data from the ICDD database (International Centre for Diffraction Data), of hexagonal β-NaYF4 (card no. 28-1192)45 as can be seen in Fig. 1(c). The low phonon energy of its crystal lattice, i.e. ≈370 cm−1,46,47 reduces the probability of nonradiative excited states depopulation, facilitating the generation of efficient UC luminescence.48Fig. 1(d) shows the transmission electron microscopy (TEM) image of the obtained nanocrystals, revealing their elongated, rod-like shape, with an average width of ≈50–100 nm and maximum length reaching up to ≈1 μm. These results also agree with the data from the scanning electron microscopy (SEM) shown in the ESI† in Fig. S1. The performed elemental analysis reveals that the atomic concentration of lanthanides in the nanocrystals is consistent with that introduced during the synthesis process in the hydrothermal reactor, as indicated in Table S1 in the ESI† data. It is worth noting, that the material studied is stable under high-temperature condition, up to at least ≈700 K, preserving its hexagonal structure.49
Temperature sensing
The monotonic thermal change of the band intensity ratio is the results of the thermal coupling between the 2H11/2 and 4S3/2 states, with ΔE ≈ 866 ± 5 cm−1 (value determined from the spectra, using the centroids of the 525 and 550 nm emissions).6 For the calibration of the UC response from the NaYF4:Gd3+, Yb3+, Er3+ sample, its UC emission spectra were measured using a continuous wave 975 nm NIR laser in a range of temperature (≈300–600 K), with power density of ≈0.1 W cm−2. Results from Fig. 2(a) clearly confirms the temperature dependence relationship of the Er3+ thermalized bands and their radiative transitions 2H11/2 → 4I15/2 (≈525 nm) and 4S3/2 → 4I15/2 (≈550 nm), according to the diagram shown in Fig. 1(b). From the recorded spectra, by integrating the area under the bands of interest, the band intensity ratio of 525/550 nm was determined. This band intensity ratio values are plotted in Fig. 2(b) as a function of temperature, and follow Boltzmann-type behavior using the following function: | 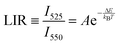 | (1) |
where LIR is the luminescence (band) intensity ratio (525/550 nm); ΔE is the energy difference between the analyzed excited states; kB is the Boltzmann constant; T is the absolute temperature; and A is a constant which depends on the states degeneracies, branching ratio of the transitions in relation to the ground state, spontaneous emission rates and energy of the transitions.6 The performed fitting resulted in ΔE ≈ 776 ± 6 cm−1 (most plausibly due to band overlapping this experimental value is lower than that obtained theoretically.), A ≈ 7.28 and R2 ≈ 0.99. To ensure good sensitivity and high accuracy of the optical temperature determination, the remote ratiometric method commonly used in luminescence thermometry was used. In order to compare the performance of different sensors (independently of the measuring setup and thermometric parameter used), it is useful to calculate its relative sensitivity (Sr) given by the formula: | 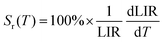 | (2) |
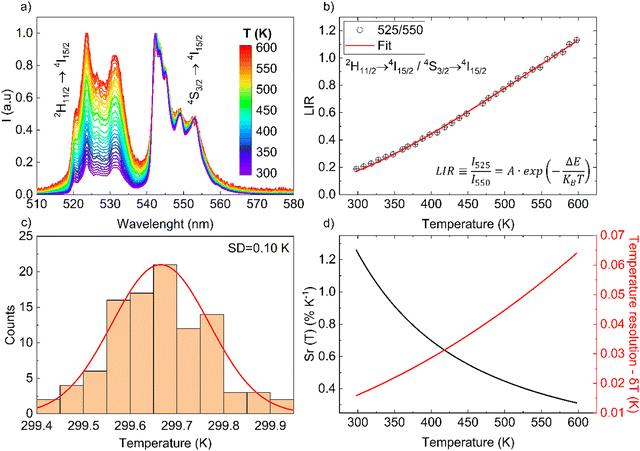 |
| Fig. 2 (a) Normalized UC emission spectra as a function of temperature, measured at λex = 975 nm and 0.1 W cm−2. (b) Determined band intensity ratios as a function of temperature. (c) Temperature distribution. (d) Relative sensitivity and Resolution as a function of temperature. | |
The sensor sensitivity developed depends on the temperature range of interest, i.e. Sr(T) parameter varies significantly, and at room temperature it reaches ≈1.26% K−1 (see Fig. 2(d)), which is high compared to other Er3+-based sensors. The performance comparison of the luminescent thermometer is given in Table 1.
Table 1 Comparison of performance of different luminescent thermometers (Er3+-based), power density meters and optical vacuum sensors
Thermometers |
Host |
Emitting ion |
Relative sensitivity Sr(T) [% K−1] |
Transitions |
λ [nm] |
Ref. |
NaYF4 |
Er3+ |
1.26 |
2H11/2 → 4I15/2/4S3/2 → 4I15/2 |
525/550 |
This work |
β-NaYF4/SiO2 core/shell nanorods |
Er3+ |
1.31 |
2H11/2 → 4I15/2/4S3/2 → 4I15/2 |
525/550 |
54
|
YF3-modified cellulose fibers |
Er3+ |
1.2 |
2H11/2 → 4I15/2/4S3/2 → 4I15/2 |
525/550 |
55
|
BaTiO3 |
Er3+ |
1.21 |
2H11/2 → 4I15/2/4S3/2 → 4I15/2 |
525/550 |
56
|
Y2SiO5 |
Er3+ |
0.7 |
2H11/2 → 4I15/2/4S3/2 → 4I15/2 |
525/550 |
57
|
Gd2O3–AuNP |
Er3+ |
0.72 |
2H11/2 → 4I15/2/4S3/2 → 4I15/2 |
525/550 |
58
|
La2CaZnO5 |
Er3+ |
0.71 |
2H11/2 → 4I15/2/4S3/2 → 4I15/2 |
525/550 |
59
|
Y2Mo3O12 |
Er3+ |
0.79 |
2H11/2 → 4I15/2/4S3/2 → 4I15/2 |
525/550 |
60
|
YVO4 |
Er3+ |
0.8 |
2H11/2 → 4I15/2/4S3/2 → 4I15/2 |
525/550 |
10
|
Power density meters |
Host |
Emitting ion |
Relative sensitivity Sr(I) [% W−1 cm2] |
Transitions |
λ (nm) |
Ref. |
NaYF4 |
Er3+ |
0.46 |
2H11/2 → 4I15/2/4S3/2 → 4I15/2 |
525/550 |
This work |
835 |
2H11/2 → 4I15/2 |
525 |
GdAl3(BO3)4 |
Cr3+ |
0.07 |
2E → 4A2/4T2 → 4A2 |
750/690 |
21
|
Optical vacuum sensors |
Host |
Emitting ion |
Relative sensitivity Sr(T) [% mbar−1] |
Transitions |
λ (nm) |
Ref. |
NaYF4 |
Er3+ |
20 (at 1 mbar) |
2H11/2 → 4I15/2/4S3/2 → 4I15/2 |
525/550 |
This work |
1.1 (at 10 mbar) |
YPO4 |
Er3+ |
10 (at 1 mbar) |
2H11/2 → 4I15/2/4S3/2 → 4I15/2 |
525/550 |
42
|
1 (at 10 mbar) |
YVO4 |
Er3+ |
40 (at 1 mbar) |
2H11/2 → 4I15/2/4S3/2 → 4I15/2 |
525/550 |
10
|
1 (at 10 mbar) |
YAlO3 (microsphere) |
Nd3+ |
<0.1 (at 1 mbar) |
4F3/2 → 4I9/2 |
914 |
11
|
PtTFPL |
Organic complex |
≈0.1 |
Triplet → singlet |
740 |
53
|
PtTFPL |
Organic complex |
≈0.1 |
Triplet → singlet |
650 |
61
|
Fig. 2(c) shows a histogram obtained based on the 100 spectra recorded under the same conditions (room temperature), from which a standard deviation of about 0.10 K can be obtained. This value can be used as an estimation of the experimental temperature error which has this temperature sensing method. In addition, a theoretical estimation of the temperature resolution δT can be also calculated using equation:
|  | (3) |
where δLIR is the uncertainty of determination of the band intensity ratio 525/550 nm and is estimated from the LIR error in the 100 measurements.
6 It can be seen that the theoretical error at room temperature is ≈0.01 K, which is smaller than the experimental value of ≈0.10 K (more reliable). As expected, according to
Fig. 2(d), when the temperature increases, the error and therefore the uncertainty of temperature determination grows, which can be explained in terms of the reduction of the signal-to-noise ratio and
Sr values with temperature.
Optical power density meter
Next, the UC emission spectra were measured as a function of laser power density (0.27–930 W cm−2) at ambient conditions (Fig. 3). As can be seen, the LIR parameter starts to significantly increase at pump power values above ≈10 W cm−2 (Fig. 4(a)). The increase in laser power density directly influences local temperature of the sample (see Fig. S2, ESI†), as it can be seen by correlating the LIR values determined from the temperature measurements (Fig. 2(b)) with the LIR values obtained from the measurements performed as a function of laser power (Fig. 4(a)). The monotonic change of LIR in the ≈5–850 W cm−2 optical power density range reveals wide operating range of the power meter based on NaYF4:Gd3+, Yb3+, Er3+. In a similar way to the previous study, the main parameters that characterize the sensor performance are the relative sensitivity and the sensing resolution for pump power density, which can be calculated as: | 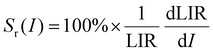 | (4) |
and | 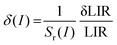 | (5) |
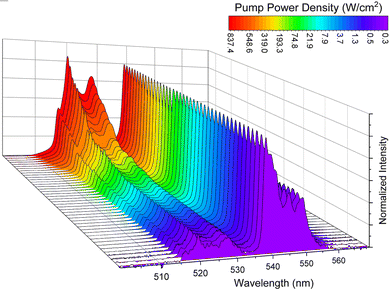 |
| Fig. 3 Normalized UC emission spectra as a function of of pump power density of the NaYF4:Gd3+, Yb3+, Er3+ material, measured at λex = 975 nm. | |
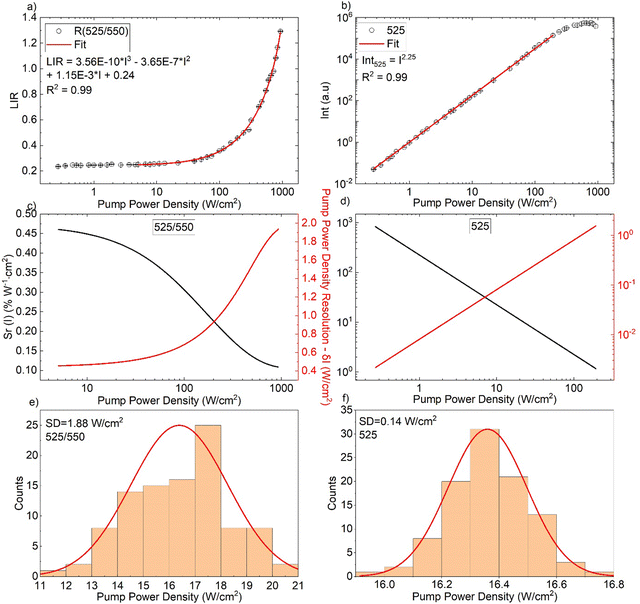 |
| Fig. 4 (a) Determined band intensity ratios of 525/550 nm as a function of pump power density (I). (b) Intensity of the 525 nm as a function of pump power density. (c), (d) The corresponding sensitivity and resolution density. (e), (f) Histograms showing the determined pump power density distributions (experimental resolutions) for the LIR- (e) and intensity-based sensing modes (f). | |
With respect to the Sr(I), the available literature data related to power meter is very restricted. (see Table 1). Depending on the excitation power density range of interest, using the 525/550 nm band ratio the Sr(I) varies between ≈0.46 and 0.1% W−1 cm2 (see Fig. 4(c)), which is higher than for the first optical power density meter reported by Marciniak et al.21 Moreover, in order to broaden the operating range and improve the relative sensitivity of the sensor at low power densities, we have proposed another sensing method. Namely, the non-linear variation of the 525 nm band intensity with the laser power has been analyzed and plotted in Fig. 4(b). The corresponding Sr(I) and δ(I) parameters for this approach can be obtained as follows:
| 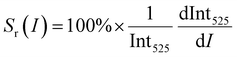 | (6) |
and
| 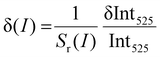 | (7) |
where Int
525 is the intensity and δInt
525 is the uncertainty of determination of the 525 nm band intensity. The calculated intensity values of the 525 nm band
vs. the pump power density (plotted in log–log representations if
Fig. 4(b)) show a clear and linear behaviour, starting from ≈0.27 W cm
−2 and ending around 200 W cm
−2, which is also the operating range for this sensing mode. The further change of this tendency (plateau observed around 500 W cm
−2) is most plausibly due to the saturation effect, which is typically observed for UC luminescence, as well as due to the increasing laser-induced heating of the sample in the high power regime.
50–52 A very high relative sensitivity of the intensity-based parameter obtained in the low power range,
e.g. Sr(
I) ≈ 835% W
−1 cm
2 at 0.27 W cm
−2 (see
Fig. 4(d)), compensates the lack of sensitivity of the LIR-based sensing method in this range. It should be kept in mind, that any deviations in the laser power, as well as scattering and reabsorption effects of the incident photons (in the sample is immersed in any non-transparent medium) can affect the intensity of the emission band, affecting the optical readouts of the power density. It can be seen from the histograms (
Fig. 4(e) and (f)) that for a selected power density of ≈16 W cm
−2 the experimental error for the LIR-based sensing method. For the intensity-based one, are ≈1.88 and 0.14 W cm
−2, respectively. Similarly, like in the case of temperature sensing, the experimentally determined uncertainties for the power density readouts (sensing resolution) are larger than the theoretically estimated values.
Low pressure (vacuum) sensing
For the pressure, a set of UC spectra has been measured using a fixed laser (≈20 W cm−2) and at different vacuum levels (see Fig. 5(a)). The TCLs of Er3+ associated with the ratio of band intensities (525/550 nm) clearly increase with changes in the vacuum level of the system (1000–0.07 mbar), as can be seen in Fig. 5(a) and (b), confirming the postulated enhancement of light-heat conversion under vacuum conditions (see Fig. S3, ESI†). While at ambient pressure, the abundant concentration of air molecules (cooling gas) transfers the generated heat to the surroundings, under vacuum conditions the amount of air molecules is limited significantly, for this reason the sample heats up because the heat dissipation (convection) is less efficient.42
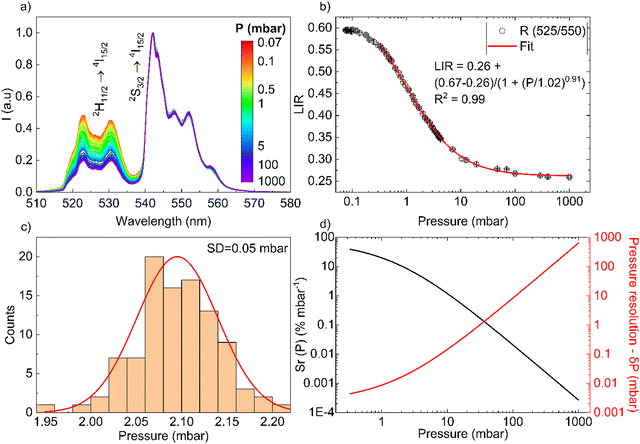 |
| Fig. 5 (a) UC emission spectra of the NaYF4:Gd3+, Yb3+, Er3+ material as a function of pressure, measured under (λex = 975 nm and 20 W cm−2). (b) Determined band intensity ratios as a function of pressure. (c) Histogram of the experimentally determined distribution of pressure values around 2 mbar. (d) Sensitivity and pressure sensing resolution as a function of pressure. | |
The determined LIR values are plotted in Fig. 5(b) in logarithmic representation, and correlated with the vacuum level of the system, from 0.33 to 1000 mbar (R2 = 0.99), using a logistic fit. The relative sensitivity and pressure resolution were estimated based on the following equations:
| 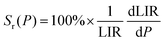 | (8) |
and
|  | (9) |
The Fig. 5(d) presents the calculated relative pressure sensitivity Sr(P) and the resolution of pressure sensing δP. For the pressure reduction, the value of Sr(P) increase, begins at atmospheric pressure with 3 × 10−4% mbar−1 and up to ≈40% mbar−1 (at around 0.3 mbar).
Finally, with the available literature data in Table 1, we have compared the performance of the developed luminescent sensor, but the data of vacuum sensors are very limited for those working at low pressure, i.e. around 0.1 mbar. The sensitivity of the sensor depend of the pressure range of interest, i.e. around 1 mbar, if we compare the Sr(P) values, from the different sensors, our sensor have ≈20% mbar−1, this is lower compared to the YVO4,10 but higher compared to the YPO4 sensor,42 YAlO3 perovskite-based sensor (microsphere),11 or organic sensors.53 However, at a pressure of around 10 mbar, the developed sensor has Sr(P) ≈ 1.1% mbar−1, approximately the same as for the vanadate (perovskite sensor does no operate in this range) and the phosphate sensor. Nonetheless, the proposed sensor can be used with acceptable resolution in the range of 0.33–100 mbar, as shown in Fig. 5(d).
Conclusion
In this work, we have demonstrated for the first time the use of lanthanide-doped UC nanomaterial (NaYF4:Gd3+, Yb3+, Er3+) as a multifunctional optical sensor of temperature, laser power density and pressure (vacuum), operating in the visible range upon NIR excitation at 975 nm. The detection of these three parameters (T, I, P) is based on heating the UC material and using the Er3+ band intensity ratio (525/550 nm) as a sensing parameter. The applied detection methods provide good sensitivity of optical monitoring of temperature (≈300–600 K), laser power (≈0.2–850 W cm−2) and pressure (≈0.33–1000 mbar), ensuring accurate determination of the given quantities. In addition, for the detection of laser power, we developed a new bi-modal sensing strategy, using either the ratiometric approach or the single band emission intensity (525 nm) to improve the sensitivity of the material in the low power density regime. Another particularly important advantage of this sensor is its non-invasive character (remote detection), which allows detection in different environments. Moreover, it is also worth noting that this material presents the possibility of use as an optical nano-heater for various applications. Provided that to measure any of the three parameters, the other two must be controlled, otherwise it will induce a change in the LIR and it will not be known if it is due to a change in the study parameter. This study may be the beginning for a new class of sensors based on single compound for the multi-parameter detection of diverse temperature-dependent physical quantities.
Experimental section
Materials
All chemicals and reagents were commercially available and used without further purification unless otherwise stated. Y(NO3)3·6H2O (99,99%), Gd(NO3)3·6H2O (99,99%), Yb(NO3)3·5H2O (99,99%), and Er(NO3)3·5H2O (99,99%), were purchased from Sigma Aldrich, oleic acid from Fisher Chemicals, and NaOH (pellets) (99%) and NaF (99%), from Scharlau Chemie. For all experiments the ultra-pure, deionised water was used.
Synthesis
The UC nanoparticles were obtained following the modified procedure reported by Zhang et al.43 At a first stage, 7.1 g of oleic acid was added to a 50 mL Erlenmeyer flask, followed by the addition of 0.7 g of NaOH (pellets) under vigorous stirring. After 2 minutes, 13 mL of ethanol was added and the suspension was heated to 30–40 °C in a water bath. Then, a solution of 300 mg of NaF (7.2 mmol) in 8.3 mL of deionized water was added to the reaction flask. When all reagents were completely dissolved, a solution of 1.12 mmol of Ln(NO3)3 [containing the following mole % of lanthanide ions: Y(III) 78%, Gd(III) 10%, Yb(III) 10%, and Er(III) 2%] in 1.5 mL of deionized water was added slowly with vigorous stirring. A white precipitate is formed and the suspension was heated to 40–50 °C for 10 min. Then, the suspension was placed in a hydrothermal autoclave reactor and heated to 230 °C for 12 hours. Once the reactor was cooled, the organic phase was decanted and discarded, and the solid material was washed with 25 mL of ethanol and centrifuged at 3000 rpm for 5 min. This process was repeated three times. Finally, the product was washed twice with 10 mL of deionized water and placed on a stove at 90 °C overnight.
Characterization
X-ray powder diffraction patterns were recorded at room temperature on a PANanalytical X’pert X-ray diffractometer with Cu Kα radiation (1.54184 Å). A transmission electron microscope (TEM), JEOL JEM 2100 with 0.24 nm resolution with an X-ray dispersive energy microanalyzer (EDX) Oxford X-MAX 80 mm2 was used to identify the shape of the nanoparticles. UC emission spectra were recorded in the visible range using an Andor Shamrock 500 spectrometer, coupled to the Andor Newton silicon CCD camera. The excitation source was a tunable CW Ti:Sapphire laser system, Spectra Physics 3900S pumped with a 15 W 532 nm Spectra Physics Millenia, adjusted at 975 nm.
Measurements
For the all experiments, a pellet was made from the material (≈260 μm thick) and placing the material on a small glass plate. For optical measurements of the sample as a function of temperature, the luminescence measurements were performed from 300 to 600 K using a tubular electric furnace (Gero RES-E 230/3), and a K-type thermocouple was used to control the temperature of the sample. In order to measure the laser power, a photodiode (Ophir StarLite) was used. Finally, for low pressure measurements, vacuum was obtained using an oil pump (Edwards RV3), where the manometer was connected to a digital controller (Edwards Active Gauge Controller Single Display).
Author contributions
C.H.A.: data curation, formal analysis, investigation, validation, writing – original draft. G. B. S.: investigation, writing – review & editing. I. R. M.: validation, investigation, project administration, supervision, writing – review & editing. J. S.: formal analysis, validation, writing – review & editing. K. S: data curation, writing – review & editing. K. S. C.: sotfware, writing – review & editing. Ł. M.: validation, writing – review & editing. M. R.: conceptualization, data curation, validation, funding acquisition, investigation, supervision, writing – review & editing.
Conflicts of interest
The authors declare no competing financial interest.
Acknowledgements
This work was financially supported by Ministerio de Ciencia e Innovacion of Spain (MICIIN) under the National Program of Sciences and Technological Materials (PID2019-106383GB-C44 and PID2019-107335RA-I00) and Gobierno de Canarias (PROID2021010102 and PROID2020010067) and EU-FEDER funds. M. R. acknowledges support from Fondo Social Europeo and Agencia Estatal de Investigación (RYC2020-028778-I/AEI/10.13039/501100011033).
References
- Y. Fei and Y. Wang, Rev. Mineral. Geochem., 2000, 41, 521–557 CrossRef.
-
J. M. Recio, J. M. Menendez and A. Otero de la Roza, An Introduction to High-Pressure Science and Technology, CRC Press, Portland, 2016 Search PubMed.
- M. A. Antoniak, S. J. Zelewski, R. Oliva, A. Żak, R. Kudrawiec and M. Nyk, ACS Appl. Nano Mater., 2020, 3, 4209–4217 CrossRef CAS.
- S. Goderski, M. Runowski, P. Woźny, V. Lavín and S. Lis, ACS Appl. Mater. Interfaces, 2020, 12, 40475–40485 CrossRef CAS PubMed.
- T. Tröster, Handb. Phys. Chem. Rare Earths, 2003, 33, 515–589 Search PubMed.
- C. D. S. Brites, A. Millán and L. D. Carlos, Handb. Phys. Chem. Rare Earths, 2016, 49, 339–427 CAS.
- M. Runowski, A. Shyichuk, A. Tymiński, T. Grzyb, V. Lavín and S. Lis, ACS Appl. Mater. Interfaces, 2018, 10, 17269–17279 CrossRef CAS PubMed.
- K. Dziubek, M. Citroni, S. Fanetti, A. B. Cairns and R. Bini, J. Phys. Chem. C, 2017, 121, 2380–2387 CrossRef CAS.
- A. Katrusiak, Acta Crystallogr., Sect. B: Struct. Sci., Cryst. Eng. Mater., 2019, 75, 918–926 CrossRef CAS.
- M. Runowski, P. Woźny, S. Lis, V. Lavín and I. R. Martin, Adv. Mater. Technol., 2020, 5, 1901091 CrossRef CAS.
- K. Soler-Carracedo, I. R. Martin, M. Runowski, L. L. Martín, F. Lahoz, A. D. Lozano-Gorrín and F. Paz-Buclatin, Adv. Opt. Mater., 2020, 8, 2000678 CrossRef CAS.
- D. Jaque and F. Vetrone, Nanoscale, 2012, 4, 4301–4326 RSC.
- M. D. Dramićanin, J. Appl. Phys., 2020, 128, 40902 CrossRef.
- P. Shi, Y. Duan, W. Wei, Z. Xu, Z. Li and T. Han, J. Mater. Chem. C, 2018, 6, 2476–2482 RSC.
- A. Bednarkiewicz, L. Marciniak, L. D. Carlos and D. Jaque, Nanoscale, 2020, 12, 14405–14421 RSC.
- J. G. Bünzli and C. Piguet, Chem. Soc. Rev., 2005, 34, 148–177 RSC.
-
M. Sato, S. W. Kim, Y. Shimomura, T. Hasegawa, K. Toda and G. Adachi, in Chapter 278 – Rare Earth-Doped Phosphors for White Light-Emitting Diodes, ed. B. Jean-Claude and P. Vitalij, Elsevier, 2016, p.1 Search PubMed.
- J. G. Buenzli, Trends Chem., 2019, 1, 751–762 CrossRef.
- B. Golesorkhi, H. Nozary, A. Fürstenberg and C. Piguet, Mater. Horiz., 2020, 7, 1279–1296 RSC.
- F. Maturi, C. Brites, R. Silva, K. Nigoghossian, D. Wilson, R. Ferreira, S. Ribeiro and L. Carlos, Adv. Photonics Res., 2021, 3, 2100227 CrossRef.
- L. Marciniak, M. Szalkowski, A. Bednarkiewicz and K. Elzbieciak-Piecka, J. Mater. Chem. C, 2022, 10, 11040–11047 RSC.
- R. G. Geitenbeek, H. W. de Wijn and A. Meijerink, Phys. Rev. Appl., 2018, 10, 064006 CrossRef CAS.
- P. Du, L. Luo, H. Park and J. S. Yu, Chem. Eng. J., 2016, 306, 840–848 CrossRef CAS.
- P. Du, L. Laihui, W. Li, Y. Qingying and H. Chen, Appl. Phys. Lett., 2014, 104, 152902 CrossRef.
- M. Runowski, P. Woźny, N. Stopikowska, I. R. Martin, V. Lavín and S. Lis, ACS Appl. Mater. Interfaces, 2020, 12, 43933–43941 CrossRef CAS PubMed.
- S. V. Rashchenko, A. Kurnosov, L. Dubrovinsky and K. D. Litasov, J. Appl. Phys., 2015, 117, 145902 CrossRef.
- T. Zheng, M. Runowski, P. Woźny, S. Lis and V. Lavín, J. Mater. Chem. C, 2020, 8, 4810–4817 RSC.
- M. Runowski, P. Woźny, V. Lavín and S. Lis, Sens. Actuators, B, 2018, 273, 585–591 CrossRef CAS.
- Y. Wang, T. Seto, K. Ishigaki, Y. Uwatoko, G. Xiao, B. Zou, G. Li, Z. Tang, Z. Li and Y. Wang, Adv. Funct. Mater., 2020, 30, 2001384 CrossRef CAS.
- H. K. Mao, J. Xu and P. M. Bell, J. Geophys. Res., 1986, 91, 4673–4676 CrossRef CAS.
- A. Dewaele, M. Torrent, P. Loubeyre and M. Mezouar, Phys. Rev. B: Condens. Matter Mater. Phys., 2008, 78, 104102 CrossRef.
- F. Datchi, A. Dewaele, P. Loubeyre, R. Letoullec, Y. L. Godec and B. Canny, High Press. Res., 2007, 27, 447–463 CrossRef CAS.
- J. W. Gregory, K. Asai, M. Kameda, T. Liu and J. P. Sullivan, Proc. Inst. Mech. Eng., Part G, 2008, 222, 249–290 CrossRef CAS.
- S. M. Peak and A. N. Watkins, ACS Appl. Nano Mater., 2020, 3, 9813–9821 CrossRef CAS.
- P. Cortelletti, A. Skripka, C. Facciotti, M. Pedroni, G. Caputo, N. Pinna, M. Quintanilla, A. Benayas, F. Vetrone and A. Speghini, Nanoscale, 2018, 10, 2568–2576 RSC.
-
A. Benayas, E. Hemmer, G. Hong and D. Jaque, Near Infrared-Emitting Nanoparticles for Biomedical Applications, Springer International Publishing AG, Cham, 2020 Search PubMed.
- C. D. S. Brites, K. Fiaczyk, J. F. C. B. Ramalho, M. Sójka, L. D. Carlos and E. Zych, Adv. Opt. Mater., 2018, 6, 1701318 CrossRef.
- S. Balabhadra, M. L. Debasu, C. D. S. Brites, R. A. S. Ferreira and L. D. Carlos, J. Phys. Chem. C, 2017, 121, 13962–13968 CrossRef CAS.
- K. Trejgis, A. Bednarkiewicz and L. Marciniak, Nanoscale, 2020, 12, 4667–4675 RSC.
-
L. Marciniak, K. Elzbieciak-Piecka, K. Kniec and A. Bednarkiewicz, Chem. Eng. J., 2020, 388, 124347 Search PubMed.
-
M. Runowski, Pressure and Temperature Optical Sensors: Luminescence of Lanthanide-Doped Nanomaterials for Contactless Nanomanometry and Nanothermometry, in Handbook of Nanomaterials in Analytical Chemistry, ed. C. M. Hussain, Elsevier, 2020, pp. 227–273 Search PubMed.
- M. Runowski, P. Woźny and I. R. Martín, J. Mater. Chem. C, 2021, 9, 4643–4651 RSC.
- F. Zhang, Y. Wan, T. Yu, F. Zhang, Y. Shi, S. Xie, Y. Li, L. Xu, B. Tu and D. Zhao, Angew. Chem., Int. Ed., 2007, 46, 7976–7979 CrossRef CAS PubMed.
- K. Zheng, K. Y. Loh, Y. Wang, Q. Chen, J. Fan, T. Jung, S. H. Nam, Y. D. Suh and X. Liu, Nano Today, 2019, 29, 100797 CrossRef CAS.
-
H. Fujii, Private Communication, Central Research Laboratory Hitachi Limited, Tokyo, Japan, 1975 Search PubMed.
- J. F. Suyver, J. Grimm, M. K. van Veen, D. Biner, K. W. Krämer and H. U. Güdel, J. Lumin., 2006, 117, 1–12 CrossRef CAS.
- M. M. Lage, R. L. Moreira, F. M. Matinaga and J. Gesland, Chem. Mater., 2005, 17, 4523–4529 CrossRef CAS.
- C. Renero-Lecuna, R. Martín-Rodríguez, R. Valiente, J. González, F. Rodríguez, K. W. Krämer and H. U. Güdel, Chem. Mater., 2011, 23, 3442–3448 CrossRef CAS.
- R. A. Janjua, C. Gao, R. Dai, Z. Sui, M. A. Ahmad Raja, Z. Wang, X. Zhen and Z. Zhang, J. Phys. Chem. C, 2018, 122, 23242–23250 CrossRef CAS.
- M. Pollnau, D. R. Gamelin, S. R. Lüthi, H. U. Güdel and M. P. Hehlen, Phys. Rev. B: Condens. Matter Mater. Phys., 2000, 61, 3337–3346 CrossRef CAS.
- Y. Lei, H. Song, L. Yang, L. Yu, Z. Liu, G. Pan, X. Bai and L. Fan, J. Chem. Phys., 2005, 123, 174710 CrossRef PubMed.
- M. Runowski, P. Woźny, S. Lis, V. Lavín and I. R. Martin, Adv. Mater. Technol., 2020, 5, 1901091 CrossRef CAS.
- B. Zelelow, G. E. Khalil, G. Phelan, B. Carlson, M. Gouterman, J. B. Callis and L. R. Dalton, Sens. Actuators, B, 2003, 96, 304–314 CrossRef CAS.
- M. Runowski, N. Stopikowska, D. Szeremeta, S. Goderski, M. Skwierczyńska and S. Lis, ACS Appl. Mater. Interfaces, 2019, 11, 13389–13396 CrossRef CAS PubMed.
- M. Skwierczyńska, N. Stopikowska, P. Kulpiński, M. Kłonowska, S. Lis and M. Runowski, Nanomaterials, 2022, 12, 1926 CrossRef PubMed.
- T. Zheng, M. Runowski, P. Woźny, B. Barszcz, S. Lis, M. Vega, J. Llanos, K. Soler-Carracedo and I. R. Martín, J. Alloys Compd., 2022, 906, 164329 CrossRef CAS.
- N. Rakov and G. S. Maciel, Sens. Actuators, B, 2012, 164, 96–100 CrossRef CAS.
- M. L. Debasu, D. Ananias, I. Pastoriza-Santos, L. M. Liz-Marzán, J. Rocha and L. D. Carlos, Adv. Mater., 2013, 25, 4868–4874 CrossRef CAS PubMed.
- V. Kumar, S. Som, S. Dutta, S. Das and H. C. Swart, RSC Adv., 2016, 6, 84914–84925 RSC.
- H. Lv, P. Du, L. Luo and W. Li, Mater. Adv., 2021, 2, 2642–2648 RSC.
- J. W. Gregory, H. Sakaue, T. Liu and J. P. Sullivan, Annu. Rev. Fluid Mech., 2014, 46, 303–330 CrossRef.
|
This journal is © The Royal Society of Chemistry 2023 |
Click here to see how this site uses Cookies. View our privacy policy here.