DOI:
10.1039/D3TC01199B
(Paper)
J. Mater. Chem. C, 2023,
11, 8929-8934
Tuning the rare-earth UiO-66 metal–organic framework platform for white light emission†
Received
5th April 2023
, Accepted 6th June 2023
First published on 6th June 2023
Abstract
Metal–organic frameworks (MOFs) have received notable attention owing to their structural diversity, permanent porosity, and high surface areas. In addition to these properties, MOFs made of rare-earth (RE) metals have the potential to be used in photoluminescent applications, where photoluminescence is dictated by the identity of the metal ion and organic linker that are used to construct the RE-MOF. In this study, we investigate the photoluminescent properties of RE-UiO-66 by synthesizing and characterizing mono-, bi-, and tri-metallic analogues of RE-UiO-66 (RE = Tb(III), Eu(III), and Gd(III)), with the aim of designing a MOF with white light emitting properties. Additionally, as a proof of concept, the new Tb
:
Gd
:
Eu-UiO-66 MOF is used as a surface coating on a UV light emitting diode (LED) to give a white light emitting device where photostability of the MOF is tested.
Introduction
The development of energy efficient lighting technologies is important for reducing global electricity consumption, and thus greenhouse gas emissions.1 As such, the replacement of traditional, low energy efficiency lighting sources such as incandescent and fluorescent lamps, is required for mitigating the global energy crisis.2 As one of the most promising solutions, light emitting diodes (LEDs) have gained interest due to their relatively long lifespan,3 high energy efficiency,4,5 and accessibility to a wide range of colours and temperatures.6 By tuning the colour and temperature of specific phosphors, white LEDs (WLEDs) can be produced and used for applications in solid state lighting and displays.4,7
Currently, there are three popular strategies for the production of WLEDs; (i) utilizing multiple LED chips, where each chip emits red, green, and blue (RGB) light that is mixed to give white,4 (ii) coating UV-LED chips with phosphors that absorb UV light and emit triple wavelength RGB,8,9 (iii) coating blue LED chips with phosphors that absorb a portion of blue light, and emit green and red.4,10 When designing a material for approach ii, the Commission International de l’Eclairage (CIE) chromaticity diagram is often used to determine and compare the colour purity of the RGB emitting phosphor.11 Two coordinates from the visible region are calculated, where pure white is defined as x, y = 0.33, 0.33.
Significant effort has gone into the development of new white light (RGB) emitting materials including the study of organic molecules or polymers,12,13 metal-doped materials,14,15 metal complexes,16 and nanomaterials as phosphors.17,18 In the solid-state, pure organic molecules and polymers are prone to luminescence quenching, posing a challenge for long term lighting applications.19 On the other hand, inorganic phosphors have higher efficiencies and longer lifetimes relative to organic phosphors, but often suffer from challenges related to solution processability.20
Metal–organic frameworks (MOFs) are a class of coordination polymers that are comprised of inorganic metal nodes and organic linkers, forming 2- or 3-dimensional network structures.21–26 MOFs have the potential to combine the advantageous properties of both traditional organic and inorganic phosphors. Currently, only a handful of white light emitting MOFs have been reported in the literature,27–34 with some emitting from the organic component,27 inorganic component,35 both metal and organic components,28–30,36 and others requiring doping with coordination compounds,31 perovskites,32 or rare-earth (RE) elements.37 MOFs comprised of RE elements commonly have trivalent RE ions as part of their metal node, with the nodes separated from each other by organic linkers, preventing self-quenching and photobleaching,38,39 and thus making RE-MOFs interesting candidates for WLEDs.40 In particular, RE-MOFs comprised of multinuclear cluster nodes41 allow for the incorporation of multiple RE ions at high concentrations, but with ordered separation, giving rise to a high degree of colour tunability in these materials.
The archetypal MOF, Zr-UiO-66, has been reported in numerous studies for its robust structure,42,43 high surface area,44,45 and porosity.46 More recently, we reported RE analogues of UiO-66 comprised of RE6−cluster nodes bridged by 1,4-benzenedicarboxylate (BDC2−) linkers (Fig. 1).47 Given that Eu(III) and Tb(III) ions emit red and green light, respectively,48,49 and BDC2− emits in the blue region,50 we hypothesized that the RE-UiO-66 platform would be ideal for the design of a white light (RGB) emitting MOF. In addition to emitting blue light, the BDC2− linker in RE-UiO-66 plays a key role in the sensitization of the RE metals via the antenna effect.51–56 Herein, we evaluate the photoluminescent behaviour of mixed metal RE-UiO-66 analogues (RE = Tb(III), Eu(III), and Gd(III)) by tuning the ratio of RE ions to give bi- and tri-metal RE6−nodes, ultimately leading to the formation of a white light emitting MOF.
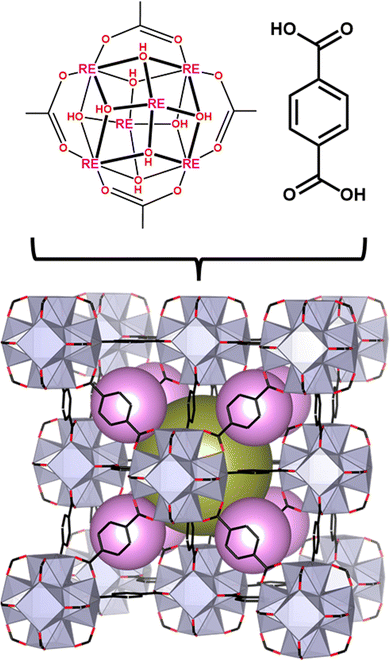 |
| Fig. 1 Structure of RE-UiO-66 depicting the RE6 cluster with the H2BDC linker forming the fcu net with the tetrahedral cage (pink spheres) and octahedral cage (gold sphere). | |
Results and discussion
RE-UiO-66 analogues with mono- (Tb(III), Gd(III), Eu(III)), bi-(Gd
:
Eu, Tb
:
Gd, Tb
:
Eu) and tri-metal (Tb
:
Gd
:
Eu) nodes are synthesized using a RE(NO3)3·xH2O precursor (see Table S1 for details, ESI†) with the ditopic linker H2BDC in the presence of a fluorinated modulator, 2,6-difluorobenzoic acid (2,6-DFBA), in N,N-dimethylacetamide (DMA). In order to confirm the bulk crystallinity and phase purity of all mono-, bi-, and tri-metal RE-UiO-66 analogues, powder X-ray diffraction (PXRD) data were collected. As can be observed in Fig. 2a and Fig. S1 (ESI†), all reflections are consistent with the expected fcu topology of RE-UiO-66. N2 adsorption–desorption analysis of bi- and tri-metal RE-UiO-66 show reversible Type I isotherms (Fig. 2b and Fig. S2, ESI†), as expected for RE-UiO-66, with an experimental Brunauer–Emmett–Teller (BET) surface area of 930 m2 g−1 for the tri-metal Tb
:
Gd
:
Eu-UiO-66 analogue (Fig. 2b). Diffuse reflectance infrared Fourier transform spectroscopy (DRIFTS) of Tb
:
Gd
:
Eu-UiO-66 confirms the presence of some μ3-OH and/or terminal –OH groups in the RE6-cluster nodes (Fig. S3, ESI†), however it should be noted that there is the possibility for μ3-OH and μ3-F ligands due to the use of a fluorinated modulator during the MOF synthesis.57 Indeed, the 19F-NMR spectrum (Fig. S4, ESI†) of a digested sample of Tb
:
Gd
:
Eu-UiO-66 displays a peak at approximately −165 ppm. This peak is assigned to HF and has been observed in other cluster-based RE-MOFs containing μ3-F bridges.58 Scanning electron microscopy (SEM) micrographs reveal the expected octahedral morphology of Tb
:
Gd
:
Eu-UiO-66 with an average crystallite size of 21 μm (Fig. 3). Additionally, energy dispersive X-ray spectroscopy (EDS) shows a homogeneous distribution of Tb(III), Gd(III), and Eu(III) ions (Fig. 3, Fig. S5, ESI†), suggesting that all three RE ions are incorporated in the individual crystallites of the MOF. After several attempts at producing a white light emitting MOF, through systematically varying the ratio of Tb
:
Gd
:
Eu, the ideal ratio was found to be 1.5
:
4.1
:
0.4. The incorporation of RE ions in RE-UiO-66 with this molar ratio is confirmed by inductively coupled plasma-mass spectrometry (ICP-MS) (see Table S2 for ICP-MS data on all bi- and tri-metal analogues, ESI†). Finally, to unequivocally confirm the presence of the three RE(III) ions in the tri-metal RE-UiO-66 crystallites, single crystals of the tri-metal RE-UiO-66 suitable for single crystal X-ray diffraction (SCXRD) were grown and the X-ray diffraction data collected. As can be seen in Fig. S6 (ESI†), upon UV light irradiation, the MOF crystals emit a cool white colour. When the same ratio of Tb
:
Gd
:
Eu obtained by ICP-MS was used to solve the crystal structure of tri-metal RE-UiO-66, adequate wR2 values were obtained (Table S3, ESI†). As such, the combination of white light emission observed from the single crystals (Fig. S5, ESI†) and the crystal structure solution showing acceptable wR2 values when solved with Tb(III), Gd(III), and Eu(III), further confirms the presence of the three RE(III) ions and the expected ratio of 1.5
:
4.1
:
0.4 for Tb
:
Gd
:
Eu.
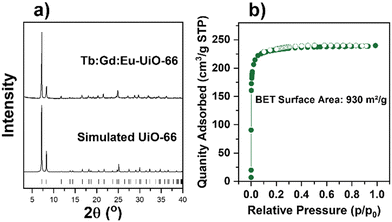 |
| Fig. 2 (a) PXRD pattern of synthesized Tb : Gd : Eu-UiO-66 and the simulated pattern, and (b) nitrogen sorption isotherm of Tb : Gd : Eu-UiO-66. | |
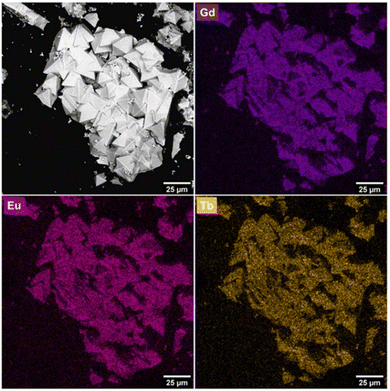 |
| Fig. 3 SEM micrographs and EDS mapping of Gd:Eu:Tb-UiO-66. | |
Eu(III) and Tb(III) ions emit from their 5D0 and 5D4 states, which have energies of 17
250 and 20
500 cm−1, respectively.59 Tb(III) emission can be sensitized via the antenna effect through energy transfer from the triplet excited state (T1) of an organic ligand to its 5D4 state (20
500 cm−1), whereas Eu(III) emission can be sensitized through energy transfer to its 5D0 state (17
250 cm−1), or higher lying 5DJ states (5D1 = 19
000 cm−1, and 5D2 = 21
450 cm−1).60 As such, sensitization of both Tb(III) and Eu(III) via the antenna effect requires a linker with T1 energy of at least 22
350 cm−1 according to Latva's rule.59,60 If the T1 energy of the linker is too close to the acceptor state of the lanthanoid, energy back-transfer to the linker can occur, leading to quenching of the lanthanoid emission.60 Alternatively, if the T1 energy of the linker is too high (>26
000 cm−1), there will be inefficient energy transfer to the lanthanoid.61,62 BDC2− has a T1 energy of 25
641 cm−1,50 which is sufficient for sensitizing both Eu(III) and Tb(III) emission. Given that Eu(III) and Tb(III) ions may be present in the same RE6-cluster of RE-UiO-66, and therefore 3.92 Å apart, energy transfer from Tb(III) to Eu(III) is also possible.63 Tb(III) ions give rise to green emission with sharp emission bands at 489, 544, 582 and 617 nm, 5D4 → 7F6,5,4,3 as can be seen in Tb-UiO-66 (Fig. S7a, ESI†). Eu(III) ions give rise to red emission with sharp emission bands at 590, 615, 655, 696 nm, 5D0 → 7F1,2,3,4 as can be observed in Eu-UiO-66 (Fig. S7b, ESI†). Eu-UiO-66 also exhibits a broad blue emission with moderate intensity spanning 350–500 nm corresponding to the singlet state (S1) emission of the BDC2− linker and suggesting that energy transfer from the BDC2− linker to Eu(III) does not occur with 100% efficiency.
In order to increase the contribution of the blue linker emission in RE-UiO-66 to generate a white light emitting MOF, a RE(III) ion with high energy 4f excited states is required to further supress the linker T1 to RE(III) energy transfer pathway in RE-UiO-66. Given that Gd(III) has very high energy 4f excited states (>32
000 cm−1), strong BDC2− linker emission is observed in Gd-UiO-66, giving rise to a broad band centered at 425 nm in the blue (Fig. S7c, ESI†). The excited state energy, energy transfer pathways, and emissive states in RE-UiO-66 analogues can be visualized in Fig. 4. The BDC2− linker acts as the light harvester, with absorbance spanning 200–400 nm (Fig. S8a, ESI†). When excited into this absorption band, the excited singlet state of the linker is generated. Owing to the presence of heavy RE(III) ions, intersystem crossing (ISC) occurs and gives rise to the T1 state of BDC2−. This is followed by non-radiative energy transfer, or the antenna effect, from the BDC2− T1 state to the 4f excited states of RE(III) ions. Owing to the energy of the T1 state of BDC2−, energy transfer to both Eu(III) and Tb(III) is possible, whereas energy transfer to the 4f states of Gd(III) is not possible.
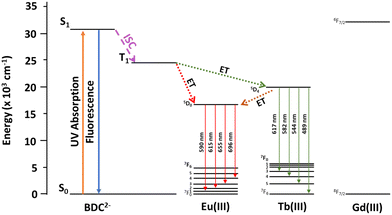 |
| Fig. 4 Jablonski diagram depicting excited state energy levels and the possible energy transfer pathways for the BDC2− linker and Eu(III), Tb(III), and Gd(III). | |
Working towards a white light emitting (RGB) RE-UiO-66 analogue, bi-metal analogues of the MOF were first studied to gain information about the antenna effect in mixed-metal RE-UiO-66. In Gd:Eu-UiO-66 (Fig. S9a, ESI†), the blue emission (λem = 350–500 nm) from the linker is more intense than that observed for Eu-UiO-66 (Fig. S7b, ESI†), relative to the Eu(III) emission. This demonstrates that the presence of Gd(III) leads to less efficient energy transfer from the BDC2− linker T1 state to the Eu(III) 5D0, 5D1 and 5D2 states, in comparison to that observed in Eu-UiO-66. Tb:Gd-UiO-66 (Fig. S9b, ESI†) on the other hand, exhibits the same photoluminescence emission spectrum as Tb-UiO-66 (Fig. S7a, ESI†), meaning that the presence of Gd(III) does not significantly alter the efficiency of energy transfer from the BDC2− linker T1 state to the Tb(III) 5D4 state. The photoluminescence emission spectrum of Tb:Eu-UiO-66 (Fig. S9c, ESI†) has a weak blue emission (λem = 350–500 nm) from the linker, in contrast to Tb-UiO-66 and Tb:Gd-UiO-66, which suggests that the addition of Eu(III) decreases the efficiency of energy transfer from the BDC2− linker T1 state to the Tb(III) 5D4 state, or where it's possible that energy transfer from Tb(III) to Eu(III) might be playing a role. Both Eu(III) and Tb(III) transitions are observed in the emission spectrum of Tb:Eu-UiO-66 with an overlap of the 5D4 → 7F3 transition of Tb(III) and 5D0 → 7F2 transition of Eu(III), hence displaying emission in the blue, green, and red regions.
Given that green, blue, and red emission is observed in Tb-UiO-66, Gd-UiO-66, and Eu-UiO-66, respectively, as well as in the bi-metal RE-UiO-66 analogues, we reasoned that a judicious mixture of Tb
:
Gd
:
Eu should give rise to white light emission. Indeed, the tri-metal RE-UiO-66 analogues composed of Tb(III), Gd(III), and Eu(III) (Tb
:
Gd
:
Eu-UiO-66), demonstrate strong blue emission from the linker, narrow green emission from Tb(III), and narrow red emission from Eu(III) (Fig. 5a) when excited into the BDC2− linker at 312 nm (Fig. S8 and S10, ESI†). Tuning the composition of Tb
:
Gd
:
Eu to 1.5
:
4.1
:
0.4 in the hexanuclear cluster gives a distribution of green, blue, and red emission simultaneously, giving rise to white-light emission with the CIE 1931 color coordinate of x = 0.3103, y = 0.3901, corresponding to a cool white colour and correlated colour temperature (CCT) of 6287 K (Fig. 5b). Tb
:
Gd
:
Eu-UiO-66 shows a quantum yield of 11.4% (Fig. S11, ESI†), similar to that observed for other photoluminescent and white light emitting RE-MOFs.40,64 Additionally, the trimetallic RE-MOF displays average lifetimes of 22.5 (478 nm), 34.9 (543 nm), and 16.2 μs (613 nm), for the 5D4 → 7F6 emission from Tb(III) ions, 5D4 → 7F5 emission from Tb(III) ions, and overlapping 5D4 → 7F3 emission from Tb(III) ions with 5D0 → 7F2 emission from Eu(III) ions, respectively (Fig. S12 and Table S4, ESI†). When compared to the single-metal Tb-UiO-66, which has an emission lifetime on the order of 1000 μs (5D4 → 7F5)56 the trimetallic Tb
:
Gd
:
Eu-UiO-66 displays a lower average lifetime, which suggests energy transfer from Tb(III) to Eu(III) is occurring in the trimetallic MOF.63,65
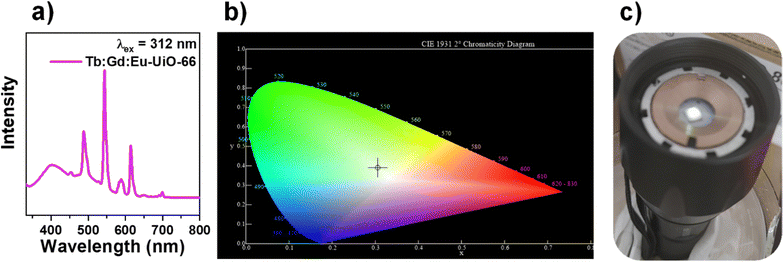 |
| Fig. 5 (a) Photoluminescence emission spectrum of Tb : Gd : Eu-UiO-66, (b) the CIE diagram and (c) Tb : Gd : Eu-UiO-66 on the LED flashlight with UV-LED chip (λ = 310 nm). | |
To demonstrate a proof of concept white light emitting device incorporating Tb
:
Gd
:
Eu-UiO-66, the MOF was coated onto a UV-LED chip (λ = 310 nm) where the white light emission can be visually observed (Fig. 5c). To test the photostability of Tb
:
Gd
:
Eu-UiO-66 under ambient conditions, the MOF was irradiated at 312 nm and emission spectra were collected at 0, 1, 2, 3, 4, 5, and 6 hours (Fig. 6 and Fig. S13, ESI†). Although there are some minor intensity differences observed, in all cases, the blue, green, and red emission bands are maintained, demonstrating that the MOF continues to emit white light for hours under ambient conditions.
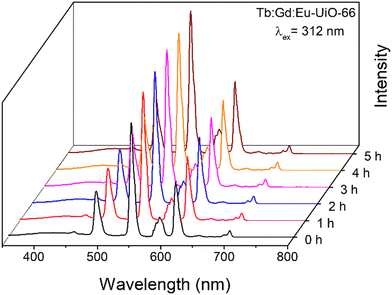 |
| Fig. 6 Photoluminescence emission spectrum of Tb : Gd : Eu-UiO-66 as a function of time under ambient conditions, irradiated at 312 nm. | |
Conclusions
In conclusion, the photoluminescent behaviour of RE-UiO-66 has been tuned through the generation of bi-metal and tri-metal Tb
:
Gd
:
Eu analogues, ultimately leading to the formation of a white light emitting MOF. The structure and phase purity of all MOFs is confirmed by PXRD and nitrogen adsorption–desorption analysis with the materials demonstrating high surface area and porosity. The elemental composition of the bi- and tri-metal analogues is determined by ICP-MS while SEM-EDS confirms that the Tb(III), Gd(III), and Eu(III) ions are evenly distributed throughout the MOF crystallites. A ternary system (Tb
:
Gd
:
Eu-UiO-66) was found to be necessary for the generation of a white light emitting MOF and single crystals of the tri-metal MOF are observed to emit white light, further confirming that all 3 metal ions are present in individual crystallites. Finally, as a proof of concept, Tb
:
Gd
:
Eu-UiO-66 was applied as a surface coating on a UV-LED, giving rise to a device that emits a cool white colour, with no significant photobleaching or photodegradation observed within 6 hours.
Experimental
Synthetic procedures
RE-UiO-66 was synthesized through a solvothermal synthesis where a suspension of RE(NO3)3·xH2O (see Table S2 for details, ESI†), terephthalic acid (0.171 mmol, 28.5 mg) and 2,6-difluorobenzoic acid (2.78 mmol, 440 mg) was prepared in 8 mL of DMA in a 6-dram vial. The solution was sonicated until all reagents dissolved and was placed in a 120 °C oven for 72 hours. Once out of the oven, the solution was cooled down to room temperature before centrifugation and subsequent removal of the DMA reaction solvent. The solid material was then washed with fresh DMF (3 × 5 mL) over the course of 24 hours and later with fresh acetone (3 × 5 mL) over the course of 2 days. The material was set to dry in a vacuum oven at 80 °C for 1 hour and was then activated at 120 °C for 20 hours using a Micromeritics Smart VacPrep instrument.
Single crystals of Tb
:
Gd
:
Eu-UiO-66 were synthesized through a solvothermal procedure. For that, 0.0798 (35.6 mg), 0.2366 (104 mg), and 0.0246 mmol (10.8 mg) of Tb(NO3)3·xH2O, Gd(NO3)3·xH2O, and Eu(NO3)3·xH2O, respectively, were added to 25 mL of DMA, followed by the addition of formic acid (15 mL), terephthalic acid (0.42 mmol, 70 mg) and 2,6-difluorobenzoic acid (5.69 mmol, 900 mg). After that, the suspension was sonicated until all solids were dissolved and aliquots of 4 mL were distributed in 6-dram vials. The vials were then sealed and placed into a preheated oven at 140 °C for 96 h.
Characterization and photoluminescence data information can be found in the ESI.†
Author contributions
Zvart Ajoyan – conceptualization, methodology, investigation, validation, visualization, writing – original draft; Hudson A. Bicalho – investigation, validation, visualization, project administration, writing – original draft; P. Rafael Donnarumma – conceptualization, investigation, validation; Artsiom Antanovich – investigation, validation; Ashlee J. Howarth – conceptualization, funding acquisition, project administration, resources, supervision, writing – review & editing.
Conflicts of interest
There are no conflicts to declare.
Acknowledgements
The authors thank Prof. Tomislav Friščić for access to X-ray facilities and Dr Vladimir Lesnyak for access and assisting photoluminescence measurements. We acknowledge the support of the Natural Sciences and Engineering Research Council of Canada (NSERC) [funding reference number: RGPIN-2018-04388 (A. J. H.)]. Cette recherche a été financée par le Conseil de recherches en sciences naturelles et en génie du Canada (CRSNG) [numéro de reference: RGPIN-2018-04388 (A. J. H.)]. All structural figures were made using VESTA 3.66
Notes and references
- R. Pode, Renewable Sustainable Energy Rev., 2020, 133, 110043 CrossRef
.
- D. F. de Souza, P. P. F. da Silva, L. F. A. Fontenele, G. D. Barbosa and M. de Oliveira Jesus, Energy Rep., 2019, 5, 409–424 CrossRef
.
- A. Bergh, G. Craford, A. Duggal and R. Haitz, Phys. Today, 2001, 54, 42–47 CrossRef CAS
.
- J. Cho, J. H. Park, J. K. Kim and E. F. Schubert, Laser Photonics Rev., 2017, 11, 1600147 CrossRef
.
- T. Taguchi, J. Light Visual Environ., 2003, 27, 131–139 CrossRef
.
- P. Pust, P. J. Schmidt and W. Schnick, Nat. Mater., 2015, 14, 454–458 CrossRef CAS PubMed
.
- J. Chen, S. Mukherjee, W. Li, H. Zeng and R. A. Fischer, Nat. Rev. Mater., 2022, 7, 677–678 CrossRef
.
-
A. Mills, III-Vs rev., 2005, 18, 32–34 Search PubMed
.
-
S. Tabuchi, Japanese Utility Model Patent Application, Publication No. S50-79379, 1973 Search PubMed
.
- Y. Shimizu, Japanese Patent Application Search PubMed
, Publication No. H08-7614, 1996.
- C. S. McCamy, Color Res. Appl., 1992, 17, 142–144 CrossRef
.
- Y. Yang, M. Lowry, C. M. Schowalter, S. O. Fakayode, J. O. Escobedo, X. Xu, H. Zhang, T. J. Jensen, F. R. Fronczek, I. M. Warner and R. M. Strongin, J. Am. Chem. Soc., 2006, 128, 14081–14092 CrossRef CAS PubMed
.
- S. Mukherjee and P. Thilagar, Dyes Pigm., 2014, 110, 2–27 CrossRef CAS
.
- Y. Yang, L. Chen, F. Jiang, M. Yu, X. Wan, B. Zhang and M. Hong, J. Mater. Chem. C, 2017, 5, 1981–1989 RSC
.
- Y. Wang, J. Ding, Y. Wang, X. Zhou, Y. Cao, B. Ma, J. Li, X. Wang, T. Seto and Z. Zhao, J. Mater. Chem. C, 2019, 7, 1792–1820 RSC
.
- P. Coppo, M. Duati, V. N. Kozhevnikov, J. W. Hofstraat and L. De Cola, Angew. Chem., Int. Ed., 2005, 44, 1806–1810 CrossRef CAS PubMed
.
- M. J. Bowers, J. R. McBride and S. J. Rosenthal, J. Am. Chem. Soc., 2005, 127, 15378–15379 CrossRef CAS PubMed
.
- Y. Hu, X. Liang, D. Wu, B. Yu, Y. Wang, Y. Mi, Z. Cao and Z. Zhao, J. Mater. Chem. C, 2020, 8, 734–741 RSC
.
- J. Cornil, D. Beljonne, J. P. Calbert and J. L. Brédas, Adv. Mater., 2001, 13, 1053–1067 CrossRef CAS
.
- F. So, J. Kido and P. Burrows, MRS Bull., 2008, 33, 663–669 CrossRef CAS
.
- B. F. Hoskins and R. Robson, J. Am. Chem. Soc., 1990, 112, 1546–1554 CrossRef CAS
.
- H. Li, M. Eddaoudi, M. O'Keeffe and O. M. Yaghi, Nature, 1999, 402, 276–279 CrossRef CAS
.
- M. Kondo, T. Yoshitomi, H. Matsuzaka, S. Kitagawa and K. Seki, Angew. Chem., Int. Ed. Engl., 1997, 36, 1725–1727 CrossRef CAS
.
- F. Serpaggi and G. Férey, J. Mater. Chem., 1998, 8, 2737–2741 RSC
.
- O. M. Yaghi and H. Li, J. Am. Chem. Soc., 1995, 117, 10401–10402 CrossRef CAS
.
- O. M. Yaghi, G. Li and H. Li, Nature, 1995, 378, 703–706 CrossRef CAS
.
- M.-S. Wang, S.-P. Guo, Y. Li, L.-Z. Cai, J.-P. Zou, G. Xu, W.-W. Zhou, F.-K. Zheng and G.-C. Guo, J. Am. Chem. Soc., 2009, 131, 13572–13573 CrossRef CAS PubMed
.
- M.-S. Wang, G.-C. Guo, W.-T. Chen, G. Xu, W.-W. Zhou, K.-J. Wu and J.-S. Huang, Angew. Chem., Int. Ed., 2007, 46, 3909–3911 CrossRef CAS PubMed
.
- D. F. Sava, L. E. S. Rohwer, M. A. Rodriguez and T. M. Nenoff, J. Am. Chem. Soc., 2012, 134, 3983–3986 CrossRef CAS PubMed
.
- J. He, M. Zeller, A. D. Hunter and Z. Xu, J. Am. Chem. Soc., 2012, 134, 1553–1559 CrossRef CAS PubMed
.
- C.-Y. Sun, X.-L. Wang, X. Zhang, C. Qin, P. Li, Z.-M. Su, D.-X. Zhu, G.-G. Shan, K.-Z. Shao, H. Wu and J. Li, Nat. Commun., 2013, 4, 2717 CrossRef PubMed
.
- C. Peng, X. Song, J. Yin, G. Zhang and H. Fei, Angew. Chem., Int. Ed., 2019, 58, 7818–7822 CrossRef CAS PubMed
.
- L. Qiu, C. Yu, X. Wang, Y. Xie, A. M. Kirillov, W. Huang, J. Li, P. Gao, T. Wu, X. Gu, Q. Nie and D. Wu, Inorg. Chem., 2019, 58, 4524–4533 CrossRef CAS PubMed
.
- N.-C. Chiu, K. T. Smith and K. C. Stylianou, Coord. Chem. Rev., 2022, 459, 214441 CrossRef CAS
.
- S. Dang, J.-H. Zhang and Z.-M. Sun, J. Mater. Chem., 2012, 22, 8868–8873 RSC
.
- K. Liu, H. You, Y. Zheng, G. Jia, Y. Huang, M. Yang, Y. Song, L. Zhang and H. Zhang, Cryst. Growth Des., 2010, 10, 16–19 CrossRef CAS
.
- R. Peña-Rodríguez, J. A. Molina-González, H. Desirena-Enrriquez, E. Armenta-Jaime, J. M. Rivera and S. E. Castillo-Blum, J. Mater. Chem. C, 2021, 9, 15891–15899 RSC
.
- L. H. Slooff, A. Polman, S. I. Klink, L. Grave, F. C. J. M. van Veggel and J. W. Hofstraat, J. Opt. Soc. Am. B, 2001, 18, 1690–1694 CrossRef CAS
.
- H. A. Bicalho, F. Saraci, J. D. J. Velazquez-Garcia, H. M. Titi and A. J. Howarth, Chem. Commun., 2022, 58, 10925–10928 RSC
.
- Y. Wang, K. Zhang, X. Wang, X. Xin, X. Zhang, W. Fan, B. Xu, F. Dai and D. Sun, J. Mater. Chem. C, 2020, 8, 1374–1379 RSC
.
- F. Saraci, V. Quezada-Novoa, P. R. Donnarumma and A. J. Howarth, Chem. Soc. Rev., 2020, 49, 7949–7977 RSC
.
- A. Dhakshinamoorthy, A. Santiago-Portillo, A. M. Asiri and H. Garcia, ChemCatChem, 2019, 11, 899–923 CrossRef CAS
.
- A. Shaabani, R. Mohammadian, S. E. Hooshmand, A. Hashemzadeh and M. M. Amini, ChemistrySelect, 2017, 2, 11906–11911 CrossRef CAS
.
- Y. Jiao, Y. Liu, G. Zhu, J. T. Hungerford, S. Bhattacharyya, R. P. Lively, D. S. Sholl and K. S. Walton, J. Phys. Chem. C, 2017, 121, 23471–23479 CrossRef CAS
.
- S. Chavan, J. G. Vitillo, D. Gianolio, O. Zavorotynska, B. Civalleri, S. Jakobsen, M. H. Nilsen, L. Valenzano, C. Lamberti, K. P. Lillerud and S. Bordiga, Phys. Chem. Chem. Phys., 2012, 14, 1614–1626 RSC
.
- X. Yang, Z. Li and S. Tang, Cryst. Growth Des., 2021, 21, 6092–6100 CrossRef CAS
.
- P. R. Donnarumma, S. Frojmovic, P. Marino, H. A. Bicalho, H. M. Titi and A. J. Howarth, Chem. Commun., 2021, 57, 6121–6124 RSC
.
- M. H. V. Werts, R. T. F. Jukes and J. W. Verhoeven, Phys. Chem. Chem. Phys., 2002, 4, 1542–1548 RSC
.
- G. Deng, Nat. Chem., 2018, 10, 110 CrossRef CAS PubMed
.
- D. Briones, P. Leo, J. Cepeda, G. Orcajo, G. Calleja, R. Sanz, A. Rodríguez-Diéguez and F. Martínez, CrystEngComm, 2018, 20, 4793–4803 RSC
.
- T. Tachikawa, J. R. Choi, M. Fujitsuka and T. Majima, J. Phys. Chem. C, 2008, 112, 14090–14101 CrossRef CAS
.
- S. Bordiga, C. Lamberti, G. Ricchiardi, L. Regli, F. Bonino, A. Damin, K. P. Lillerud, M. Bjorgen and A. Zecchina, Chem. Commun., 2004, 2300–2301 RSC
.
- J.-K. Cheng, P.-X. Yin, Z.-J. Li, Y.-Y. Qin and Y.-G. Yao, Inorg. Chem. Commun., 2007, 10, 808–810 CrossRef CAS
.
- W. Chen, J.-Y. Wang, C. Chen, Q. Yue, H.-M. Yuan, J.-S. Chen and S.-N. Wang, Inorg. Chem., 2003, 42, 944–946 CrossRef CAS PubMed
.
- H.-Q. Yin, X.-Y. Wang and X.-B. Yin, J. Am. Chem. Soc., 2019, 141, 15166–15173 CrossRef CAS PubMed
.
- Z. Ajoyan, G. A. Mandl, P. R. Donnarumma, V. Quezada-Novoa, H. A. Bicalho, H. M. Titi, J. A. Capobianco and A. J. Howarth, ACS Mater. Lett., 2022, 1025–1031 CrossRef CAS
.
- J. P. Vizuet, M. L. Mortensen, A. L. Lewis, M. A. Wunch, H. R. Firouzi, G. T. McCandless and K. J. Balkus, J. Am. Chem. Soc., 2021, 143, 17995–18000 CrossRef CAS PubMed
.
- X. Song, Q. Huang, J. Liu, H. Xie, K. B. Idrees, S. Hou, L. Yu, X. Wang, F. Liu, Z. Qiao, H. Wang, Y. Chen, Z. Li and O. K. Farha, ACS Appl. Mater. Interfaces, 2023, 15, 18229–18235 CrossRef CAS PubMed
.
- D. Parker, Coord. Chem. Rev., 2000, 205, 109–130 CrossRef CAS
.
- M. Latva, H. Takalo, V.-M. Mukkala, C. Matachescu, J. C. Rodríguez-Ubis and J. Kankare, J. Lumin., 1997, 75, 149–169 CrossRef CAS
.
- Y. Zhang, W. Thor, K.-L. Wong and P. A. Tanner, J. Phys. Chem. C, 2021, 125, 7022–7033 CrossRef CAS
.
- M. W. Mara, D. S. Tatum, A.-M. March, G. Doumy, E. G. Moore and K. N. Raymond, J. Am. Chem. Soc., 2019, 141, 11071–11081 CrossRef CAS PubMed
.
- A. N. Carneiro Neto, R. T. Moura, A. Shyichuk, V. Paterlini, F. Piccinelli, M. Bettinelli and O. L. Malta, J. Phys. Chem. C, 2020, 124, 10105–10116 CrossRef CAS
.
- H. Li, H.-B. Liu, X.-M. Tao, J. Su, P.-F. Ning, X.-F. Xu, Y. Zhou, W. Gu and X. Liu, Dalton Trans., 2018, 47, 8427–8433 RSC
.
- I. Carrasco, F. Piccinelli and M. Bettinelli, J. Lumin., 2017, 189, 71–77 CrossRef CAS
.
- K. Momma and F. Izumi, J. Appl. Crystallogr., 2011, 44, 1272–1276 CrossRef CAS
.
Footnotes |
† Electronic supplementary information (ESI) available. CCDC 2265674. For ESI and crystallographic data in CIF or other electronic format see DOI: https://doi.org/10.1039/d3tc01199b |
‡ Z. A. and H. A. B. contributed equally to this work |
|
This journal is © The Royal Society of Chemistry 2023 |
Click here to see how this site uses Cookies. View our privacy policy here.