DOI:
10.1039/D3TC00215B
(Paper)
J. Mater. Chem. C, 2023,
11, 4094-4103
An efficient pink luminescent Eu(III) coordination polymer excited on a blue LED chip†
Received
18th January 2023
, Accepted 20th February 2023
First published on 9th March 2023
Abstract
Two new sets of lanthanide-based coordination polymers (CPs), [Ln(DHTP)(NO3)(H2O)4]n [Ln1 = Gd1, Eu1] and [Eu2(DHTP)(TTA)4(DMF)2(MeOH)2]n (Eu2) using 2,5-dihydroxyterephthalic acid (DHTP) and incorporating thenoyltrifluoroacetone (TTA) units, have been synthesized via condensation reactions and characterized. The single-crystal X-ray diffraction studies revealed that the Eu1 CP has a 2D zig–zag chain while the Eu2 CP exhibits a 2D polymeric structure derived from dinuclear Eu(III) building blocks. A detailed photoluminescence study proved that the band structure of the DHTP ligand is suitable for the luminescence sensitization of Eu(III). Efficient red emission is observed for the Eu(III) polymers in the solid state with a fairly large luminescence intrinsic quantum yield (QEu) of 52% from the Eu2 CP. Finally, a light emitting diode (LED) was fabricated by using a resin of 10% Eu2 CP-silicon oil as the emitting layer over an InGaN blue LED chip. Interestingly, the Eu2/InGaN LED displays pink light with CIE 1931 chromaticity coordinates of (0.437, 0.185).
1. Introduction
Coordination polymer (CP) synthesis continues to stimulate research interests worldwide due to their potential for applications in a range of fields.1 Among the plethora of metal ions, trivalent lanthanide [Ln(III)] ions remain at the forefront to synthesize lanthanide coordination polymers (LnCPs) due to their structural diversity and intriguing unique photophysical properties.2–5 These properties include, but are not limited to, highly monochromatic photoluminescence (PL) (full width at half maxima (FWHM) = less than 10 nm), a large Stokes shift of the absorbed and emitted radiation, high PL quantum yield and a long luminescence lifetime (millisecond to microsecond). Moreover, a special property is the fingerprint PL (red PL for Eu(III), green PL for Tb(III), orange to deep red for Sm(III) etc.…) that covers the entire visible spectrum.6 Thus, manipulation of PL colour could be achieved by just changing the Ln(III) ions without the use of expensive synthetic chemistry, which is needed when a transition metal ion is chosen. The LnCPs are designed and synthesised by self-assembly of a suitable organic “linker” with the Ln(III) metal ion into multi-dimensional solids.7 In addition to their fascinating optical properties, LnCPs possess good chemical and thermal stability8 with improved mechanical strength.9 Because of these features, several LnCPs have been used in a range of applications such as sensors10 and full-colour and white LEDs.11
Within the class of LnCPs, the Eu(III) CPs have attracted attention for red LEDs. Hasegawa and co-workers have developed a red phosphor excited by a blue LED.12 The synthetic manipulation of red phosphors can be readily achieved by altering the organic linker(s).13,14 First, the organic linker itself can act as an antenna, and because Eu(III) has a low-lying, first excited state of 5D0 at 17
000 cm−1, any organic linker with triplet state energy higher than 19
000 cm−1 can sensitize the Eu(III) centre efficiently and promote its emission quantum yields according to Latva’s empirical rule.15 Second, attachment of various functional groups such as hydroxyl, amino or nitro groups can alter the nature of the steric effects through variable hydrogen bonds (HBs) in the crystal lattice. This could lead to the formation of a tight 3D packed array of polymers that would suppress the non-radiative relaxation transitions about the Eu(III) centre. In addition, these packed arrays can enhance thermal stability of the polymers, making them suitable materials for LEDs.16
Ln(III) ions are hard acids and thus have a strong tendency to coordinate with hard bases. Due to the strong oxophilicity of Ln(III) ions,17 many studies have focused on the linkers containing multi-carboxyl aromatic systems.18,19 The multidentate carboxyl groups in the ligands provide abundant coordination modes with Ln(III) that lead to variable structures and luminescence properties.20 In the present work, we have utilized DHTP as a prime organic antenna moiety to design and synthesize a novel class of Eu(III) CPs of the general formula: [Eu(DHTP)(NO3)(H2O)4]n (Eu1) (Scheme 1). Long-range non-covalent interactions (NCI) such as inter- and intra-molecular HB interactions play a central role in supramolecular chemistry and molecular recognition.21,22 In the DHTP linker, there are two hydroxyl groups at 2 and 5 positions that could be involved in inter- and intra-molecular HB interactions to form 2D and 3D supramolecular structures, which is indeed the case as determined using single crystal X-ray diffraction studies (vide supra). Moreover, HB interactions could be beneficial in improving the PL properties by suppressing the non-radiative relaxation transitions.23,24 Furthermore, the supramolecular structures formed through the HB effect could trigger other NCIs such as π⋯π interactions (vide supra)25 and will certainly be helpful in improving the thermal stability, energy migration and electron transport properties.24 Moreover, the presence of water and solvent molecule(s) in the inner-coordination sphere has a detrimental effect on the PL processes due to vibronic coupling. Nevertheless, this can be overcome by replacing them with additional suitable ligand(s). One of the ligands of choice is “β-diketone” that has dominated the lanthanide coordination chemistry. We have employed TTA as an ancillary ligand to synthesize another set of Eu(III) CPs with the formula: [Eu2(DHTP)(TTA)4(DMF)2(MeOH)2]n (Eu2). The presence of TTA would result in chemically and thermally stable Eu2 CPs due to the presence of hard donor oxygen atoms with favourable triplet states, especially for Eu(III), improving the PL properties of polymers. The photophysical properties of the Eu(III) CPs were analysed in detail by means of excitation and emission time-resolved spectroscopy. The effect of the bonding ancillary ligand in the present LnCPs has been discussed in detail by correlating with the Judd–Ofelt (Ω2 and Ω4) intensity parameter, radiative (kR) and non-radiative (kNR) decay rates, and radiative lifetime (τr). Encouraged by the interesting photophysical properties, we doped the Eu2 CP onto a lnGaN blue LED device. The fabricated device displayed pink emission with CIE coordinates of x = 0.41 and y = 0.18.
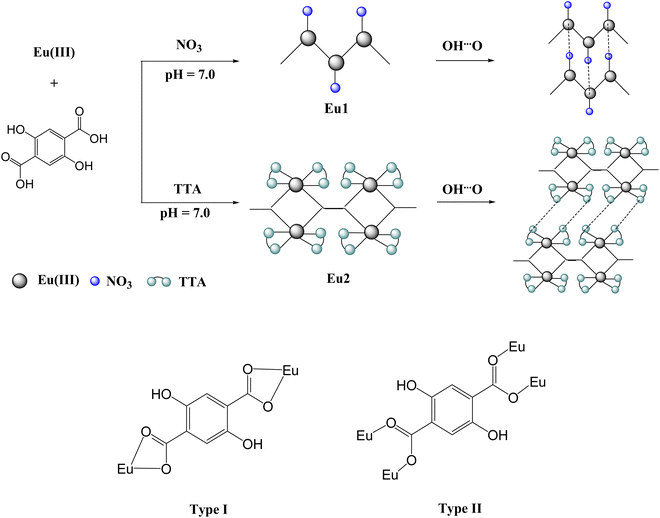 |
| Scheme 1 The synthetic routes for the coordination polymers: Eu1 and Eu2. Bottom: The different coordination modes of the DHTP ligand. | |
2. Experimental section
General details
All organic reagents, metal salts and solvents were purchased from commercial suppliers and used as received without further purification. Infrared spectra were recorded using KBr pellets on a PerkinElmer FT–IR spectrometer BX in the range of 4000–400 cm−1. Electrospray (ES) mass spectra were recorded on a VG Autospec magnetic sector instrument. Elemental analysis for carbon, hydrogen and nitrogen was performed using the PerkinElmer 2400 CHNS/O Series II Elemental Analyser.
Synthesis of Ln1 CPs (Eu1 and Gd1)
The general procedure of preparing Ln1 CPs involves reacting Ln(NO3)3·xH2O (Ln = Eu or Gd) salts with the ligands in a 1
:
1 molar ratio in the presence of MeOH/water. The mixture was refluxed for 4 hours at 100 °C. For example, DHTP (0.050 g, 0.25 mmol) was dissolved in a mixture of MeOH (10 mL) and water (30 mL) and refluxed for 30 minutes. The pH of the solution was adjusted to 7 by the addition of a few drops of dilute NH3. Then Eu(NO3)3·5H2O (0.10 g, 0.25 mmol) dissolved in 2 mL of water was added dropwise to the previous solution. The mixture was stirred at 100 °C for 4 hours and then cooled to RT. Colorless crystals formed, which were filtered and washed with MeOH. The polymers are air and moisture stable solids, and their characterization data are as follows:
[Eu(DHTP)(NO3)(H2O)4]n (Eu1)
Colorless blocks. Yield 80%. EuC8H12N1O13 (482.08) Exp (calc): %C 20.01 (19.93), %H 2.43 (2.50), %N 2.72 (2.90); ESI-MS m/z 567.2 {[Eu(DHTP)2H2O]}+, 432 {[Eu(DHTP)(NO3) H2O]}+, and 646 {[Eu(DHTP)2(NO3)(H2O)]} (Fig. S1, ESI†). FT-IR cm−1 (solid): 3345, 2960, 1590, 1506, 798.
[Gd(DHTP)2(NO3)(H2O)4]n (Gd1)
Colorless blocks. Yield is 80% of yellow powder. GdC8H12N1O13 (487.43) Exp (calc): C% 19.63 (19.69), H% 2.45 (2.48), N% 2.79 (2.86); ESI-MS m/z: 633 {[Gd(DHTP)2(NO3)(H2O)]}+, 651 {[Gd(DHTP)2(NO3)(H2O)2]}+.
Synthesis of Eu2 CP
The general procedure of preparing the Eu2 polymer involved reacting EuCl3·6H2O, DHTP and TTA in a molar ratio of 1
:
1:2 in MeOH/DMF at pH 7. For example, TTA (0.23 mmol, 0.050 g) and EuCl3·6H2O (0.040 g) were dissolved in MeOH (7 mL) and water (5 mL). The pH was adjusted to 7 by the addition of dilute NH3 solution. After stirring for 30 min at 80 °C, DHTP (0.11 mmol, 0.020 g) was added. The reaction mixture was stirred at 100 °C for 2 hours. DMF (2 mL) was added and the reaction mixture was left to cool at room temperature. Pale yellow crystals formed overnight. The solid was filtered off and washed with MeOH. The Eu2 polymer is an air and moisture stable solid, and its characterization data are as follows:
[Eu2(DHTP)(TTA)4(DMF)2(MeOH)2]n (Eu2)
Pale yellow blocks. Yield 78%. EuC24H23N1O10F6S2 (815.32) exp (calc): C% 35.39 (35.36), H% 2.77 (2.84), N% 1.79 (1.72) ESI-MS m/z: 836.25 {[Eu(DHTP)(TTA)2(DMF)-(MeOH)](H2O)}+ and 1654.25 {[Eu2(DHTP)(TTA)4(DMF)2(MeOH)2](H2O)2}+. FT-IR cm−1 (solid): 3237, 1599, 2930, 1124, 1247 and 680.
Single crystal X-ray diffraction analyses
Single crystals of Eu1 and Eu2 were obtained as detailed above and the data were collected at 150 K, using a STOE IPDS II diffractometer equipped with Mo-Kα radiation. The sample temperature was controlled using an Oxford Diffraction Cryojet apparatus. Data were integrated using the Stoe X-AREA26 software package. The numerical absorption coefficient, μ, for MoKα radiation is 7.383. The numerical absorption correction was applied using the X-RED software package.27 The data were corrected for Lorentz and Polarizing effects. The structures were solved using direct methods and subsequent difference Fourier maps and then refined on F2 by a full-matrix least-squares procedure using anisotropic displacement parameters with SHELXS-97.28 All of the hydrogen atoms were positioned geometrically in idealized positions and refined with the riding model approximation, with Uiso(H) = 1.2 or 1.5Ueq(C). All the refinements were performed using the WinGX29 suite of programs. For the molecular graphics the program Mercury package was used.30 The non-hydrogen atoms were refined anisotropically. In [Eu2(DHTP)(TTA)4(DMF)2(MeOH)2]n (Eu2), the carbon atom C22 from the TTA unit is disordered and was refined as isotropic to keep the refinement stable. Comments on the checkcif files can be found in detail in the supplementary information.
Photophysical measurements
UV-visible absorption spectra were recorded on a Varian Cary 5000 UV-visible spectrophotometer in the 250–800 nm range. Quartz cuvettes of 1 cm path length were used and solvent background corrections were applied. Steady state emission spectra and phosphorescence lifetimes (τobs) for Eu(III) f–f transitions in the solid state were recorded on an Edinburgh FS5 fluorimeter at RT. The lifetimes were measured by recording the decay at the maximum of the emission spectra. The goodness of the fits was judged by the value of the reduced chi-squared (χ2). The triplet state energy of DHTP was recorded from its Gd(III) polymer in ethanol (1 × 10−5 M) at 77 K using an NMR tube. Important photophysical parameters such as Judd–Ofelt (J–O) parameters (Ω2 and Ω4), radiative (kR) and non-radiative (kNR) decay rates, radiative lifetime (τr) and intrinsic quantum yield (QEuEu) were calculated using the following eqn (1)–(6) and details were reported elsewhere.31 | 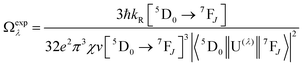 | (1) |
| 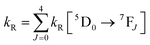 | (2) |
| 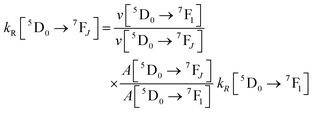 | (3) |
|  | (4) |
|  | (5) |
| 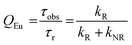 | (6) |
Fabrication of a LED package
An InGaN blue LED as a side-view type LED package (Nichia Corporation) was fabricated. A silicon resin with 10% of Eu2 CP was doped on the surface of the blue LED.
3. Results and discussion
3.1 Synthesis, characterization and thermal analysis of Eu1 CP
New Ln(III) CPs, where Ln is Eu and Gd with DHTP, have been successfully synthesized and their structures were established by single crystal X-ray diffraction, elemental analysis, infrared spectra and thermogravimetric analysis. The synthetic routes for the polymers are presented in Scheme 1. The first set of polymers, Ln1, was obtained from the reaction of Ln(III) nitrate salts with DHTP in a 1
:
1 molar ratio in aqueous media at pH 7. After a couple of hours under reflux, the polymers started to precipitate. Crystals from [Eu(DHTP)(NO3)(H2O)4]∞ (Eu1) were successfully obtained by solvent diffusion within a few minutes. Eu1 CP exhibits a 2D zig–zag chain in a 2D network. However, another set of Ln(III) CPs has been designed by the incorporation of TTA units to afford further protection for the Ln(III) centre from coordinating water molecules. EuCl3·6H2O, DHTP and TTA moieties were reacted in a molar ratio of 1
:
1
:
2 in a mixture of MeOH and DMF at pH 7. Sharp needles of the CP: [Eu2(DHTP)(TTA)4(DMF)2(MeOH)2]n (Eu2) were obtained by the slow evaporation of the reaction mixture within a few hours. The second polymer, Eu2, is based on dinuclear Eu(III) building blocks that exhibit a different 2D chain of polymers.
Infrared results
The solid-state infrared spectra (IR) of DHTP and its CPs were investigated (Fig. S2, ESI†). For DHTP, a strong stretching band of O–H attributed to the carboxylic acid and alcohol is observed at 3450 cm−1; and a carbonyl (C
O) band attributed to the acid is observed at 1638 cm−1. However, to determine the coordination mode of the carboxylate group, one can use the equation:32
Δυas–s = [υas(COO) − υs(COO)] |
where υas(COO) and υs(COO) are, respectively, the asymmetric and the symmetric stretching of C
O in the carboxylic acid. Generally, if the stretching difference Δυas–s value is less than 200 cm−1, it is believed that COO− adopts the bidentate and bridging mode. If the Δυas–s value is greater than 200 cm−1, it is believed that the COO− adopts the monodentate mode. In the IR spectra of the Ln(III) CPs, the calculated Δυas–s value is less than 200 cm−1 for the coordinating DHTP. For example, for Eu1, the asymmetric stretching vibration peak of the COO− groups is located at 1590 cm−1, while the symmetric stretching vibration absorption peak appeared at 1506 cm−1. The calculated Δυas–s value is 84 cm−1. These results confirm that the COO− groups adopt the bidentate chelating mode by bridging the two Ln(III) centres in all sets of CPs. In addition, the CPs exhibit a broad band centered at around 3300 cm−1 confirming the presence of OH units of DHTP protonated and uncoordinated to the metal centres.
Thermogravimetric analysis
The thermal stability of the Ln(III) CPs has been investigated using thermogravimetric analysis, TGA. Fig. S3 (ESI†) shows the thermogram of Eu2 recorded under an inert N2 atmosphere. The TGA curve gives a good indication of the stability of the polymer up to 170 °C. In fact, the first decomposition process occurs between 170–360 °C as the TTA units are lost with a weight loss of 51.1%, calc (52.1%). The second weight loss takes place between 360–1150 °C as the main coordination polymer [Eu(DHTP)] decomposes with a weight loss of 26.6%, calc (27.2%). Finally, the last decomposition step corresponds to the residual europium oxide with a weight loss of 22.1%, calc (21.7%). We observed similar behaviour for other Ln1 CPs.
3.2 Analysis of single crystal X-ray structures
Crystal structure of Eu1.
Crystals from [Eu(DHTP)(NO3)(H2O)4]∞ (Eu1) crystallize in the monoclinic space group P21/c. The important crystallographic data and structure refinement for all the Ln(III) complexes reported herein are listed in Table 1. X-ray diffraction studies reveal a 1D zig–zag polymer of Eu1 assembled from DHTP2− and Eu(NO3)2+ units. The asymmetric unit of Eu1 contains one Eu(III) centre coordinating to one DHTP2− ligand, one nitrate ion and 8 water molecules. Each Eu(III) centre is in a 9-coordinate environment, with four carboxylate oxygen atoms (O5, O6, O7 and O8) derived from two bridging DHTP ligands, and another four oxygen atoms (O1, O2, O3 and O4) from four aqua molecules and one oxygen atom (O9) from the nitrate ion, Fig. 1(a). The EuO9 coordination polyhedron can be described as a capped square-antiprism with approximate C2-symmetry around the metal centre. The bond distances to the Eu(III) center are comparable to those of previously published Eu(III) CPs from carboxylate units.33 The Eu–Ocarboxylate bond length is in the range of 2.480(7)–2.508(8) Å (Table 2); the Eu–Onitrate bond length is 2.535(7) Å; and the Eu–Oaqua bond length is in the range of 2.372(7)–2.422(8) Å. The angle between the two Eu(OCO) planes is 81.78° identified by atoms: C1, Eu1 and C5.
Table 1 Crystal data and structure refinement for Eu1 and Eu2 CPs
Identification code |
Eu1
|
Eu2
|
Empirical formula |
C8H12EuNO15 |
C24H20EuF6NO9S2 |
Formula weight |
514.14 |
796.49 |
Temperature |
150(2) K |
150(2) K |
Wavelength |
0.71073 Å |
0.71073 Å |
Crystal system |
Monoclinic |
Monoclinic |
Space group |
P21/c |
P21/n |
a
|
15.460(3) Å |
9.990(2) Å |
b
|
6.4600(13) Å |
19.280(4) Å |
c
|
15.470(3) Å |
15.570(3) Å |
α
|
90° |
90° |
β
|
90.10(3)° |
90.30(3)° |
γ
|
90° |
90° |
Volume |
1545.0(5) Å3 |
2998.9(10) Å3 |
Z
|
4 |
4 |
Density (calculated) |
2.210 Mg m−3 |
1.760 Mg m−3 |
Absorption coefficient |
4.144 mm−1 |
2.317 mm−1 |
F(000) |
1000 |
1560 |
Crystal size |
0.200 × 0.200 × 0.100 mm3 |
0.180 × 0.160 × 0.050 mm3 |
Theta range for data collection |
2.633 to 26.356° |
2.428 to 27.497° |
Index ranges |
−17 ≤ h ≤ 19, −8 ≤ k ≤ 8, −19 ≤ l ≤ 19 |
−12 ≤ h ≤ 12, −23 ≤ k ≤ 25, −20 ≤ l ≤ 20 |
Reflections collected |
11687 |
27796 |
Independent reflections |
3137 [R(int) = 0.1054] |
6863 [R(int) = 0.0592] |
Completeness to theta = 25.242° |
99.8% |
99.9% |
Refinement method |
Full-matrix least-squares on F2 |
Full-matrix least-squares on F2 |
Data/restraints/parameters |
3137/8/218 |
6863/209/442 |
Goodness-of-fit on F2 |
1.266 |
1.152 |
Final R indices [I > 2sigma(I)] |
R
1 = 0.06608, wR2 = 0.1594 |
R
1 = 0.0644, wR2 = 0.1502 |
R indices (all data) |
R
1 = 0.0951, wR2 = 0.1754 |
R
1 = 0.0756, wR2 = 0.1566 |
Extinction coefficient |
0.0013(4) |
0.0038(4) |
Largest diff. peak and hole |
3.826 and −2.625 e−3 |
1.631 and −1.818 e Å−3 |
CCDC deposition number |
2204044
|
2204043
|
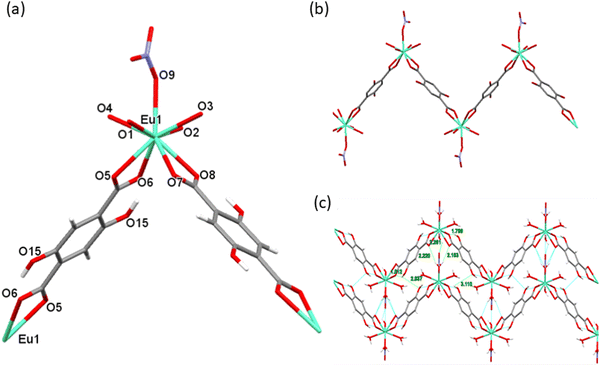 |
| Fig. 1 (a) Presentation of the coordination sphere of Eu(III) in Eu1. (b) View of the two-dimensional infinite zig–zag chain formed by Eu1 CP. (c) View of the 3D supramolecular zigzag sheets formed by Eu1 CP. | |
Table 2 Bond lengths [Å] and angles [°] for Eu1
Eu(1)–O(1) |
2.376(9) |
Eu(1)–O(6) |
2.475(9) |
Eu(1)–O(2) |
2.365(9) |
Eu(1)–O(7) |
2.512(10) |
Eu(1)–O(3) |
2.412(10) |
Eu(1)–O(8) |
2.486(9) |
Eu(1)–O(4) |
2.411(10) |
Eu(1)–O(9) |
2.536(9) |
Eu(1)–O(5) |
2.496(9) |
|
|
|
|
|
|
O(2)–Eu(1)–O(3) |
85.5(3) |
O(2)–Eu(1)–O(7) |
122.7(3) |
O(1)–Eu(1)–O(3) |
85.0(3) |
O(1)–Eu(1)–O(7) |
76.6(3) |
O(4)–Eu(1)–O(3) |
134.5(3) |
O(4)–Eu(1)–O(7) |
144.9(3) |
O(2)–Eu(1)–O(6) |
71.3(3) |
O(3)–Eu(1)–O(7) |
73.6(3) |
O(1)–Eu(1)–O(6) |
127.8(3) |
O(6)–Eu(1)–O(7) |
93.6(3) |
O(3)–Eu(1)–O(6) |
141.8(3) |
O(8)–Eu(1)–O(7) |
51.7(3) |
Interestingly, DHTP adopts the coordination mode of type I (Scheme 1) to bridge two Eu(III) centres to form an infinite 2D zig–zag chain as shown in Fig. 1b. The calculated Eu⋯Eu separation is 11.518 Å. The alcohol group (–OH) of DHTP does not participate in coordination to the Eu(III) centre, but is involved in the formation of hydrogen interactions of O15–H⋯O15′ and O16–H⋯O16′ between the alcohol groups on both sides of the adjacent zigzag chains of Eu1 to link the 2D chains into 2D supramolecular zigzag sheets (Fig. 1c). The distances of the hydrogen interactions in these contacts are 3.113 and 3.314 Å respectively. There are other O–H⋯O contacts that also play an important role in stabilizing the architecture which have been identified between the aqua ligands and the COO units. For example, the O2–H⋯O5 contact is found to be 2.772 Å. As expected, the 3D supramolecular zig–zag sheets of Eu1 are packed in an ABAB sequence along the a-axis direction.
We also synthesised a Gd1 CP with DHTP. All of the spectroscopic and analytical data confirmed the formulation of the CP: [Gd(DHTP)(NO3)(H2O)4]n (Gd1).
Crystal structure of Eu2
The crystal structure of the Eu2 CP exhibits a 2D coordination polymer derived from dinuclear Eu(III) building blocks, Fig. 2. The asymmetric unit contains one independent Eu(III) centre in an 8-coordinate environment, which is bonded to two carboxylate oxygen atoms from two bridging DHTP ligands, four oxygen atoms from two TTA units, one oxygen atom from DMF and one oxygen atom from a methanol molecule. The EuO8 coordination polyhedron has the geometry of a distorted square antiprism, with approximate C2-symmetry around the metal centre. The bond distances to the Eu(III) centre are comparable to those of known Eu(III) polymers with ligands that adopt similar coordination modes.34 As summarized in Table 3, the Eu–Ocarboxylate bonds are in the range of 2.340(6)–2.364(7) Å, Eu–OTTA bonds are in the range of 2.302(7)–2.368(7) Å; and the Eu–ODMF bond length is 2.397(7) Å. The angle between the two Tb(OCO) planes is 79.90°.
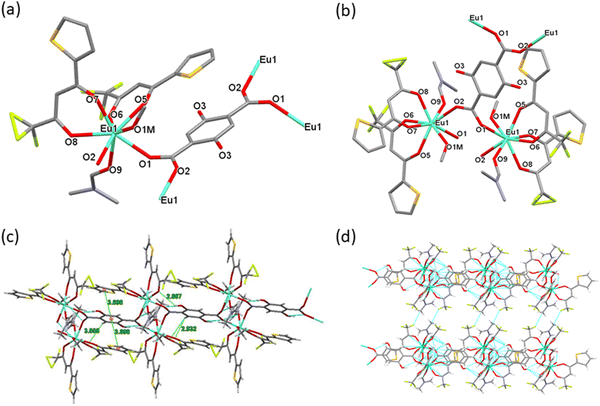 |
| Fig. 2 (a) The asymmetric unit of Eu2. (b) Presentation of the dinuclear Eu(III) building blocks in Eu2. (c) View of the Eu2 polymer. (d) View of the 3D supramolecular sheets formed by the Eu2 CP. | |
Table 3 Bond lengths [Å] and angles [°] for Eu2a
Symmetry transformations used to generate equivalent atoms: #1 −x + 1, −y + 1, −z + 1 and #2 −x + 2, −y + 1, −z + 1.
|
Eu(1)–O(1) |
2.339(5) |
Eu(1)–O(6) |
2.304(6) |
Eu(1)–O(2) |
2.362(5) |
Eu(1)–O(7) |
2.325(6) |
Eu(1)–O(1M) |
2.445(6) |
Eu(1)–O(8) |
2.370(6) |
Eu(1)–O(5) |
2.365(6) |
Eu(1)–O(9) |
2.392(6) |
|
|
|
|
O(6)–Eu(1)–O(7) |
87.6(2) |
O(7)–Eu(1)–O(8) |
72.1(2) |
O(6)–Eu(1)–O(1) |
106.3(2) |
O(1)–Eu(1)–O(8) |
140.8(2) |
O(7)–Eu(1)–O(1) |
146.1(2) |
O(2)#1–Eu(1)–O(8) |
73.1(2) |
O(6)–Eu(1)–O(2)#1 |
142.7(2) |
O(5)–Eu(1)–O(8) |
135.1(2) |
O(7)–Eu(1)–O(2)#1 |
100.6(2) |
O(6)–Eu(1)–O(9) |
76.1(2) |
O(1)–Eu(1)–O(2)#1 |
86.9(2) |
O(7)–Eu(1)–O(9) |
145.9(2) |
Compared with Eu1, DHTP adopts a different coordination mode of type II to connect the Eu(III) centers (Scheme 1). Each building block contains two Eu(III) centres linked together by one carboxylate unit from one side of DHTP (Fig. 2b). So, within the di-nuclear building block, the Eu⋯Eu separation is 5.224 Å. The adjacent building blocks connect to each other by one carboxylate unit from the other side of DHTP resulting in the formation of a two-dimensional polymer as shown in Fig. 2c. The polymer exhibits π⋯π stacking interactions of 3.698 Å between the DHTP and the thenoyl rings that are responsible for the stability of the polymer. The Eu⋯Eu separation between two successive building blocks is 12.045 Å. In addition, each building block exhibits variable H-bonding interactions of OH⋯O(TTA) (2.667 Å) and (DHTP) CH⋯O(TTA) (2.932 and 3.558 Å). Finally, the parallel chains of polymers are packed together through CH⋯F contacts that results in the formation of 3D supramolecular sheets, Fig. 2d.
4. Photophysical properties
The UV/Vis absorption spectrum of the free DHTP was determined in dilute tetrahydrofuran solution (10−5 M) and is shown in Fig. S4 (ESI†). The spectrum displayed a strong π–π* transition λabsmax = 374 nm with a molar absorption coefficient (ε) of 26
000 M−1 cm−1 implying that the organic linker has a good light absorbing capability. The Ln CPs are not soluble in common solvents and their absorption spectra are not recorded in solution.
Apart from the light absorbing capability, an important parameter of efficiently emitting lanthanide material is energy matching between the triplet band energy (T1) of the chelating organic ligand and the emitting state of the Ln(III) ion in question. Generally, in CPs, the organic linker DHTP would most likely behave as an aggregated organic compound with band-network structures rather than a single molecule.35 Upon coordination to the Ln(III) centre, the triplet state energy of the band is responsible for the sensitization process. Thus, the triplet band energy (T1) of DHTP can be determined experimentally from the phosphorescence spectrum of its iso-structural Gd(III) CP recorded in ethanol at 77 K. Generally, Gd(III) complexes are optimal for this purpose due to the large probability of the phosphorescence because of the combination of both paramagnetic36 and heavy atom effects. Moreover, 6P7/2 of Gd(III) lies at 32
000 cm−1, which is too high to be populated by most of the organic ligands.37 Therefore, we synthesized a new Gd1 CP (please see the Experimental section) and measured its phosphorescence spectrum at 77 K and fluorescence spectrum at room temperature (Fig. S5, ESI†). From the 77 K phosphorescence spectrum of Gd1, T1 of the DHTP is calculated which is equal to 21
800 cm−1 (458 nm) and is comparable to that of related terephthalate ligands reported previously.38 The energy gap (ΔE) between T1 and 5D0 falls within the range suggested by Latva's rule,39,40 ΔE = 4500 cm−1, highlighting the efficiency of Eu-CPs. Moreover, according to Reinhoudt's empirical rule,41 the intersystem crossing (ISC) process becomes effective if the energy difference, ΔE(S1 – T1), is around 5000 cm−1. To check this, we have also calculated the singlet state energy (S1) of DHTP which is 27
000 cm−1 (370 nm) with the ΔE(S1 – T1) ≈ 5200 cm−1 implying effective ISC in the sensitization process, Fig. S6 (ESI†).
The steady-state PL spectra of Eu(III) CPs were investigated in detail in the solid-state at room temperature, Fig. 3, and are summarized in Table 4. Upon excitation, the CPs exhibited typical Eu(III) emission transitions originating from the 5D0 excited state to the ground mutiplets: 7F0–4. The spectra in each case are dominated by the electric-dipole 5D0→7F2 transitions. It has been well documented that the ratio between the relative intensity of the magnetic-dipole and electric-diploe transitions i.e. R21 = I(5D0→7F2)/I(5D0→7F1) (I = integrated emission intensity of the corrected emission spectrum) can be used as a criterion for the symmetry distortion of the Eu(III) ion's nearest surroundings. The high value of R21 ≈ 10.28 and 12.70, respectively, for Eu1 and Eu2 suggests that the surroundings around the Eu(III) ion in the CPs are non-centrosymmetric, with a low symmetry of the crystal field (C2) for the Eu(III) centre in 8-coordinate and 9-coordinate geometries in agreement with their X-ray crystal structures.42 Moreover, the higher total integral intensity of 5D0→7F2 transition compared to 5D0→7F1 also indicates that the forced electric dipole and the dynamic coupling mechanism are dominant over magnetic dipoles.42
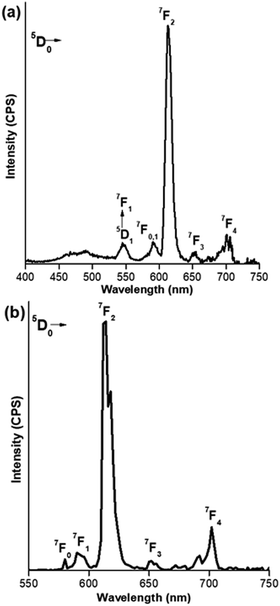 |
| Fig. 3 Room temperature solid-state PL spectra of the Eu-CPs: (a) Eu1 (excited at 370 nm) and (b) Eu2 (excited at 345 nm). | |
Table 4 Summary of the experimental photophysical parameters of the Eu(III) CPs at RT
|
Ω
2
|
Ω
4
|
FWHM |
τ
obs
|
τ
cr
|
K
R
|
K
NR
|
Q
Eu
|
R
21
|
CIE(x,y) |
× 10−20 cm2 |
(nm) |
(μs) |
(μs) |
(s−1) |
(s−1) |
Ω
2 and Ω4 were calculated by applying eqn (1) and (2). KR and kNR were calculated by applying eqn (2) and (4). QEu was calculated by applying eqn (6). |
Eu-1
|
18.05 |
6.29 |
11.01 |
78.00 |
1463 |
683.58 |
12136.93 |
5.33 |
10.28 |
(0.518, 0.340) |
Eu-2
|
22.29 |
8.50 |
8.78 |
600 |
1756 |
869.39 |
797.27 |
52.16 |
12.70 |
(0.668, 0.325) |
The CIE (Commission Internationale de l’Eclairage) coordinates are used to characterize the color and location of all the color coordinates produced within the limit of the CIE chromaticity diagram. The CIE color coordinates of Eu-CPs are shown in Fig. 4. The CIE color coordinates of Eu1 fall in the orange region while Eu2 displays typical red emission with CIE color coordinates very close to the National Television System Committee (NTSC) (0.668, 0.325) and thus Eu2 could be a promising candidate as a red component for the fabrication of LEDs.43 The orange emission of Eu1 could be due to the presence of residual ligand fluorescence, which arose because of the presence of water/DMF molecules in the inner coordination sphere that deactivates the excited state non-radiatively.
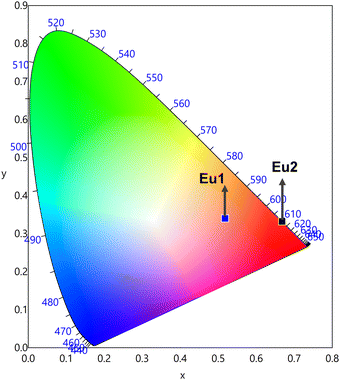 |
| Fig. 4 The CIE 1931 chromaticity diagram of the Eu(III) CPs in the solid-state. | |
To further understand the extent of participation of the non-radiative process in both Eu(III) CPs, we utilized time-resolved spectroscopy and determined the 5D0 excited state lifetime (τobs). τobs was determined by fitting the decay curves in Fig. S7 (ESI†). As expected Eu1 displayed an 8.46 fold shorter τobs value than Eu2. To clearly understand, we have utilized the steady-state emission spectra and τobs to calculate important photophysical parameters such as the radiative decay rate (kR), non-radiative decay rate (kNR) and the intrinsic quantum yield of the Eu(III) centre, QEu. The Ω2 and Ω4 values were also calculated by applying the mathematical eqn (1)–(6). All the obtained data are summarized in Table 4. It can be clearly seen from the table that Eu1 exhibited large KNR = 12,136.93 s−1 compared to Eu2 (KNR = 797.27 s−1). This behaviour is further reflected in the intrinsic quantum yield of Eu1 (QEu = 5.33) that is more than 10 times lower than that of Eu2 (QEu = 52.16). The value of QEu indicates the extent of non-radiative de-activation processes in the inner and outer coordination spheres of the Ln(III) ion. The Eu(III) emission is more efficient in Eu2 CP due to the presence of chelating TTA units that protect the Eu(III) center efficiently from coordinating water molecules that lead to de-activation processes. Furthermore, the high values of Ω2viz. 18.05 × 10−20 cm2 and 22.29 × 10−20 cm2 for Eu1 and Eu2, respectively, imply that the Eu(III) ions in the CPs are in a highly polarizable chemical environment, which further leads to higher values of R21. The higher Ω2 value of Eu2 compared to Eu1 could be attributed to strong delocalization of the asymmetric β-diketonate oxygen charge around Eu(III) inside the coordination sphere.44 Finally, the calculated Ω4 parameter is related to long-range effects such as NCIs (HB, π–π stacking, etc.) and rigidity. The high values of the Ω4 parameter in Table 4 suggest that the Eu(III) CPs display these features as verified by the crystal structure of the polymers.
Fabrication of LED
Because of the large intrinsic quantum yield of Eu2 CP (QEu = 52.16), its potential application as a red phosphor in fabricating a LED was investigated.45 First, the excitation spectrum of Eu2 in the solid-state was studied and presented in Fig. 5a. A broad band from 300 to 420 nm is observed in addition to 4f–4f transitions of Eu(III) from 7F0 → 5D2 and 7F0 → 5D1 transitions at 466 and 507 nm, respectively. This means that the Eu(III) centre can be sensitized by the absorption of light between 300 and 420 nm. In fact, DHTP can absorb UV-light at 378 nm. Therefore, the Eu(III) centre can also be sensitized by using blue LED irradiation under excitation at 375 nm. Indeed, a LED was fabricated by coating a resin of 10% Eu2 CP-silicon oil onto a blue InGaN chip.12 The emission spectrum of the Eu2/InGaN LED device is shown in Fig. 5b. It shows a combination of blue emission from the InGaN chip and a red luminescence from Eu2 CP. Interestingly, the CIE 1931 chromaticity coordinates exhibit a considerable shift of the colour coordinates of Eu2 from (0.668, 0.325) to (0.437, 0.185) when doped on the blue chip. A simple colour detection under a UV lamp reveals the emission of pink light from the LED (Fig. 5b inset). The red light from Eu(III) has mixed with the blue light from the InGaN chip to generate the pink light from the chip device. As a result, the Eu2 coordination polymer is promising for application as a red phosphor in fabricating LEDs.
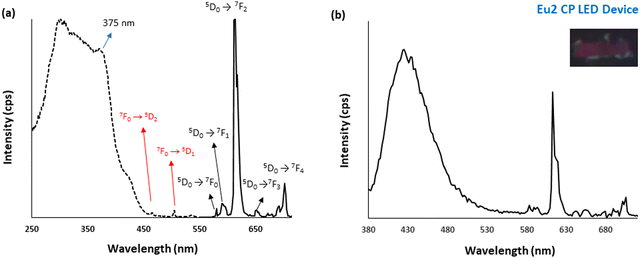 |
| Fig. 5 (a) The excitation and emission spectra of Eu2 CP and (b) the emission spectrum of the Eu2/InGaN chip device excited at 375 nm. | |
Conclusions
Novel Eu(III) CPs were successfully synthesized and characterized. The Eu(III) polymer: [Eu2(DHTP)(TTA)4(DMF)2(MeOH)2]n (Eu2) exhibited a large luminescence intrinsic quantum yield of 52% and high thermal stability up to 170 °C. A simple LED was fabricated by coating a resin of 10% Eu2 CP-silicon onto a blue InGaN chip. A pink light emission was observed from the device.
Conflicts of interest
The authors have no conflicts of interest to declare.
Acknowledgements
NKAl-R and MSK acknowledge His Majesty's Trust Fund for Strategic Research (Grant No. SR/SQU/SCI/CHEM/21/01) and the Ministry of Higher Education, Research and Innovation (MoHERI) (Grant No. RC-RG/SCI/CHEM/23/01) for funding. We thank Sultan Qaboos University, Oman for financial support. NAl-R thanks SQU for a PhD scholarship and RI thanks HM's Trust Fund for a postdoctoral fellowship.
References
- B. Xu, L. Yan, H.-M. Hu, C. Bai, L.-L. Xue and S. He, J. Solid State Chem., 2020, 288, 121424–121434 CrossRef CAS
.
- C. Marchal, Y. Filinchuk, X.-Y. Chen, D. Imbert and M. Mazzanti, Chem. – Eur. J., 2009, 15, 5273–5288 CrossRef CAS PubMed
.
- M.-L. Ma, J.-H. Qin, C. Ji, H. Xu, R. Wang, B.-J. Li, S.-Q. Zang, H.-W. Hou and S. R. Batten, J. Mater. Chem. C, 2013, 1, 4634–4639 RSC
.
- B. Gao, L. Fang and J. Men, Polymer, 2012, 53, 4709–4717 CrossRef CAS
.
- Y.-H. Han, C.-B. Tian, Q.-H. Li and S.-W. Du, J. Mater. Chem. C, 2014, 2, 8065–8070 RSC
.
- Z. S. Al-Farsi, A. Al-Rashdi and N. K. Al-Rasbi, Polyhedron, 2016, 117, 552–560 CrossRef CAS
.
- J. Yang, G.-D. Xie, X.-F. Chen, D. Wu, X.-M. Lin, G. Zhang and Y.-P. Cai, CrystEngComm, 2015, 17, 1326–1335 RSC
.
- C. F. Qiao, Y. Zhao, Y. Ren, X. Gao, M. Zhang, C. Zhou, G. Zhang and S. Chen, J. Solid State Chem., 2019, 270, 443–449 CrossRef CAS
.
- S. Wang, Q. Sun, B. Devakumar, J. Liang, L. Sun and X. Huang, J. Lumin., 2019, 209, 156–162 CrossRef CAS
.
- X.-Q. Song, H.-H. Meng, Z.-G. Lin and L. Wang, ACS Appl. Polym. Mater., 2020, 2(4), 1644–1655 CrossRef CAS
.
- Y. Kubo and R. Nishiyabu, Polymer, 2017, 128, 257–275 CrossRef CAS
.
- T. Koizuka, K. Yanagisawa, Y. Hirai, Y. Kitagawa, T. Nakanishi, K. Fushimi and Y. Hasegawa, Inorg. Chem., 2018, 57, 7097–7103 CrossRef CAS PubMed
.
- F. A. de Jesusa, B. V. Santanaa, G. F. da Cunha Bispob, C. I. da Silva Filhoc, S. A. Júniorc, M. E. Giroldo Valériob, J. M. Almeida Caiutd and V. H. Vitorino Sarmento, Polymer, 2019, 181, 121767 CrossRef
.
- P. Thuery and B. Masc, Cryst. Growth Des., 2010, 10(8), 3626–3631 CrossRef CAS
.
- M. Latva, H. Takalo, V. M. Mukkala, C. Matachescu, J.-C. Rodriguez-Ubis and J. Kankare, J. Lumin., 1997, 75, 149–169 CrossRef CAS
.
- A. E. Sedykh, D. G. Kurth and K. Müller-Buschbaum, Eur. J. Inorg. Chem., 2019, 4564–4571 CrossRef CAS
.
- K. P. Kepp, Inorg. Chem., 2016, 55, 9461–9470 CrossRef CAS PubMed
.
- J. Ma, F.-L. Jiang, L. Chen, M.-Y. Wu, S.-Q. Zhang, K.-C. Xiong, D. Han and M.-C. Hong, CrystEngComm, 2012, 14, 6055–6063 RSC
.
- J. D. Einkauf, J. M. Clark, A. Paulive, G. P. Tanner and D. T. de Lill, Inorg. Chem., 2017, 56, 5544–5552 CrossRef CAS PubMed
.
- A.-J. Huang, L.-H. Leng, D.-Q. Xin and S.-C. Ding, Inorg. Nano-Met. Chem., 2020, 1–8 CAS
.
- A. Lämmermann, I. Szatmári, F. Fülöp and E. Kleinpeter, J. Phys. Chem. A, 2009, 113, 6197–6205 CrossRef PubMed
.
- A. Haque, K. M. Alenezi, M. S. Khan, W.-Y. Wong and P. R. Raithby, Chem. Soc. Rev., 2023, 52, 454–472 RSC
.
- R. Ilmi, D. Zhang, L. Tensi, H. Al-Sharji, N. K. Al Rasbi, A. Macchioni, L. Zhou, W.-Y. Wong, P. R. Raithby and M. S. Khan, Dyes Pigm., 2022, 110300 CrossRef CAS
.
- R. Ilmi, J. Yin, J. D. L. Dutra, N. K. Al Rasbi, W. F. Oliveira, L. Zhou, W.-Y. Wong, P. R. Raithby and M. S. Khan, Dalton Trans., 2022, 51, 14228–14242 RSC
.
- X. Lucas, C. Estarellas, D. Escudero, A. Frontera, D. Quiñonero and P. M. Deyà, Chem. Phys. Chem., 2009, 10, 2256–2264 CrossRef CAS PubMed
.
-
Stoe & Cie, X-AREA, version 1.30: Program for the acquisition and analysis data, Stoe & Cie GmbH, Darmatadt, Germany, 2005 Search PubMed
.
-
Stoe & Cie, X-RED, version 1.28b: Program for data reduction and absorption correction, Stoe & Cie GmbH, Darmatadt, Germany, 2005 Search PubMed
.
-
G. M. Sheldrick, SHELX97. Program for crystal structure solution and refinement, University of Göttingen, Germany, 1997 Search PubMed
.
- L. J. Farrugia, J. Appl. Crystallogr., 1999, 32, 837–838 CrossRef CAS
.
- C. F. Macrae, P. R. Edgington, P. McCabe, E. Pidcock, G. P. Shields, R. Taylor, M. Towler and J. van de Streek, J. Appl. Crystallogr., 2006, 39, 453–457 CrossRef CAS
.
- R. Ilmi, W. Sun, J. D. L. Dutra, N. K. Al-Rasbi, L. Zhou, P.-C. Qian, W.-Y. Wong, P. R. Raithby and M. S. Khan, J. Mater. Chem. C, 2020, 8, 9816–9827 RSC
.
- J. Li, X. Zhang, B. Yue, A. Wang, L. Kong, J. Zhou, H. Chu and Y. Zhao, Crystals, 2017, 7, 139 CrossRef
.
- B. C. Barja, A. Remorino, M. J. Roberti and P. F. Aramendia, J. Argent. Chem. Soc., 2005, 93, 81–96 CAS
.
- J. Ye, J. Zhang, G. Ning, G. Tian, Y. Chen and Y. Wang, Cryst. Growth Des., 2008, 8, 3098–3106 CrossRef CAS
.
- J. D. Einkauf, T. T. Kelley, B. C. Chan and D. T. de Lill, Inorg. Chem., 2016, 55, 7920–7927 CrossRef CAS PubMed
.
- S. Tobita, M. Arakawa and I. Tanaka, J. Phys. Chem., 1985, 89(26), 5649–5654 CrossRef CAS
.
- R. Ilmi, M. S. Khan, Z. Li, L. Zhou, W.-Y. Wong, F. Marken and P. R. Raithby, Inorg. Chem., 2019, 58(13), 8316–8331 CrossRef CAS PubMed
.
- C. Serre, F. Millange, J. Marrot and G. Férey, Chem. Materials, 2002, 14, 2409–2415 CrossRef CAS
.
- N. K. Al-Rasbi, H. Adams and F. O. Suliman, Dyes Pigm., 2014, 104, 83–88 CrossRef CAS
.
- A. Al-Bahri, A. S. Al-Zakwani, S. M. Al-Farsi and N. K. Al-Rasbi, ChemistrySelect, 2016, 1, 1393–1399 CrossRef CAS
.
- F. J. Steemers, W. Verboom, D. N. Reinhoudt, E. B. van der Tol and J. W. Verhoeven, J. Am. Chem. Soc., 1995, 117(37), 9408–9414 CrossRef CAS
.
- R. Ilmi, S. Anjum, A. Haque and M. S. Khan, J. Photochem. Photobiol., A, 2019, 282, 111968 CrossRef
.
- R. Ilmi, D. Zhang, J. D. L. Dutra, N. Dege, L. Zhou, W.-Y. Wong, P. R. Raithby and M. S. Khan, Org. Electron., 2021, 96, 106216 CrossRef CAS
.
- I. J. Al-Busaidi, R. Ilmi, D. Zhang, J. D. L. Dutra, W. F. Oliveira, N. K. Al Rasbi, L. Zhou, W.-Y. Wong, P. R. Raithby and M. S. Khan, Dyes Pigm., 2022, 197, 109879 CrossRef CAS
.
- L. Armelaoa, S. Quicib, F. Barigelletti, G. Accorsic, G. Bottarod, M. Cavazzinib and E. Tondelloe, Coord. Chem. Rev., 2010, 254, 487–505 CrossRef
.
|
This journal is © The Royal Society of Chemistry 2023 |