DOI:
10.1039/D3TB01494K
(Review Article)
J. Mater. Chem. B, 2023,
11, 9369-9385
Comprehensive evaluation and advanced modification of polymethylmethacrylate cement in bone tumor treatment
Received
1st July 2023
, Accepted 28th August 2023
First published on 4th September 2023
Abstract
Bone tumors are invasive diseases with a tendency toward recurrence, disability, and high mortality rates due to their grievous complications. As a commercial polymeric biomaterial, polymethylmethacrylate (PMMA) cement possesses remarkable mechanical properties, injectability, and plasticity and is, therefore, frequently applied in bone tissue engineering. Numerous positive effects in bone tumor treatment have been demonstrated, including biomechanical stabilization, analgesic effects, and tumor recurrence prevention. However, to our knowledge, a comprehensive evaluation of the application of the PMMA cement in bone tumor treatment has not yet been reported. This review comprehensively evaluates the efficiency and complications of the PMMA cement in bone tumor treatment, for the first time, and introduces advanced modification strategies, providing an objective and reliable reference for the application of the PMMA cement in treating bone tumors. We have also summarized the current research on modifications to enhance the anti-tumor efficacy of the PMMA cement, such as drug carriers and magnetic hyperthermia.
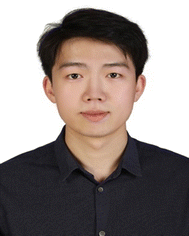
Bo Chao
| Bo Chao was born in Inner Mongolia, China. He received his BS degree in 2021. He is currently pursuing his MS degree under the guidance of Prof. Minfei Wu in the Department of Orthopedics, Second Hospital of Jilin University. His research interests are focused on the multifunctional therapy of bone tumors and the modification of bone cement. |
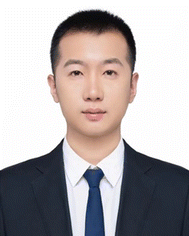
Zhonghan Wang
| Zhonghan Wang is an attending doctor in the Orthopedic Department at the Second Hospital of Jilin University. He received his BS degree from Central South University in 2015, obtained his MS degree from Jilin University in 2018 and his MD degree in 2022. His research mainly focuses on preparing anti-tumor materials and regenerative medicine of the skeletal system. |
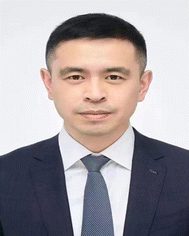
Minfei Wu
| Minfei Wu received his MD degree from the Orthopedic Department, at the Second Hospital of Jilin University in 2014. During this period, he worked as a visiting scholar at the Massachusetts General Institute affiliated with Harvard University. At present, he is a vice-principal, professor, chief physician, and doctoral supervisor at the Second Hospital of Jilin University. His research mainly focuses on the treatment of spinal tumors and the minimally invasive treatment of spinal cord injuries. He has designed a series of novel biomaterials for bone tissue engineering, including low-temperature bone cement sustained-release carrier materials, magnesium-based anti-tumor implants, and 3D printing topology-optimized spinal implants. |
1. Introduction
Bone tumors are nociceptive diseases characterized by severe recurrence, disability, and high mortality rates1,2 and can be classified as benign or malignant according to biological behaviors. Benign bone tumors include diverse tumors and are distinct in incidence and clinical presentation.3 Malignant bone tumors include metastatic and primary bone tumors.4 Osteosarcoma is the most frequently occurring primary malignant tumor that originates from the bone. In contrast, metastatic malignant bone tumors are always derived from terminal lung, breast, and prostate cancer.5–7 Intermediate bone tumors are also regarded as a type of bone tumor, such as the giant cell tumor of the bone (GCTB).8,9 Whatever the type of bone tumor, it can constitute a serious threat to the patient's quality of life and is responsible for grievous complications, involving pathological fractures, osteolysis, hypercalcemia, and extreme pain.10,11 Pathological compression fracture, spinal cord compression, and even paraplegia frequently afflict patients when cancer occurs in the spine.12
The current clinical treatment of bone tumors is predominantly surgical curettage but is generally accompanied by extensive bone defects.13,14 Notably, the most fundamental intrinsic property of bone tissue, stability, will disappear following surgical curettage without filling implants.15,16 In addition, clinical judgment of complete tumor eradication is unreliable, depending only on rapidly frozen sections and the experience of surgeons,17 resulting in residual tumor cells frequently remaining around bone defects, which are insensitive to radiation with a tendency to resist chemotherapy.18–20 As a result, the recurrence of post-operative bone tumors and persistent pain are frequent, and these issues necessitate more effective treatment.
Polymethylmethacrylate (PMMA) cement generally serves as a substitute for bone, with the advantages of being plastic, injectable, with high mechanical strength and the ability to fill defects caused by orthopedic diseases or surgical resection.21,22 PMMA cement is a double-phase system composed of polymer powder and monomer liquid.23,24 The polymer powder principally consists of spherical PMMA beads and benzoyl peroxide (BPO). The monomer liquid is mainly methyl methacrylate (MMA) and N,N-dimethyl-p-toluene (DmpT).25 While mixing the two phases, BPO reacts with DmpT to produce free radicals (benzoyl radicals and benzoyl anions), and the polymerization of MMA into PMMA will be subsequently initiated by conjugating to the polymerizable double bonds of the monomer molecules and the creation of a productive center. In addition, theoretically, PMMA cement generates heat during the polymerization reaction, killing the residual tumor cells around tumor defects by the exothermic polymerization reaction.26,27 Nevertheless, only tumor cells in an extremely restricted area will be killed by the exothermic polymerization reaction, and healthy bone tissue may be damaged as a result.28,29 Most scholars conclude that the neurological symptoms of tumor-derived bone defects filled with PMMA cement are significantly relieved, and the rate of tumor recurrence and secondary fracture are also reduced compared to patient bone defects without PMMA cement.30 However, some perspectives indicate that the absence of robust evidence to prove the risk of tumor recurrence can be reduced by filling tumor-derived bone defects with PMMA cement.31,32 There are also many reports of complications when using PMMA cement, such as cement leakage,33,34 cardiac tamponade,35 dislodgement of cement,36–38 osteoarthritis,39,40etc.
The aseptic loosening of PMMA cement may occur after injecting it into the tumor-derived bone defect due to the progressive growth and osteolysis of residual cancer cells.41,42 To avoid this situation, improving the tumor inhibition efficiency of cement is a reasonable method. Chemotherapeutics-loaded PMMA cement is the most common and accessible approach to modification.43,44 The local concentration of chemotherapeutics is prominently increased by chemotherapeutics-loaded PMMA cement, while the plasma concentration of chemotherapeutics is significantly reduced, which effectively weakens the extent of systematic side effects.45 In addition to being optimized as a drug carrier, PMMA cement can also be modified to possess magnetic hyperthermal capability.46 Magnetic hyperthermia, as an adjuvant treatment for cancer treatment, will kill tumor cells at high temperatures.47,48 The appropriate temperature between 41 °C and 45 °C will be generated in an alternating magnetic field (AMF) by mixing magnetic thermal seeds into PMMA cement.49 Notably, the benefits of PMMA cement in the treatment of bone tumors will be prominently improved by both modification methods.
In this review, the efficiency of PMMA cement in the treatment of bone tumors is comprehensively introduced, including biomechanical stabilization, the analgesic effect, and tumor recurrence prevention. In addition, the possible complications of PMMA cement during treatment will also be presented objectively. The advantages and disadvantages of the current clinical application of PMMA cement are compared and discussed, which is convenient for weighing the pros and cons more rigorously. Moreover, we also discuss the advanced modification approaches of PMMA cement and its benefits. This review aims to evaluate the various functions of PMMA cement and possible complications in bone tumor treatment, further introducing advanced modification methods, to provide an objective and reliable reference for the application of PMMA cement in treating bone tumors (Scheme 1).
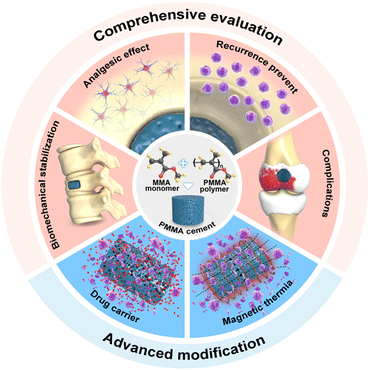 |
| Scheme 1 Schematic illustration of the comprehensive evaluation and advanced modification of PMMA cement in the treatment of bone tumors. | |
2. Evaluation of PMMA in bone tumor treatment
Currently, the major clinical treatment for bone tumors is surgical resection, which frequently involves large bone defects that require PMMA cement as an orthopedic implant to fill and provide mechanical support. Therefore, the application of PMMA cement in bone tumor treatment was evaluated systematically in this section, including biomechanical stabilization, the analgesic effect, prevention of recurrence, and complications.
2.1. Maintaining biomechanical stabilization
Healthy bone tissue possesses stable self-healing ability. However, such self-healing ability is restricted to the repair of small bone defects and is only achievable in the presence of a healthy bone tissue microenvironment.50 Critical bone defects caused by surgical curettage or osteolytic bone tumors make it difficult to restore normal physiological structure without medical intervention.51 Autologous bone grafting is an ideal filler with a high success and fit rate. Nevertheless, restricted availability of autogenous bone, infection tendency, massive blood loss, etc., are all adverse factors in clinical autologous bone grafting.52,53 Therefore, contemporary researchers and surgeons have used various biomaterials as substitutes for bone tissue.51 PMMA cement is one of the most popular biomaterials, characterized by injectability, plasticity, convenience, and good mechanical properties.54,55 Since the shape and the area of the bone defect can be neatly matched by PMMA cement, it plays a crucial role in the reconstruction of the stability of tumor-derived bone defects.
2.1.1 Restoration of spinal stability.
PMMA cement is mostly applied to spinal metastases as a filler for tumor-derived bone defects. The spine is one of the major weight-bearing organs of the body and the most frequent metastatic locale for malignant tumors with a metastatic rate of up to 70%.56 Therefore, the primary aim of reconstructive surgery after spinal tumor decompression is to restore spinal stability.57 Scoville et al.58 first demonstrated the feasibility of PMMA-assisted anterior reconstruction in a patient suffering from spinal metastases in 1967. The recovery of spinal function with the slightest damage is the major advantage of PMMA cement in vertebral replacement due to the limited life expectancy of patients, who should not be immobilized in the bed by plaster or traction in the final months but rather have quality of life through spinal reconstruction.58 In addition to patients with limited anticipated survival, reliable therapeutic results were demonstrated over a 13 year follow-up using PMMA cement in one patient with spinal metastases. The anterior bone fusion was firm and the stability of the vertebral body was restored significantly as observed in the computed tomography (CT) imaging 13 years after the operation.59 Percutaneous vertebroplasty (PVP) is a minimally invasive procedure that can infuse PMMA cement and restorative vertebral body structure.60,61 Wong et al.62 examined this procedure as ideal in situations where bone strengthening is required in patients with spinal metastasis. After filling the bone defect through PVP, the patient reported being able to recuperate mobility independently in the immediate postoperative phase.62 PVP showed good efficacy in the reconstruction of single spinal metastases but the stability of the reconstruction of multilevel spinal metastasis is unsatisfactory, even with temporary paraparesis.63 Therefore, a modified treatment of PMMA has been proposed for the reconstruction of multilevel spinal metastasis. In a study of patients with spinal metastases fixed with PMMA-augmented screws, two of the four patients who were unable to walk regained the ability to walk immediately after surgery. During the 7 month follow-up, no vertebral collapse was observed in all patients.64 Therefore, screw fixation augmented with PMMA cement can provide dependable stability for multilevel spinal metastasis. In bone cement-augmented pedicle screw fixation combined with vertebroplasty, also known as the sandwich procedure with an execution sequence, PMMA cement was injected first after tumor curettage, and then pedicle screws were tightened when the PMMA cement was solidified.65 The efficacies of PVP- and bone cement-augmented pedicle screw fixation were compared in the treatment of spinal metastases, and subtotal vertebral resection with pedicle screw fixed reconstruction showed better efficacy.66 All of the above treatments are anterior column vertebral reconstructions of spinal metastasis, and complex vertebral reconstruction requires improved procedures. In the case of a three-column osteotomy of the spinal defect, multiple auxiliary rods were required to support the strength of the structure of the sandwich procedure. The multiple-rod constructs had a lower overall hardware failure rate than the single-rod ones. The strength of the cement-augmented quadruple rod constructs provided reliable stability for patients with complex neoplastic vertebral defects.67
2.1.2 Restoration of the stability of limbs.
The tumor-derived defects of the vertebral body can be repaired with PMMA but it also can be applied to defects in limbs and other bones to restore stability, especially for bed-ridden patients with contraindications to surgery. A patient with osteolytic defects in the proximal humerus with a life expectancy of less than 1 month was administered PVP to provide axial loading with minimal damage by filling PMMA cement.68 Even for children with immature skeletons, PMMA cement as a structural strengthening material can be used to improve the mechanical stability of the bone, despite requiring the postoperative removal of the PMMA cement to avoid disturbing growth.69 GCTBs, generally present in the distal femur, are frequently conducive to severe fractures after surgical curettage.70 Injecting PMMA cement after the curettage of GCTBs can significantly restore the function of limb bones.71–75 In the report by Alejandro et al.,76 the use of bone cement after the curettage of the GCTB lesion is a better choice than allogeneic bone because adequate structural support for the bone in knee reconstruction can be provided by PMMA cement. Another study also exposited that filling PMMA cement after curettage of GCTBs not only reconstructed the tumor-derived bone defects but also reduced the risk of postoperative fracture. However, additional plate fixation will be considered if PMMA cement treatment is not an opinion, which increases the difficulty and the cost of surgery.77 A new surgical technique for subchondral bone grafting combined with PMMA cement reconstruction was developed for the treatment of GCTBs, which can more adequately restore the subchondral and cancellous positions of the joint surface with minimal mechanical problems (Fig. 1). The optimal recovery of structural integrity and limb function was achieved postoperatively.78 In addition, encouraging results were obtained with the treatment of osteolytic cancer in the pelvis with PMMA cement. All postoperative patients were able to walk independently with the loading-operated limb, and the patients appreciated the significant improvement in their quality of life.79
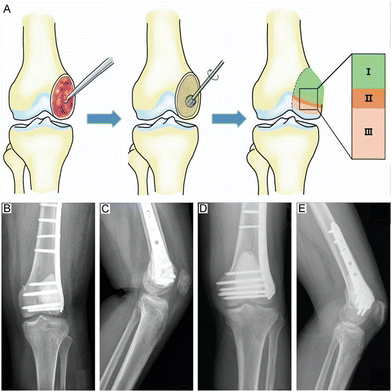 |
| Fig. 1 Schematic diagram of PMMA cement implantation after GCTB removal. (A) Schematic illustration of GCTB curettage showing an appropriately sized cortical window and the application of a high-speed burr to eliminate pockets of residual tumors in the cavity, followed by grafting of subchondral cancellous bone and cortical bone. (I) PMMA cement implant area. (II) Cortical bone grafting area. (III) Subchondral cancellous bone grafting area. (B) and (C) Follow-up X-rays at 25 months demonstrated excellent plasticity of the subchondral bone graft without joint degeneration. (D) and (E) Follow-up X-rays showed good plasticity of the subchondral bone graft without joint degeneration. Reproduced with permission.78 Copyright 2018, Wolters Kluwer Health. | |
2.2. Analgesic effect
In addition to skeletal instability, the risks of pathologic fractures or nerve compression will be increased by bone tumors. The subsequent tormenting pain or paralysis severely damages the quality of the patients' lives if not treated expeditiously.80,81 The neurophysiological mechanisms of cancer pain may be implicated in neurochemicals produced by tumors and inflammatory cells, of peripheral sensitization due to nerve compression and injury caused by tumor growth.82,83 Affected by the continuous erosion of residual cancer cells into the surrounding bone tissue and nerves, neurogenic bone pain may be stimulated by the disturbance of the highly innervated periosteum.84 Another cause of pain is the instability of pathological fracture, imposing a compulsive position on patients.85
Regardless of the cause of bone pain, filling PMMA cement in tumor-derived bone defects can effectively relieve neurological symptoms in patients. The main principles are as follows. (1) With the penetration of PMMA cement into the interspace of the partially fractured bone trabeculae, the strength and original stability of bones are restored to the greatest extent. (2) The heat and chemically toxic substances generated from the polymerization reaction of PMMA cement kill the eroded nociceptive nerve endings and the residual tumor cells.86,87 The application of PMMA cement in relieving neurological symptoms will be introduced in this section.
2.2.1 Relieving bone pain.
A certain curative effect on painful bone tumors will be presented by radiotherapy but it cannot correct the instability of bones. Therefore, the radiotherapeutic effect is usually mediocre in relieving the neurological symptoms of patients with bone tumors.88,89 To verify that PMMA cement can indeed improve the symptoms of pain in patients with bone tumors, a patient with lumbar spinal tumors from thyroid follicular carcinoma tried analgesic agents, external beam radiation therapy, radioiodine therapy, and posterior fusion surgery but the pain still reemerged and progressed90 until the pain was significantly relieved after PVP. At least as illustrated in this case, PVP can be used as an alternative pain relief method for patients with vertebral tumors. PVP is a reliable treatment for pain relief, which was also illustrated by the significant reduction in the mean amounts of non-narcotic and narcotic analgesic after surgery as compared with before surgery.91 Even for intractable pain, PVP is an effective alternative for pain relief.92 Further explanation of the mechanism is provided by the principles described above, whereby the heat and chemical toxicity of the PMMA cement polymerization reaction kills the eroded nociceptive nerve endings, thus relieving the bone pain immediately.86,87 In another retrospective analysis of patients whose primary presentation was pain and neurological impairment, the neurological status was improved in 60% of patients after injecting PMMA cement for vertebral metastases; 81% of the patients in this study were satisfied or very satisfied with the effect of pain relief.93 Yang et al.94 illustrated the idea that PMMA cement provides biomechanical stability for pain relief in fragile vertebrae by reviewing 57 patients without new or adjacent vertebral fractures within two years after PVP. More effective pain relief would be demonstrated by PVP combined with radiotherapy than PVP by itself. Patients with osteolytic metastatic spinal tumors were treated with radiotherapy to suppress the tumor or induce pain relief immediately after PVP.95
2.2.2 Relieving neurological compression.
In combination with the mechanism of cancer pain,82 an alternative mechanism in which PMMA cement can be effective in relieving pain is to relieve nerve compression, as bone eroded by tumor cells often becomes structurally unstable and then compresses nerve roots or the dural sac. Although some opinions on tumor-epidural encroachment are a relative contraindication to PVP,96,97 the choice of PVP for painful spinal metastases in patients with epidural encroachment remains a controversial issue. A retrospective study was the first to review the efficacy and safety of patients with epidural encroachment treated by PVP. In the postoperative follow-up of 51 patients included in the study, the effective pain relief rate was 94% at 1 day and 92% at 1 year, which was evaluated by the Kaplan–Meier survival curve and with a low complication rate.98 In addition, an appreciable effect of analgesia was also demonstrated in other retrospective studies of patients with epidural spinal metastases treated by PVP.99,100 Li et al.101 designed an innovative approach, PVP combined with interventional tumor removal (ITR), which can better relieve symptoms of neurological compression than PVP. The main advantage is that PVP after ITR eliminates residual tumor tissue as much as possible, providing long-term pain relief. Radicular pain related to the malignant tumor compressing the dorsal root ganglion was also significantly relieved after PVP.102 Severe pelvic bone metastasis often causes pain and gait disturbance. As shown in Fig. 2, the percutaneous injection of PMMA cement into the pelvis is a feasible and safe palliative surgical option for pain management, and not only relieves pain under local anesthesia but can also maintain walking function.103
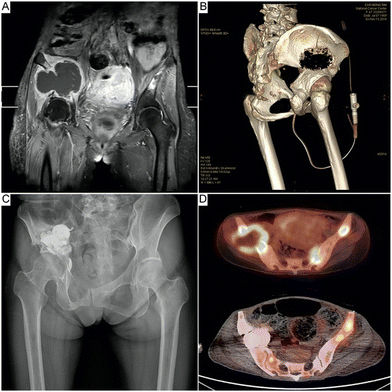 |
| Fig. 2 Representative images of percutaneous pelvic cementoplasty. (A) and (B) T1-enhanced magnetic resonance imaging and computed tomography imaging showing osteolytic bone metastases with cortical defects in the right acetabulum. (C) Post-operative X-ray showing reinforcement of the acetabulum. (D) Positron emission tomography–computed tomography images showing disease progression at 15 months postoperatively, except at the right acetabulum (upper, preoperative; lower, 15 month postoperative status). Reproduced with permission.103 Copyright 2021, Springer Nature. | |
2.3. Preventing recurrence
The recurrence of bone tumors often contributes to many grievous problems for patients and clinical management. Once the tumor recurs, the patient will not only suffer from the administering of additional treatment even reoperation, but more seriously, the patient's condition will continue to worsen.104 Local recurrence of spinal metastasis is relatively common, even after aggressive surgery. Moreover, spinal column instability and spinal cord compression will always occur consequently.105 However, due to the limited life expectancy of patients with spinal metastasis of malignant tumors, most of them died without retrospective statistics. As intermediate bone tumors, the recurrence rate of GCTBs is still high, attributed to narrow surgical margins.106 Whether patients with GCTBs treated with PMMA cement have a lower recurrence rate is consistently controversial and will be summarized and discussed in this section. For a more straightforward overview of different perspectives, we have compiled the relevant research of different perspectives in Table 1.
Table 1 Different perspectives on whether PMMA cement-treated patients with bone tumors have decreased recurrence rates
Different perspectives |
Summation |
Ref. |
Supporting perspectives |
In the subgroup of 200 patients surgically treated within the lesion, filling PMMA cement was administered in 194 patients and supported highly statistically significant administration of PMMA cement. |
107
|
Using PMMA cement as an adjuvant significantly reduces the rate of recurrence after intralesional treatment of benign giant cell tumors, and it appears to be the preferred treatment for both primary and recurrent giant cell tumors of bone. |
108
|
Minimal local recurrence rates were achieved in PMMA-treated patients, indicating that PMMA is more appropriate as a routine clinical treatment for eligible patients with GCTB. |
109
|
Recurrent GCTB treatment is available by further curettage with subsequent debridement and PMMA, with an acceptable re-recurrence rate of only 21.7%. |
110
|
The combination of all adjuvants (PMMA, burring, H2O2 − n = 42) reduces the probability of recurrence by 28.2-fold relative to curettage alone and should therefore be recommended as standard treatment. |
111
|
The combination of curettage, phenol flushing, electrocautery, and PMMA is effective in preventing local recurrence and can replace en bloc resection with large margins. |
112
|
The combination of burring, argon plasma cauterization, phenolisation, and PMMA cement in GCTB treatment is a safe and effective procedure to reduce the rate of local recurrence. |
113
|
Satisfactory function at mid-term follow-up, low recurrence rate, and effective treatment of GCTB with locally enlarged curettage, electrocautery, and PMMA cement. |
114
|
Opposing perspectives |
Analyzing the results of 49 patients with forearm GCTB treated from 2 to 28 years, the highest number of recurrences was in patients who received autograft or PMMA cement after curettage. |
115
|
There was no effect of PMMA cement use on recurrence rates compared with bone grafting (RR, 0.98; 95% CI, 0.44–2.17). |
116
|
PMMA cement is not regarded as a local adjuvant to prevent the recurrence of bone tumors; it is simply a mechanical reinforcement of the tumor cavity. |
117
|
2.3.1 Supporting perspectives.
Kivioja et al.107 reported that local recurrence rates following surgical curettage would be prominently decreased by PMMA cement, supporting the comprehensive preferential use of PMMA treatments. Similar results were reported in another study, in which 22% of GCTB patients treated with PMMA cement and 49% of GCTB patients treated with bone graft emerged with local recurrence within 63 months, evidence that further supports applying PMMA cement to inhibit the recurrence of GCTBs.108 Another systematic review and meta-analysis compared the efficacy of PMMA and allogeneic bone grafting following intralesional curettage GCTBs. The recurrence rate of GCTB patients who were administered PMMA cement repair after surgical curettage was relatively lower than that of patients who received bone grafting (RR 2.09, 95% confidence interval (1.64, 2.66), overall effect: Z = 6.00; P < 0.001).109 The burring of the cavity with a high-speed air drill followed by PMMA cement effectively inhibited the tumor recurrence.110,111 Some studies have also explored new technology and found that the combination of radical curettage, phenol irrigation, electrocautery, and PMMA cement could effectively prevent local recurrence.112,113,118 Even without any chemical or physical local adjuvant therapy, combination treatment followed by radical curettage, phenol irrigation, electrocautery, and PMMA cement could still obtain satisfactory results with a low recurrence rate.114
2.3.2 Opposing perspectives.
Many opinions indicate that PMMA cement is not an independent factor in reducing the tumor recurrence rate. There was no difference in recurrence rates in several studies that directly compared patients who received PMMA cement versus bone grafts.115,116,119 PMMA cement is not supposed to be a local adjuvant to tumor cells after curettage but rather a mechanical reinforcement of tumoral defects according to Jacob et al.117 Although there is still controversy about whether PMMA alone can reduce the rate of tumor recurrence, it was confirmed that PMMA cement possessed ideal radiological features to identify local recurrence;120,121 moreover, the tumor recurrence rate can effectively be reduced by PMMA-related combination treatment.112,113
2.4. Complications
The multifunctional role of PMMA cement in the treatment of bone tumors was introduced above. Reconstructing tumor-derived bone defects, relieving neurological symptoms, and inhibiting tumor recurrence have been achieved with considerable efficacy. However, the technique of injecting PMMA cement for the treatment of bone tumors is not without pitfalls.122 This section summarizes the challenges and potential complications during the administering of PMMA cement to facilitate predicted detection, provide treatment recommendations for complications, and minimize procedural risks.
2.4.1 Thermal damage.
Thermal heat from the polymerization reaction of PMMA cement kills residual tumor cells and pain nerve endings, as confirmed above.86,87 However, thermal damage may significantly risk overkill, injuring substantial amounts of healthy tissue.123 There was even research indicating that thermal damage to the spinal cord during PMMA cement application may be more predominant in the grey matter compared to other neural tissues.124 For injecting PMMA cement around the distal femur or proximal tibia, thermal damage to the adjacent articular cartilage occurs relatively frequently. Radev et al.125 determined possible thermal damage around PMMA by finite element analysis, implants (8–24 cc in volume) placed into a peripheral metaphyseal defect in the proximal tibia. The result indicated thermal injury to both normal bone and remaining tumor cells in the vicinity of the PMMA implant at the time of its polymerization. Although the necrotic effect extends only to limited areas surrounding bone, the surrounding cancellous and subchondral bone will be damaged. Mechanical vulnerability and the collapsing of bone under joint loading might be a consequence. In comparative research on the depth of tissue necrosis caused by different adjuvant therapies after bone tumor curettage, the average depth of tissue necrosis of PMMA cement was 0.78 mm. The authors also indicated that the exothermal effect of PMMA cement is associated with volume, and the depth of tissue necrosis caused by PMMA cement may be underestimated as compared to the actual clinical application.126
Tissue necrosis caused by the exothermal effect of PMMA cement is a risk factor for osteoarthritis. A retrospective single-center study involved fifty-three patients with GCTBs around the knee treated with curettage followed by PMMA cement; after the procedure, 17% of patients had radiographic findings of osteoarthritis.39 To safeguard against osteoarthritis, an insulating layer between the PMMA cement and articular cartilage was utilized to protect the articular cartilage. This insulating area was created using oxidized cellulose and subchondral cancellous allograft after the PMMA cement had completed polymerization. The intermediate outcome follow-up of this patient with GCTB has been promising.127 Another novel subchondral bone grafting procedure was proposed by Wu et al.78 to reduce the risk of osteoarthritis. It involved packing autogenous bone grafts, approximately 10 mm thick, in the subchondral bone defect, followed by filling PMMA cement in the remaining cavity. This novel bone-grafting procedure increased the distance between the exothermic reaction of PMMA cement and the articular cartilage, thereby reducing the pressure on the cartilage and subchondral bone layer. Similarly, Joseph et al.128 also indicated that patients with additional subchondral bone grafts after curettage had significantly fewer postoperative complications, including fractures and arthritis, and this did not increase the probability of tumor recurrence as compared with PMMA cement alone.
The temperature during the exothermic polymerization of PMMA cement in vivo contributes to the thermal necrosis of osteoblasts and impaired local circulation, which potentially lead to premature failure.129 Moreover, the degree of heat generated throughout the polymerization is influenced by the amount of reacting monomer.130 In summary, the distance between the heat of PMMA polymerization and the articular cartilage will be increased by bone grafting, which can prevent thermal damage. Alternatively, flushing the surrounding area with frozen saline as a cement-curing agent can prevent thermal necrosis.
2.4.2 Cement leakage.
The anterior and posterior walls of the vertebral body are frequently destroyed by invasive spinal metastases. Therefore, cement leakage often occurs after using PMMA cement for spinal reconstruction, including cortical cement leakage and vascular cement leakage,131 which is associated with risks of mechanical damage to the anterior aorta and posterior spinal cord of the vertebral body.132,133 Cement leakage is responsible for the procedure's location and needle approach.122 Moreover, incomplete posterior wall and injection volume are risk factors for vascular cement leakage,134,135 patients with posterior wall defects are often associated with a higher risk of cement leakage.136 Although this situation of cortical cement leakage is usual, most patients with cement leakage do not manifest corresponding clinical symptoms.137–140 Symptoms caused by cortical PMMA leakage can be relieved through the appropriate hormone and analgesic treatment.92
PMMA emboli formed by cement leakage can also transfer from the paravertebral veins to the inferior vena cava, renal vein, right heart, or pulmonary arterial system,63,141,142 even the dorsal pedal artery.143 As shown in Fig. 3, the cement leakage appeared in the eighth thoracic vertebra. The majority of PMMA emboli are small without any clinical manifestations, and no additional treatment is required.63 However, cases of severe cardiopulmonary complications also occur occasionally.144 There has been a report of fatal pulmonary embolism caused by PMMA cement,145 and a case of cardiac perforation caused by PMMA escaping into the vascular system was reported by Zhang et al.146 Even if it occurs in the administration of fracture treatment, more precautions should be taken in the procedure of bone tumor treatment. In addition, the displacement of bone marrow into the periosteal venous plexus may be attributed to intensive pressure during cement injection that travels to the pulmonary circulation as fat emboli.147–149
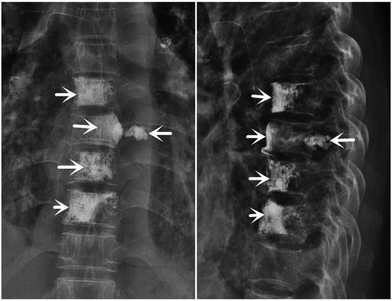 |
| Fig. 3 Representative images of cement leakage. Postoperative X-ray images show most of the PMMA cement located within the vertebral body, with very slight leakage outside of the eighth thoracic vertebra but not into the spinal canal. Reproduced with permission.63 Copyright 2020, SAGE Publications Ltd. | |
To avoid cement leakage, Li et al.150 indicated that tumor tissue should be completely eliminated before PMMA injection to create voids and subsequently, the occurrence rate of complications will be relatively reduced. Vertebral body lavage before PMMA injection,151 and limiting the PMMA injection to less than 30 ml per vertebral body will also prevent the formation of fat embolisms.152 Whatever, rigorous monitoring is the primary measure to reduce the risk of PMMA leakage,153 additional scaffolds can also provide a barrier function for cement.154,155 Some novel biomaterials exhibited appreciable effects in preventing the leakage of PMMA, such as high-viscosity cement,156–158 and composite membranes.159,160 Comprehending probable complications can optimize treatment options, innovate techniques, guide perioperative management, and provide a credible reference for researchers in the future.
3. Modification of PMMA to enhance the anti-tumor efficiency
As understood from the previous content of this article, various clinical manifestations or complications are primarily due to the residual tumor cells. Therefore, the eradication of residual tumor cells is the most essential issue. However, except for tumor cells in an extremely limited range that will be killed by the exothermic polymerization reaction of PMMA cement, residual tumor cells with strong proliferative capacity rapidly proliferate and sequentially erode the bone tissues. To improve this dilemma, effective strategies are adapted for modifying PMMA cement. As shown, the strategies of advanced modification and functional evaluation of PMMA cement are summarized in Table 2.
Table 2 Advanced modification and functional evaluation of PMMA cement
Modification strategies |
Types of drugs |
Mechanical properties |
Functional evaluation |
Ref. |
Drug carrier |
Simple drug delivery |
Cisplatin |
The mechanical properties of cement were not altered. |
The eluted cisplatin from PMMA exerts its cytotoxic action on Saos-2 cells, while the systemic concentration and the side effects are kept minimal |
45
|
DOX/pamidronate |
The cement modulus was not affected, but the strength varied based on the method and duration of storage. |
Anticipating the potential value of drug delivery in PMMA for appendicular and axial spinal applications |
161
|
Methotrexate |
The compressive strength of cement was not reduced at any of the concentrations of MTX. |
Confirming the MTX-supplemented cement can be used as an effective aid for the management of bone metastases |
162
|
Cisplatin/methotrexate |
— |
The drug concentration in bone could be maintained for 5 weeks without myelosuppression, hepatotoxicity, and nephrotoxicity in the experimental study of pigs |
163
|
Porogens |
PEG/SBA-15 |
Gentamicin |
The compressive strengths of PMMA cement with varied contents of SBA-15 were all between 80 MPa and 85 MPa, and the binding strength of the bone cement decreased sharply from 112 MPa to 39 MPa. |
A sustained drug release of up to seventy days can be achieved with sustained antibacterial activity but low toxicity to normal cells |
164
|
CMC/PLGA |
Colistin |
Modulation of the mechanical properties of PMMA cement by varying the amount of CMC incorporated, with decreasing mechanical properties as the CMC increases, is expected to provide support for craniomaxillofacial structures. |
Imparting PMMA cement porosity for sustained five-week drug release, but lacking bioassays to validate tumor suppression |
165
|
CMCS |
MTX |
The addition of 1.6% (w/w) CMCS significantly increased the flexural modulus, flexural strength, and compressive strength of MTX-PMMA by 5%, 2.8% and 5.2% respectively. |
CMCS enhances the killing effect of MTX on tumor cells while reducing the side effects associated with MTX, simultaneously guides tissue growth and satisfactory cement-bone union |
166
|
Gelatin |
Gentamicin |
After 14 d of immersion in PBS, the compressive and flexural properties of the bone cement were significantly reduced, effectively reducing the risk of adjacent vertebral fractures caused by PMMA bone cement. |
The release of GS in PMMA bone cement containing gelatine is 3.8 times higher than in conventional PMMA bone cement without gelatine, PMMA bone cement with 30% (w/w) gelatine maintains excellent antibacterial effect for at least 14 d in antibacterial tests |
167
|
PEG |
MTX |
— |
PEG porogen increases the elution of MTX and mobilizes large amounts of the drug into the surrounding environment |
168
|
γ-CD |
DOX |
The threshold of 70 MPa was exceeded after 48 hours for plain PMMA and PMMA/1.25 wt% free-DOX, while PMMA/15 wt% CD-DOX was slightly below this threshold (6.6 MPa). |
Over a period of 100 days, the composite PMMA/15 wt% CD-DOX released 100% of the initial DOX, while PMMA/1.25 wt% free-DOX released only 6% of the initial DOX; the ability to supplement additional doses of DOX after implantation contributes to the customization of personalized treatment programs. |
169
|
CMC |
Cisplatin |
The addition of CMC reduced the fracture toughness, while the modulus of elasticity was significantly decreased. |
The introduction of 3% CMC as a drug delivery vehicle and porogens for the sustained release of sufficient amounts of cisplatin and effective killing of osteosarcoma cells in vitro. |
170
|
Radioisotopes |
125I seeds |
— |
— |
Clinical efficacy can be enhanced by the combination of PVP and interstitial implantation of 125Iodine seeds. |
171
|
125I seeds |
— |
— |
The combination of PVP with 125Iodine seeds performs well in terms of rapid pain relief, improvement of daily living activities and the quality of patients’ lives. |
172
|
153Sm-EDTMP |
— |
— |
This procedure enhanced the anti-tumour potential for vertebrae with osseous metastasis without damaging adjacent tissues. |
173
|
Magnetic hyperthermia |
MNP |
Fe3O4 |
— |
PMMA cement containing 60 wt% of 100–500 nm Fe3O4 MNP still achieved approximately 90 MPa compressive strength. |
Bone cement samples containing 50 wt% MNP generates sufficient heat for the clinical application of bone tumors even in an alternating magnetic field of 120 Oe. |
174
|
Fe3O4 |
— |
— |
The tumor was completely ablated at a dose of 0.1 ml, 10% PMMA-Fe3O4, and a magnetic field exposure time of 180 s. |
175
|
Fe3O4 |
— |
— |
The feasibility of thermal therapy based on PMMA cement containing 22 wt% Fe3O4 was tested ex vivo in the beef vertebrae, and multi-cycle heating of PMMA-MNP was also verified. |
176
|
Silica-coated MNP |
— |
Both the mixing method and the percentage of filler affect the strength of PMMA cement. |
Silica-coated MNP imparts bioactivity and magnetism to PMMA cement, resulting in multifunctional stimulus-responsive composite bone cement for the treatment of bone tumors and related complications. |
177
|
Zn0.3Fe2.7O4 |
— |
Mixing the lower weight of MNPs with MMA monomer before mixing with PMMA powder can increase the mechanical strength of PMMA cement, providing reliable mechanical support. |
The biocompatibility of the cement was revealed by in vitro studies. In vivo investigations show that PMMA-Zn0.3Fe2.7O4-meditative magnetic hyperthermia locally inhibits bone metastasis and suppresses osteolytic bone resorption. |
178
|
TiO2/Fe3O4 |
— |
— |
Positive effects of TiO2 and Fe3O4 content on apatite formation capacity and heat production characteristics of PMMA cement. |
179
|
Fe3O4 |
DOX |
— |
DOX/Fe3O4@PMMA can be injected minimally invasively into the tumor, which not only causes significant tumor regression but also enhances the efficacy of oncological chemotherapy by triggering the rapid release of encapsulated DOX from the PMMA cement without side effects. |
46
|
Fe3O4 |
— |
The mechanical properties were all restored after injection of PMMA-6% Fe3O4 and its conversion to a solid and were not statistically different from the normal tibial plateau in ex vivo measurements. |
A novel, highly efficient, and minimally invasive method for complete bone tumor ablation and bone defect repair based on Fe3O4 NPs-containing PMMA magneto-thermal ablation. |
180
|
3.1. Drug carriers
Patients are routinely administered antineoplastic drugs after surgery to prevent residual tumor cells.181 However, systemic administration is associated with difficulties in achieving appropriate local (target) drug concentrations and the local efficacy is diminished.182,183
3.1.1 Simple drug delivery.
To accomplish elevated local drug concentrations and enhanced therapeutic efficacy, researchers have applied PMMA as a drug carrier to combat local tumor recurrence and adverse impacts attributed to systemic drug exposure.163 Such drug carriers are also regarded as drug delivery systems (DDS),184 which are more secure, advantageous, and effective. In the study by Kazutaka et al.,44 the anti-tumor effect even on lung metastases was employed using zoledronic acid (ZA)-loaded PMMA. This inspiration was initiated by the invention of the pioneering antibiotic-loaded PMMA cement as a measure to deliver a high local concentration of antibiotics to the peripheral tissues.185 The current research on antibiotic-loaded PMMA cement is much more advanced than anti-tumor treatment, so many research insights can be applied to anti-tumor treatment.186,187
Generally, PMMA powder is initially mixed with antineoplastic agents, including methotrexate (MTX), doxorubicin (DOX), ZA, and cisplatin, before being incorporated into the liquid component.45,161,163 On a few occasions, the drug was firstly compounded with the liquid component.188,189 Differences in drug elution efficiency exist between the two methods of mixing,190 furthermore, the elution efficiency of drugs is a distinctive determinant of effective tumor suppression. Therefore, Rosa et al.191 respectively incorporated three different chemotherapeutic agents into PMMA and further co-cultured them with tumor cells to measure the viability of the tumor cells in each group by MTT assay. Although each group of drugs had a significant inhibitory impact on tumor cells within 24 h, the viability of the tumor cells was recovered to nearly 100% at 15 days as the drug concentration decreased.191 Accordingly, inferencing that the major drawback of drug-loaded PMMA cement involves the compact structure and extremely low porosity of PMMA cement, only a bit of initially burst drug was released, and over 80–90% of the drug was contained within the cement and was incapable of being released.162 Tumor cells with tenacious proliferative capacity are incapable of being effectively inhibited for a long time by the few drugs released rapidly.191
3.1.2 Porogens.
To achieve an efficient rate of drug release, the intensive porosity of PMMA is critical. The connectivity of pores is constructed to provide pathways for drug release and even platforms for new bone growth by introducing porosity in PMMA cement.192 The anchoring effect of PMMA with bone will be enhanced if sufficient bone tissue is generated at the edge of PMMA and aseptic loosening will be prevented.193 Achieving this effect is indispensable for porogens, which are mostly composed of volatile or soluble substances,194 such as polyethylene glycol (PEG),164 poly(lactic-co-glycolic acid) (PLGA),165 carboxymethylcellulose (CMC),170 carboxymethyl chitosan (CMCS),166 and gelatin,167etc. However, porogens or large amounts of drugs could have deleterious effects on the mechanical properties of cement.195 Therefore, the mechanical strength of PMMA ought to be emphasized in the process of providing a continuous release of drugs.
By incorporating PEG into PMMA, it is possible to increase the MTX-loaded elution to obtain even 60% of the total amount of drugs. Although the changes in the mechanical properties of cement were not introduced in the article, they were presumably seriously weakened.168 Improved mechanical properties could be presented by CMCS-modified PMMA; CMCS is a natural polysaccharide with water solubility and is associated with anti-tumor activity, according to research.196,197 Liu et al.198 incorporated MTX and CMCS into PMMA-fabricated composite cement, providing a DDS with intensive drug release and more persistence than pure PMMA. Notably, the mechanical properties of the cement, as well as implant-bone integration were improved, and the possibility of secondary fracture was subsequently decreased. Moreover, a lower polymerization temperature and prolonged setting time of CMC-modified PMMA are well accepted for clinical application by surgeons.170 During mixing, the hydrophilic CMC hydrogel was dispersed throughout the hydrophobic PMMA and left voids to form pores after aqueous CMC drying, creating 34–35% open porosity (Fig. 4). Nevertheless, 80% of the total loading drugs remained entrapped in cement, which was attributed to the hydrophobic nature of PMMA resulting in body fluid not penetrating PMMA to release the drugs. Therefore, some studies have indicated that PMMA particles modified with ovalbumin exhibited improved biocompatibility and hydrophilicity.184 In addition, the refilling of chemotherapeutics can be achieved by a PMMA-based DDS modified with insoluble γ-cyclodextrin (γ-CD) polymeric microparticles (a cyclic oligosaccharide), which can thermodynamically entrap and release DOX due to their binding strength and affinity.199 PMMA/γ-CD composite cement served as the first chemotherapeutic delivery system capable of refilling and consistently releasing DOX with sufficient mechanical properties, achieving the excellent effect of the inhibition of tumor cells. Therefore, a more pioneering opportunity for the modification of dense PMMA cement will be provided by continuous innovation and the development of porogens.
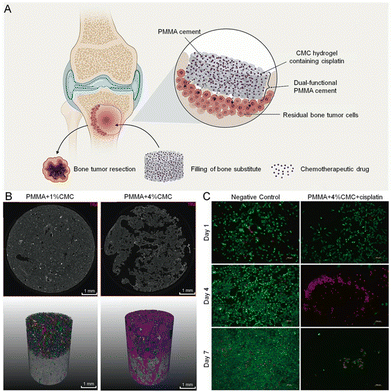 |
| Fig. 4 Schematic representation of PMMA cement modified with porogens for the treatment of bone tumors. (A) Schematic illustration showing how dual-functional porous and cisplatin-loaded PMMA cement for the reconstruction of load-bearing bone defect kills bone tumor cells. (B) 2D micro-CT X-ray images of transversal cross-sections and 3D reconstructions of PMMA-based cement with different CMC content. The colorful shapes above indicate substantial porosity in the porous PMMA specimens. (C) Cytotoxic effects of 4% CMC-containing cisplatin-loaded PMMA cement release assessed by live/dead staining. Reproduced with permission.170 Copyright 2022, Elsevier. | |
3.1.3 Radioisotopes.
Radiotherapy is frequently applied for bone tumors,200 and greater efficacy will be achieved with the interstitial implantation of radioactive seeds.171 PVP combined with 125Iodine seed implantation in the treatment of spinal metastases has manifested a considerable therapeutic effect,172 reducing its side effects and simultaneously enhancing its anti-tumor activity to target metastasis. In addition, discrepant effects will be presented by different radioisotopes due to their respective physical and biological properties.201 Lu et al.173 incorporated samarium-153-ethylenediamine tetramethylene phosphonate (153Sm-EDTMP) into PMMA, injecting it into the vertebrae of dogs to investigate its safety and feasibility. The anti-tumor activity would be observably intensified without damaging adjacent tissues when the dose of 153Sm-EDTMP is lower than 70 mCi.
3.2. Magnetic hyperthermia
Hyperthermia involves generating temperatures generally between 41–46 °C and eradicating tumor cells sensitive to temperature without adverse effects.202 Magnetic hyperthermia, one of the types of hyperthermia, produces heat via magnetic nanoparticles (MNP) when exposed to the AMF.203 Based on this property, the incorporation of MNPs into PMMA has been investigated for the magnetic thermal therapy of bone tumors, providing reliable multifunctional therapeutic effects. The magnetic hyperthermia treatment has the following fundamental advantages when treating bone tumors locally: (1) the AMF penetrates deeper than any other activation mechanism (light or acoustic), allowing deeper tissue to be reached; (2) the nanoscale size drives the magnetic properties that determine the heating capacity.204 Notably, PMMA modified by different MNPs exhibits discrepancies, including oxides or alloys of magnetic elements such as iron, cobalt, and nickel.202,205 A composite PMMA cement incorporating magnetite-containing bioactive glass-ceramic exhibited thermal effects while still maintaining desirable mechanical properties without modulating the morphological, mechanical, and calorimetric characterization.206
Fe3O4 MNP is capable of generating effective magnetic thermal properties via the application of a controllable external AMF.207,208 However, the properties of Fe3O4-incorporated PMMA deserve to be further verified. Kawashita et al.174 first comprehensively investigated the magnetic thermal ability, compressive property, setting time, and maximum temperature during the setting of Fe3O4-incorporated PMMA with different concentrations, showing the feasibility of Fe3O4-modified PMMA as a magnetic cement, but only in vitro. In subsequent experiments by Ling et al.175 Fe3O4-modified PMMA was applied to kill subcutaneous tumors in vivo through magnetic thermal treatment, showing a considerable anti-tumor effect. The temperature was mildly increased to simulate the clinical procedure in the spine.176 In addition, a positive correlation between the coagulation necrosis area of tumor tissue and the concentrations of Fe3O4 was indicated175 but the potential toxicity of Fe3O4 should not be ignored.209 Therefore, the quantity and dimensions of Fe3O4 MNP are crucial factors in the regulation of magnetic thermal capacity as well as possible cytotoxicity to tumor cells.210,211 The biosafety of PMMA-6% Fe3O4 presented no abnormal indicators regarding heart function, liver function, kidney function, and blood tests. In addition, excellent mechanical support was provided while simultaneously exhibiting the ideal magnetic thermal killing effect after minimally invasive injection into tibial tumor defects in vivo (Fig. 5).180 Such magnetic thermal cement can also be applied as the DDS for synergistic magnetic thermal ablation and chemotherapy, which was achieved by a Fe3O4-modified PMMA mixed with DOX (DOX/Fe3O4@PMMA).46 Excellent magnetic thermal properties and also the controllable release of DOX stimulated by AFM would be accomplished by DOX/Fe3O4@PMMA and without the presence of potential side effects. This novel cement is promising for clinical application in the efficient therapy of bone tumors.
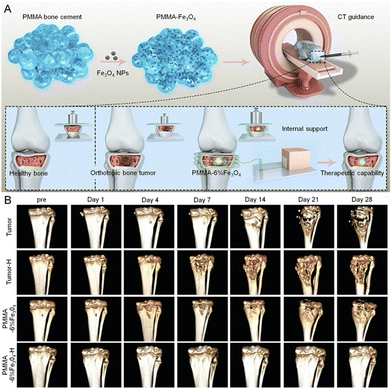 |
| Fig. 5 Schematic representation of magnetothermal PMMA cement for the treatment of bone tumors. (A) Schematic illustration of PMMA-Fe3O4 cement for internal mechanical support and magnetic thermal ablation of bone tumors. (B) 3D-reconstructed CT images at each follow-up time point, the volume of bone defects in the PMMA-6%Fe3O4-H group increased slightly after the 28-day follow-up. Reproduced with permission.180 Copyright 2019, Ivyspring International Publisher. | |
In addition to Fe3O4, the reasonable addition of components will lead to cement with other aspects of improvement. Bone defects caused by bone tumors frequently pose intractable problems for treatment and PMMA cement with TiO2 can eliminate this problem.179 PMMA cement containing 15% or more TiO2 and 25% or more Fe3O4 has high bone affinity, forming apatite on their surfaces within 14 days in a simulated body fluid. This modified strategy provides a practical treatment option for patients with tumor-derived bone defects. Ren et al.178 synthesized a novel composite PMMA consisting of Zn and Fe, which minimally affected the physicochemical properties of the cement, according to the requirements of clinical transformation. To further enhance the biological activity, silica-coated MNPs were fabricated for incorporation into PMMA.177 The bioactive behavior was derived from the existence of Si-OH groups due to the silica shell, facilitating apatite nucleation.
In brief, enhancing the effectiveness of other treatments (radiotherapy, chemotherapy, immunotherapy, etc.) without increasing the toxicity is the most prominent contribution of thermotherapy, so the subsequent clinical application of magnetic thermal cement has a broader prospect. However, restricted by the magnetic field generator's fabrication technology and equipment requirements, the development of magnetic thermal cement remains to be explored and researched in a multidisciplinary manner.
4. Conclusion
The complex pathological mechanism of bone tumors not only presents severe challenges to patients but also perplexes surgeons. Patients are loath to tolerate persistent torment derived from bone tumors, including pain, neurological signs, bone instability, and tendencies to relapse. However, the current primary clinical treatment is insufficient, subjecting the patient to the restrictions of curettage, large bone defects, persistent pain, and high recurrence rates. Fortunately, as a commercial biomaterial, PMMA has the potential to address these issues based on the advantages of being plastic, injectable, and its prominent mechanical properties.
The margin of bone defects originating from osteolytic tumors or the curettage of tumors can be perfectly filled by PMMA cement with plasticity, providing stable mechanical support. Moreover, the residual tumor cells and eroded nociceptive nerve endings will be killed by the heat and chemically toxic substances generated from the exothermic polymerization reaction of PMMA. The pain felt by patients and the risk of recurrence will be reduced but there is no denying that the issue regarding whether PMMA can reduce the tumor recurrence rate is controversial, and additional complications will be certainly generated from the exothermic polymerization reaction of PMMA. Fortunately, researchers have gained extensive experience from previous failures due to medical negligence, and they avoid the occurrence of similar complications as much as possible to provide more dependable operational experience in the future. Notably, many projects for PMMA modification were also supposed to resolve the fundamental trouble caused by residual tumor cells. Whether PMMA is modified to DDS or magnetic thermal cement, residual tumor cells can be effectively eradicated on the premise of the invariable bottommost compressive properties and physicochemical properties of cement. Therefore, these projects offer progressive prospects for optimizing the treatment options and alleviating symptoms in patients in the future.
5. Prospects
The effectiveness of PMMA cement in the treatment of bone tumors deserves some appreciation and despite some complications, there are considerable prospects with the appropriate modifications. Although the stability of small bone defects can be reconstructed by PMMA cement, the ability is disputed when disposing of large defects.212 Implant looseness and failure are frequently attributed to the intrinsic cytotoxicity and bioinertness of PMMA.213,214 Inorganic bone cement is less toxic and more biocompatible but has not been extensively applied in bone tumor treatment.215 Therefore, some researchers have incorporated inorganic cement or bioactive glasses into PMMA cement, accomplishing the improved modification of composite cement with excellent polymerization temperature, biocompatibility, and compressive properties.216 Most significantly, the current work indicates that incorporating inorganic cement or bioactive glasses into PMMA cement facilitates pore construction, which is potentially adjunctive to the release of chemotherapeutic agents. The optimal mixing ratios are requisite for further research and continuous improvement and simultaneously, better biocompatibility will be demonstrated by mixing some additives in PMMA cement that are conducive to repairing tumor-derived bone defects.
PMMA cement loaded with drugs has considerable anti-tumor efficacy and conditions that damage healthy bone tissue are not unusual due to the rapid and massive release of drugs.188 To construct a reasonable DDS based on PMMA cement, the particle size and concentration of diverse antineoplastic agents should be rigorously investigated. It is worth noting that alendronate, a prevalent anti-tumor drug incorporated into PMMA cement as a drug carrier can, in combination with the tumor microenvironmental response, block the exchange of substances between the tumor and the surrounding normal tissues.217 This is a promising osmotic anti-tumor approach.218 Immunotherapy is also included as it is involved in most physiological and pathological processes in the body.219,220 In addition, the sustained release effect of drugs can be achieved by porogen-modified PMMA cement, averting damage to healthy bone tissue and inhibiting residual tumor cells. However, current research on porogen-modified PMMA cement is inadequate for providing a reliable DDS for the clinical treatment of bone tumors. A type of PMMA cement modified by a specific porogen acts as a sustained drug release platform with the addition of replaceable drugs to achieve different therapeutic effects. In addition, because the most prominent contribution of hyperthermia is enhancing the effectiveness of other treatments (radiotherapy, chemotherapy, immunotherapy, etc.) without increasing toxicity, a broad horizon for the efficient treatment of bone tumors is created by the development of magnetic thermal cement. At present, except for Fe3O4 MNP-modified PMMA cement, other types of MNP are few. The development of novel MNPs to modify PMMA cement could perhaps attain unexpected improvements. Nevertheless, the magnetic field generator's fabrication technology and equipment requirements consistently bewilder researchers. Photothermal therapy is also an optimal treatment for hyperthermia without requirements as stringent as magnetic thermal requirements, but the current research on photothermal PMMA cement applied to bone tumors is very sparse. In summary, PMMA cement plays an essential role in bone tumor treatment, with irreplaceable advantages and can be modified to achieve better therapeutic effects in the future.
Author contributions
Contributions: (I) conceptualization: Bo Chao, Zhonghan Wang, and Minfei Wu; (II) writing – original draft: Bo Chao; (III) funding acquisition: Jianhang Jiao, Zhonghan Wang, and Minfei Wu; (IV) writing – review & editing: Bo Chao, Jianhang Jiao, Lili Yang, Yang Wang, Tong Yu, He Liu, Han Zhang, Mufeng Li, Wenjie Wang, Xiangran Cui, Shangyu Du, Zhonghan Wang, and Minfei Wu.
Conflicts of interest
There is no conflict of interest to declare.
Acknowledgements
This work was supported by the Department of Science and Technology of Jilin Province (20210204104YY, YDZJ202201ZYTS135, YDZJ202301ZYTS031, YDZJ202301ZYTS032), and Bethune Plan of Jilin University (Grant No. 2023B08, 2023B10).
References
- H. Sung, J. Ferlay, R. L. Siegel, M. Laversanne, I. Soerjomataram, A. Jemal and F. Bray, Ca-Cancer J. Clin., 2021, 71, 209–249 CrossRef PubMed.
- F. Verrecchia and F. Redini, Front. Oncol., 2018, 8, 133 CrossRef PubMed.
- S. De Salvo, V. Pavone, S. Coco, E. Dell’Agli, C. Blatti and G. Testa, J. Clin. Med., 2022, 11, 699 CrossRef PubMed.
- W. J. Anderson and L. A. Doyle, Histopathology, 2021, 78, 644–657 CrossRef PubMed.
- P. S. Steeg, Nat. Rev. Cancer, 2016, 16, 201–218 CrossRef CAS PubMed.
- J. S. Whelan and L. E. Davis, J. Clin. Oncol., 2018, 36, 188–193, DOI:10.1200/JCO.2017.75.1743.
- H. M. Zakaria, J. T. Llaniguez, E. Telemi, M. Chuang, M. Abouelleil, B. Wilkinson, A. Chandra, D. Boyce-Fappiano, E. Elibe, L. Schultz, F. Siddiqui, B. Griffith, S. N. Kalkanis, I. Y. Lee and V. Chang, Neurosurgery, 2020, 86, 705–716 CrossRef PubMed.
- L. van der Heijden, P. D. S. Dijkstra, J. Y. Blay and H. Gelderblom, Eur. J. Cancer, 2017, 77, 75–83 CrossRef PubMed.
- H. Li, J. Gao, Y. Gao, N. Lin, M. Zheng and Z. Ye, Front. Oncol., 2020, 10, 580–605 CrossRef PubMed.
- J. M. Argiles, S. Busquets, B. Stemmler and F. J. Lopez-Soriano, Nat. Rev. Cancer, 2014, 14, 754–762 CrossRef CAS PubMed.
- S. D’Oronzo, R. Coleman, J. Brown and F. Silvestris, J. Bone Oncol., 2019, 15, 100205 CrossRef PubMed.
- D. M. Sciubba, R. J. Petteys, M. B. Dekutoski, C. G. Fisher, M. G. Fehlings, S. L. Ondra, L. D. Rhines and Z. L. Gokaslan, J. Neurosurg. Spine, 2010, 13, 94–108 Search PubMed.
- J. Gill and R. Gorlick, Nat. Rev. Clin. Oncol., 2021, 18, 609–624 CrossRef PubMed.
- M. U. Jawad, B. H. Pollock, E. Alvarez, J. R. Carr-Ascher, R. L. Randall and S. W. Thorpe, Ann. Surg. Oncol., 2022, 29, 4363–4372 CrossRef PubMed.
- T. Winkler, F. A. Sass, G. N. Duda and K. Schmidt-Bleek, Bone Joint Res., 2018, 7, 232–243 CrossRef CAS PubMed.
- B. W. Tan, Q. Tang, Y. J. Zhong, Y. L. Wei, L. F. He, Y. T. Wu, J. B. Wu and J. F. Liao, Int. J. Oral Sci., 2021, 13, 9 CrossRef CAS PubMed.
- J. Liao, K. Shi, Y. Jia, Y. Wu and Z. Qian, Bioact. Mater., 2021, 6, 2221–2230 CrossRef CAS PubMed.
- F. Wu, J. Xu, M. Jin, X. Jiang, J. Li, X. Li, Z. Chen, J. Nie, Z. Meng and G. Wang, Front. Mol. Biosci., 2022, 8, 705148 CrossRef PubMed.
- H. K. Brown, M. Tellez-Gabriel and D. Heymann, Cancer Lett., 2017, 386, 189–195 CrossRef CAS PubMed.
- X. Xue, H. Qu and Y. Li, Exploration, 2022, 2, 20210134 CrossRef PubMed.
- J. Garnon, L. Meylheuc, R. L. Cazzato, D. Dalili, G. Koch, P. Auloge, B. Bayle and A. Gangi, Diagn. Interv. Imaging, 2019, 100, 743–752 CrossRef CAS PubMed.
- J. K. Liu, R. I. Apfelbaum, B. W. Chiles III and M. H. Schmidt, Neurosurg. Focus, 2003, 15, E2–E2 Search PubMed.
- T. A. G. van Vugt, J. J. Arts and J. A. P. Geurts, Front. Microbiol., 2019, 10, 1626 CrossRef PubMed.
- T. Jaeblon, JAAOS – J. Am. Acad. Orthop. Surg., 2010, 18, 297–305 CrossRef PubMed.
- K. D. Kuehn, W. Ege and U. Gopp, Orthop. Clin. North Am., 2005, 36, 17–28 CrossRef PubMed.
- E. C. Krishnan, C. Nelson and J. R. Neff, Med. Phys., 1986, 13, 233–239 CrossRef CAS PubMed.
- D. Gundapaneni and T. Goswami, J. Appl. Biomater. Biomech., 2014, 12, 193–202 Search PubMed.
- A. Gangi and X. Buy, Semin. Interventional Radiol., 2010, 27, 124–136 CrossRef PubMed.
- E. B. Dolan, M. G. Haugh, D. Tallon, C. Casey and L. M. McNamara, J. R. Soc., Interface, 2012, 9, 3503–3513 CrossRef CAS PubMed.
- G. W. Froschle, J. Mahlitz, H. U. Langendorff, E. Achilles, J. Pollock and K. H. Jungbluth, Anticancer Res., 1997, 17, 995–1002 CAS.
- Y. i Kim, H. G. Kang, J. H. Kim, S. k Kim, P. P. Lin and H. S. Kim, Bone Joint J. B, 2016, 98, 703–709 CrossRef PubMed.
- A. Balestrino, S. Boriani, R. Cecchinato, A. Parafioriti, M. Gambarotti and A. Gasbarrini, Eur. Spine J., 2020, 29, 3157–3162 CrossRef PubMed.
- R. P. Bhide, A. Barman, S. M. Varghese, A. Chatterjee, S. Mammen, J. George and R. Thomas, Am. J. Phys. Med. Rehabil., 2014, 93, 431–436 CrossRef PubMed.
- S. Ramanathan, T. Vora, A. Gulia, A. Mahajan and S. Desai, Skeletal Radiology, 2017, 46, 715–718 CrossRef PubMed.
- I. Tran, U. Gerckens, J. Remig, G. Zintl and J. Textor, Spine (Phila Pa 1976), 2013, 38, E316–318 CrossRef PubMed.
- M. Nagae, Y. Mikami, K. Mizuno, T. Harada, T. Ikeda, H. Tonomura, R. Takatori, H. Fujiwara and T. Kubo, Medicine, 2016, 95, e5178 CrossRef PubMed.
- P. C. Gerszten and E. A. Monaco III, Neurosurg. Focus, 2009, 27, E9 Search PubMed.
- P. C. Gerszten and W. C. Welch, J. Neurosurg. Spine, 2007, 6, 92–95 Search PubMed.
- L. van der Heijden, M. A. J. van de Sande, A. C. Heineken, M. Fiocco, R. G. H. H. Nelissen and P. D. S. Dijkstra, J. Bone Jt. Surg., Am. Vol., 2013, 95, e159 CrossRef PubMed.
- Y. Araki, N. Yamamoto, K. Hayashi, A. Takeuchi, S. Miwa, K. Igarashi, Y. Taniguchi, H. Yonezawa, S. Morinaga and H. Tsuchiya, JB JS open access, 2020, 5, e19.00068 CrossRef PubMed.
- M.-P. Henrichs, J. Krebs, G. Gosheger, A. Streitbuerger, M. Nottrott, T. Sauer, S. Hoell, G. Singh and J. Hardes, World J. Surg. Oncol., 2014, 12, 330 CrossRef PubMed.
- H. M. Wang, C. S. Galasko, S. Crank, G. Oliver and C. A. Ward, Clin. Orthop. Relat. Res., 1995, 173–186 Search PubMed.
- F. Greco, L. de Palma, N. Specchia, S. Jacobelli and C. Gaggini, Orthopedics, 1992, 15, 189–194 CrossRef CAS PubMed.
- K. Koto, H. Murata, Y. Sawai, E. Ashihara, M. Horii and T. Kubo, Oncol. Lett., 2017, 14, 1648–1656 CrossRef PubMed.
- H. Oezben, L. Eralp, G. Baysal, A. Cort, N. Sarkalkan and T. Ozben, Acta Orthop. Et Traumatologica Turcica, 2013, 47, 184–192 CrossRef PubMed.
- B. Liang, D. Zuo, K. Yu, X. Cai, B. Qiao, R. Deng, J. Yang, L. Chu, Z. Deng, Y. Zheng and G. Zuo, Mater. Sci. Eng., C, 2020, 108, 110460 CrossRef CAS PubMed.
- F. De Tommasi, C. Massaroni, R. F. Grasso, M. Carassiti and E. Schena, Sensors, 2021, 21, 5470 CrossRef CAS PubMed.
- Z. Huang, Z. Tian, M. Zhu, C. Wu and Y. Zhu, Adv. Ther., 2021, 4, 2000212 CrossRef CAS.
- M. Miola, A. Bellare, R. Gerbaldo, F. Laviano and E. Verne, Ceram. Int., 2021, 47, 17633–17643 CrossRef CAS.
- N. Alcorta-Sevillano, I. Macías, A. Infante and C. I. Rodríguez, Cells, 2020, 9, 2630 CrossRef CAS PubMed.
- Y. L. Xia, H. Y. Wang, Y. H. Li and C. F. Fu, Front. Mater., 2022, 9, 929618 CrossRef.
- F. Migliorini, G. La Padula, E. Torsiello, F. Spiezia, F. Oliva and N. Maffulli, Eur. J. Med. Res., 2021, 26, 118 CrossRef PubMed.
- J. G. Seiler III and J. Johnson, J. South. Orthop. Assoc., 2000, 9, 91–97 Search PubMed.
- Y. Gong, B. Zhang and L. Yan, Front. Mater., 2022, 9, 912713 CrossRef.
- G. Szczesny, M. Kopec, D. J. Politis, Z. L. Kowalewski, A. Lazarski and T. Szolc, Materials, 2022, 15, 3622 CrossRef CAS PubMed.
- W. B. Jacobs and R. G. Perrin, Neurosurg. Focus, 2001, 11, e10 CAS.
- T. Mrozek, J. Spindel, L. Miszczyk, B. Koczy, A. Chrobok, B. Pilecki, P. Tomasik and J. Matysiakiewicz, Ortopedia, Traumatologia, Rehabilitacja, 2005, 7, 481–485 Search PubMed.
- W. B. Scoville, A. H. Palmer, K. Samra and G. Chong, J. Neurosurg., 1967, 27, 274–279 CAS.
- K. M. I. Salem and C. G. Fisher, Eur. Spine J., 2016, 25, 3916–3922 CrossRef PubMed.
- S. T. Sorensen, A. O. Kirkegaard, L. Carreon, R. Rousing and M. O. Andersen, Spine J., 2019, 19, 1067–1075 CrossRef PubMed.
- M. Stangenberg, L. Viezens, S. O. Eicker, M. Mohme, K. C. Mende and M. Dreimann, Neurosurg. Focus, 2017, 43, E3 Search PubMed.
- J. Wong, E. Chow, E. de Sa, C. Rowsell, L. Probyn, M. Christakis, E. Sinclair, C. Law and J. Finkelstein, J. Palliative Med., 2009, 12, 97–100 CrossRef PubMed.
- G. Shi, F. Feng, C. Hao, J. Pu, B. Li and H. Tang, J. Int. Med. Res., 2020, 48 DOI:10.1177/0300060519835084.
- J. S. Jang, S. H. Lee, C. H. Rhee and S. H. Lee, J. Neurosurg., 2002, 96, 131–134 Search PubMed.
- J.-w Tan, B.-h Shen, W. Du, J.-q Liu and S.-q Lu, Chin. Med. J., 2013, 126, 2495–2498 Search PubMed.
- F. Tao, Z. Shi, H. Tao, A. Wei, H. Tao, H. Cao, Y. Zhao, Y. Zhang and W. Xiang, Br. J. Neurosurg., 2020, 34, 308–312 CrossRef PubMed.
- C. I. Cady-McCrea, J. C. Gilbert and M. A. Galgano, World Neurosurg., 2021, 152, 162–166 CrossRef PubMed.
- R. L. Cazzato, J. Garnon, D. Dalili, P. A. Autrusseau, P. Auloge, P. De Marini, X. Buy, J. Palussiere and A. Gangi, Tech. Vasc. Interventional Radiol., 2022, 25, 00803 Search PubMed.
- M. T. Wallace and R. M. Henshaw, J. Pediatr. Orthop., 2014, 34, 92–100 CrossRef PubMed.
- Y. Panchwagh, A. Puri, M. Agarwal, C. Anchan and M. Shah, Indian J. Orthop., 2007, 41, 139–145 CrossRef PubMed.
- S. P. Gupta and G. Garg, J. Orthop. Traumatol., 2016, 17, 239–247 CrossRef PubMed.
- A. F. Mavrogenis, V. G. Igoumenou, P. D. Megaloikonomos, G. N. Panagopoulos, P. J. Papagelopoulos and P. N. Soucacos, Sicot-J, 2017, 3, 54 CrossRef PubMed.
- J. Segura, J. Albareda, A. L. Bueno, A. Nuez, D. Palanca and F. Seral, La Chirurgia degli organi di movimento, 1997, 82, 373–380 CAS.
- Y. Song, C.-F. Li, X.-T. Shi, Y.-Q. Cheng, H.-Q. Suo and J.-G. Liu, China J. Orthop. Traumatol., 2019, 32, 372–376 Search PubMed.
- Q. Zhang, Y. Cai, X. Niu and L. Hao, Chin. J. Surgery, 1999, 37, 730–732 CAS.
- A. Zylberberg, G. Bayley, L. Gala and P. R. Kim, Case Rep. Orthop., 2015, 283294 Search PubMed.
- S. Tsukamoto, A. F. Mavrogenis, M. Akahane, K. Honoki, A. Kido, Y. Tanaka, D. M. Donati and C. Errani, BMC Musculoskeletal Disord., 2022, 23, 477 CrossRef CAS PubMed.
- M. Wu, S. Yao, Y. Xie, F. Yan, Z. Deng, J. Lei and L. Cai, Medicine, 2018, 97, e13154 CrossRef PubMed.
- G. Guzik, J. Orthop. Surg. Res., 2016, 11, 54 CrossRef PubMed.
- N. Sethakorn, E. Heninger, C. Sanchez-de-Diego, A. Ding, R. C. Yada, S. C. Kerr, D. Kosoff, D. J. Beebe and J. S. M. Lang, Cancers, 2022, 14, 757 CrossRef CAS PubMed.
- R. E. Coleman, P. I. Croucher, A. R. Padhani, P. Clezardin, E. Chow, M. Fallon, T. Guise, S. Colangeli, R. Capanna and L. Costa, Nat. Rev. Dis. Primers, 2020, 6, 83 CrossRef PubMed.
- P. Romero-Morelos, E. Ruvalcaba-Paredes, D. Garciadiego-Cazares, M. PerezSantos, S. Reyes-Long, A. Alfaro-Rodriguez, M. Salcedo, J. Mancilla-Ramirez and C. Bandala, Curr. Neuropharmacol., 2021, 19, 308–319 CrossRef CAS PubMed.
- P. Mantyh, Pain, 2013, 154, S54–S62 CrossRef PubMed.
- N. Figura, J. Smith and H. H. M. Yu, Hematol./Oncol. Clin. North Am., 2018, 32, 447–458 CrossRef PubMed.
- R. E. Coleman, Cancer Treat. Rev., 2001, 27, 165–176 CrossRef CAS PubMed.
- T. F. Jakobs, C. Trumm, M. Reiser and R. T. Hoffmann, Eur. Radiol., 2007, 17, 2166–2175 CrossRef CAS PubMed.
- B. Roedel, F. Clarencon, S. Touraine, E. Cormier, L. Molet-Benhamou, L. Le Jean, H. Brisse, S. Neuenschwander and J. Chiras, J. Neuroradiol., 2015, 42, 222–228 CrossRef PubMed.
- L. Prié, P. Lagarde, J. Palussière, S. el Ayoubi, J. M. Dilhuydy, M. Durand, J. M. Vital and G. Kantor, Cancer Radiother., 1997, 1, 234–239 CrossRef PubMed.
- H. A. Gilbert, A. R. Kagan, H. Nussbaum, A. R. Rao, J. Satzman, P. Chan, B. Allen and A. Forsythe, AJR. Am. J. Roentgenol., 1977, 129, 1095–1096 CrossRef CAS PubMed.
- O. Togao, F. Mihara, T. Yoshiura, T. Noguchi, Y. Kuwabara, K. Yoshimitsu and H. Honda, Fukuoka igaku zasshi Hukuoka acta medica, 2005, 96, 93–99 Search PubMed.
- Y.-Y. Tseng, Y.-L. Lo, L.-H. Chen, P.-L. Lai and S.-T. Yang, Surg. Neurol., 2008, 70, 78–84 CrossRef PubMed.
- P. Xie, Y. Zhao and G. Li, Clin. Neurol. Neurosurg., 2015, 138, 157–161 CrossRef PubMed.
- L. Alvarez, A. Perez-Higueras, D. Quinones, E. Calvo and R. E. Rossi, Eur. Spine J., 2003, 12, 356–360 CrossRef CAS PubMed.
- Y. Y. Tseng, S. T. Yang, P. H. Tu, T. C. Yang and Y. L. Lo, Chin. J. Minimally Invasive Neurosurg., 2008, 51, 280–284 CrossRef PubMed.
- J. S. Jang and S. H. Lee, J. Neurosurg. Spine, 2005, 2, 243–248 Search PubMed.
- K. J. Murphy and H. Deramond, Neuroimaging Clin. N. Am., 2000, 10, 535–545 CAS.
- A. Cotten, N. Boutry, B. Cortet, R. Assaker, X. Demondion, D. Leblond, P. Chastanet, B. Duquesnoy and H. Deramond, Radiographics, 1998, 18, 311–320 CrossRef CAS PubMed ; discussion 320-313.
- G. Saliou, E. M. Kocheida, P. Lehmann, C. Depriester, G. Paradot, D. Le Gars, A. Balut and H. Deramond, Radiology, 2010, 254, 882–890 CrossRef PubMed.
- G. Sun, L. Li, P. Jin, X. W. Liu and M. Li, J. Surg. Oncol., 2014, 110, 123–128 CrossRef PubMed.
- G. Sun, P. Jin, M. Li, Y. Lu, X. Liu, F. Li, Z. Xie, J. Ding and Z. Peng, Technol. Cancer Res. Treat., 2011, 10, 267–274 CrossRef CAS PubMed.
- Y. Li, Y. F. Gu, Z. K. Sun, C. G. Wu, Y. D. Li, W. Wang, Y. C. Chen and J. Lu, Eur. Radiol., 2013, 23, 2754–2763 CrossRef PubMed.
- J. H. Woo, H. S. Park, J. I. Han and D. Y. Kim, Pain Physician, 2013, 16, E405–E410 Search PubMed.
- J. W. Park, H.-j Lim, H. G. Kang, J. H. Kim and H.-S. Kim, Ann. Surg. Oncol., 2022, 29, 1413–1422 CrossRef PubMed.
- I. Laufer, A. Hanover, E. Lis, Y. Yamada and M. Bilsky, J. Neurosurg. Spine, 2010, 13, 109–115 Search PubMed.
- D. Lau, M. R. Leach, F. La Marca and P. Park, J. Neurosurg. Spine, 2012, 17, 565–576 Search PubMed.
- M. Campanacci, N. Baldini, S. Boriani and A. Sudanese, J. Bone Jt. Surg. Am., 1987, 69, 106–114 CrossRef CAS.
- A. H. Kivioja, C. Blomqvist, K. Hietaniemi, C. Trovik, A. Walloe, H. C. Bauer, P. H. Jorgensen, P. Bergh and G. Follerås, Acta Orthop., 2008, 79, 86–93 CrossRef PubMed.
- W. T. Becker, J. Dohle, L. Bernd, A. Braun, M. Cserhati, A. Enderle, L. Hovy, Z. Matejovsky, M. Szendroi, K. Trieb and P. U. Tunn, J. Bone Jt. Surg Am., 2008, 90, 1060–1067 CrossRef PubMed.
- D. Zuo, L. Zheng, W. Sun, D. Fu, Y. Hua and Z. Cai, World J. Surg. Oncol., 2013, 11, 148 CrossRef PubMed.
- M. Balke, H. Ahrens, A. Streitbuerger, G. Koehler, W. Winkelmann, G. Gosheger and J. Hardes, J. Cancer Res. Clin. Oncol., 2009, 135, 149–158 CrossRef PubMed.
- M. Balke, L. Schremper, C. Gebert, H. Ahrens, A. Streitbuerger, G. Koehler, J. Hardes and G. Gosheger, J. Cancer Res. Clin. Oncol., 2008, 134, 969–978 CrossRef CAS PubMed.
- P. H. Vora, R. Musa, N. M. Bhavsar and D. Shah, J. Orthop. Case Rep., 2017, 7, 68–71 Search PubMed.
- O. Ofluoglu, Acta Orthop. Belg., 2008, 74, 831–836 Search PubMed.
- E. Sirin, A. H. Akgulle, O. M. Topkar, O. Sofulu, S. E. Baykan and B. Erol, Acta Orthop. Et Traumatol. Turcica, 2020, 54, 524–529 CrossRef PubMed.
- N. G. Harness and H. J. Mankin, J. Hand Surg. Am., 2004, 29, 188–193 CrossRef PubMed.
- Y.-p Liu, K.-h Li and B.-h Sun, Clin. Orthop. Related Res.®, 2012, 470, 2886–2894 CrossRef PubMed.
- J. Bickels and D. A. Campanacci, J. Bone Jt. Surg. Am. Vol., 2020, 102, 164–174 CrossRef PubMed.
- C. Errani, P. Ruggieri, M. A. N. Asenzio, A. Toscano, S. Colangeli, E. Rimondi, G. Rossi, A. Longhi and M. Mercuri, Cancer Treat. Rev., 2010, 36, 1–7 CrossRef PubMed.
- T. Ozalp, H. Yercan, G. Okçu, O. Ozdemir and E. Coşkunol, Acta Orthop. Traumatol. Turc, 2006, 40, 144–150 Search PubMed.
- W. Becker, Z. Orthop. Ihre Grenzgeb, 1989, 127, 379–381 CrossRef CAS PubMed.
- T. Wada, M. Kaya, S. Nagoya, S. Kawaguchi, K. Isu, T. Yamashita, S. Yamawaki and S. Ishii, J. Orthop. Sci., 2002, 7, 194–198 CrossRef PubMed.
- S. Yevich, L. Tselikas, G. Gravel, T. de Baere and F. Deschamps, Semin. Interventional Radiol., 2018, 35, 268–280 CrossRef PubMed.
- D. A. Nelson, M. E. Barker and B. H. Hamlin, Int. J. Hyperthermia, 1997, 13, 287–306 CrossRef CAS PubMed.
- S. Aydin, E. Bozdag, E. Sunbuloglu, H. Unalan, M. Hanci, O. Aydingoz and C. Kuday, Eur. Spine J., 2006, 15, 341–346 CrossRef PubMed.
- B. R. Radev, J. A. Kase, M. J. Askew and S. D. Weiner, J. Biomech., 2009, 42, 1120–1126 CrossRef PubMed.
- B. Bombardier, D. Haase, K. Sweeney, E. Friedman, T. Poppe and N. Hughes, J. Surg. Oncology, 2021, 123, 1299–1303 CrossRef CAS PubMed.
- D. Clarke, D. Nepaul, H. Chindepalli and K. Lawson, West Indian Med. J., 2018, 67, 148–152 Search PubMed.
- J. Benevenia, S. M. Rivero, J. Moore, J. A. Ippolito, D. A. Siegerman, K. S. Beebe and F. R. Patterson, Clin. Orthop. Relat. Res., 2017, 475, 776–783 CrossRef PubMed.
- K. Y. Huang, J. J. Yan and R. M. Lin, Spine, 2005, 30, E585–E588 CrossRef PubMed.
- L. A. Thomson, F. C. Law, K. H. James, C. A. Matthew and N. Rushton, Biomaterials, 1992, 13, 811–818 CrossRef CAS PubMed.
- X. Shi, Y. Cui, Y. Pan, B. Wang and M. Lei, BMC Cancer, 2021, 21, 764 CrossRef CAS PubMed.
- K. Kita, Y. Takata, K. Higashino, K. Yamashita, F. Tezuka, T. Sakai, A. Nagamachi and K. Sairyo, Surg. J., 2017, 3, e1–e5 CrossRef PubMed.
- S.-L. Zhang, B. Xu, Y.-J. Lao and H.-F. Sheng, China J. Orthop. Traumatol., 2021, 34, 738–742 Search PubMed.
- Y. Cui, Y. Pan, Y. Lin, C. Mi, B. Wang and X. Shi, J. Orthop. Sci., 2022, 27, 79–83 CrossRef PubMed.
- L. Wang, C. Zhang, H. Liang, T. Huang, W. Zhong, Z. Zhao and X. Luo, World J. Surg. Oncol., 2022, 20, 112 CrossRef PubMed.
- H. Sun, Z. Yang, Y. Xu, X. Liu, Y. Zhang, Y. Chen, D. Xu, Y. Yang, D. Li and J. Xia, Eur. Spine J., 2015, 24, 1768–1777 CrossRef PubMed.
- A. O. Kirkegaard, S. T. Sorensen, D. S. Ziegler, L. Carreon, M. O. Andersen and R. Rousing, Danish Med. J., 2018, 65, A5509 Search PubMed.
- Q.-H. Tian, X.-Q. Sun, Y.-Y. Lu, T. Wang, C.-G. Wu, M.-H. Li and Y.-S. Cheng, J. Vasc. Int. Radiol., 2016, 27, 1420–1424 CrossRef PubMed.
- F.-A. Wang, S.-C. He, E.-H. Xiao, S.-X. Wang, L. Sun, P.-H. Lv and W.-N. Huang, Pain Physician, 2016, 19, E559–E567 Search PubMed.
- L. Wu, J. Fan, Q. Yuan, X. Zhang, M. Hu and K. Zhang, Int. J. Hyperthermia, 2021, 38, 1069–1076 CrossRef CAS PubMed.
- A. Baumann, J. Tauss, G. Baumann, M. Tomka, M. Hessinger and K. Tiesenhausen, Eur. J. Vasc. Endovasc. Surg., 2006, 31, 558–561 CrossRef CAS PubMed.
- S. Telera, N. Gorgoglione, L. Raus, A. Vidiri, V. Villani, A. Pace, A. Fabi, F. Crispo, M. Castiglione, I. Sperduti and R. Boccaletti, World Neurosurg., 2019, 127, E751–E760 CrossRef PubMed.
- I. Panagiotis, K. Panagiotis and V. Vasilios, Eur. Spine J., 2014, 23, S187–S191 CrossRef PubMed.
- S. F. Hassani, E. Cormier, E. Shotar, M. Drir, J. P. Spano, L. Morardet, J. P. Collet, J. Chiras and F. Clarencon, Eur. Radiol., 2019, 29, 663–673 CrossRef PubMed.
- F. Monticelli, H. J. Meyer and E. Tutsch-Bauer, Forensic Sci. Int., 2005, 149, 35–38 CrossRef CAS PubMed.
- Y. Zhang, X. Liu and H. Liu, Orthop. Surg., 2022, 14, 456–460 CrossRef PubMed.
- N. Aebli, J. Krebs, G. Davis, M. Walton, M. J. A. Williams and J. C. Theis, Spine, 2002, 27, 460–466 CrossRef PubMed.
- H. Ahmadzai, S. Campbell, C. Archis and W. A. Clark, Spine J., 2014, 14, E1–E5 CrossRef PubMed.
- H. Feng, J. Wang, P. Guo, J. Xu, W. Chen and Y. Zhang, Pain Physician, 2016, 19, E767–E773 Search PubMed.
- K. Li, J. Yan, Q. Yang, Z. Li and J. Li, J. Orthop. Surg. Res., 2015, 10, 20 CrossRef PubMed.
- S. Hoppe, T. Elfiky, M. J. B. Keel, E. Aghayev, T. M. Ecker and L. M. Benneker, Eur. Spine J., 2016, 25, 3463–3469 CrossRef PubMed.
- P. F. Heini and R. Orler, Orthopade, 2004, 33, 22–30, DOI:10.1007/s00132-003-0574-3.
- J. M. Mathis, Am. J. Neuroradiol., 2003, 24, 1697–1706 Search PubMed.
- F. H. Cornelis, F. Petitpierre, T. Fabre, O. Gille, N. Amoretti and O. Hauger, Eur. Radiol., 2017, 27, 3942–3946 CrossRef PubMed.
- G. C. Anselmetti, A. Manca, S. Tutton, G. Chiara, A. Kelekis, F. R. Facchini, F. Russo, D. Regge and F. Montemurro, Pain Physician, 2013, 16, E397–E404 Search PubMed.
- S. Tang, W. Fu, H. Zhang, H. Zhang and B. Liang, World Neurosurg., 2019, 132, E739–E745 CrossRef PubMed.
- W. Wang, H. Liu, Z. Wu, Y. Teng, Y. Huang, T. Liu and H. Yang, Geriatric Orthop. Surg. Rehabil., 2022, 13 DOI:10.1177/21514593221119625.
- R. Mohamed, C. Silbermann, A. Ahmari, M. Bohner, S. Becker and G. Baroud, Spine, 2010, 35, 353–360 CrossRef PubMed.
- M. Inoue, M. Sakane and T. Taguchi, J. Biomed. Mater. Res. Part B Appl. Biomater., 2014, 102, 1786–1791 CrossRef PubMed.
- T.-H. Leu, Y. Wei, Y.-S. Hwua, X.-J. Huang, J.-T. Huang and R.-J. Chung, Polymers, 2019, 11, 1971 CrossRef CAS PubMed.
- J. H. Healey, F. Shannon, P. Boland and G. R. DiResta, Clin. Orthop. Relat. Res., 2003, S263–S275, DOI:10.1097/01.blo.0000093053.96273.ee.
- G. Maccauro, A. Cittadini, M. Casarci, F. Muratori, D. De Angelis, C. Piconi, M. A. Rosa, A. Spadoni, M. Braden and A. Sgambato, J. Mater. Sci. Mater. Med., 2007, 18, 839–844 CrossRef CAS PubMed.
- R. Llombart-Blanco, C. Villas, A. Silva, A. Aldaz, I. Navarro, J. Forteza, S. Martin Algarra and M. Alfonso, Eur. Spine J., 2017, 26, 3216–3224 CrossRef PubMed.
- L. Bao, X. Li, Y. Qi, Z. Wang and J. Li, Chem. Eng. Sci., 2020, 215, 115379 CrossRef CAS.
- M. Shi, J. D. Kretlow, A. Nguyen, S. Young, L. S. Baggett, M. E. Wong, F. K. Kasper and A. G. Mikos, Biomaterials, 2010, 31, 4146–4156 CrossRef CAS PubMed.
- B. M. Liu, M. Li, B. S. Yin, J. Y. Zou, W. G. Zhang and S. Y. Wang, PLoS One, 2015, 10, e0144407 CrossRef PubMed.
- X. Zheng, Y. Wang, J. Liu, J. Han, Z. Cui, S. Wu, Y. Liang, S. Zhu, X. Ge and Z. Li, Mater. Res. Express, 2022, 9, 035405 CrossRef.
- J. A. Handal, N. C. Tiedeken, G. E. Gershkovich, J. A. Kushner, B. Dratch and S. P. Samuel, J. Surg. Res., 2015, 194, 161–166 CrossRef CAS PubMed.
- E. L. Cyphert, N. Kanagasegar, N. Zhang, G. D. Learn and H. A. von Recum, Macromol. Biosci., 2022, 22, e2100415 CrossRef PubMed.
- Z. Wang, L. P. Nogueira, H. J. Haugen, I. C. M. Van der Geest, P. C. D. A. Rodrigues, D. Janssen, T. Bitter, J. J. J. P. van den Beucken and S. C. G. Leeuwenburgh, Bioact. Mater., 2022, 15, 120–130 CrossRef CAS PubMed.
- Z. Yang, D. Yang, L. Xie, Y. Sun, Y. Huang, H. Sun, P. Liu and Z. Wu, Acta Radiol., 2009, 50, 1142–1148 CrossRef PubMed.
- S. Wang, G. Shi and X. Meng, Pak. J. Pharm. Sci., 2015, 28, 1039–1042 Search PubMed.
- J. Lu, J. Deng, H. Zhao, M. Shi, J. Wang and L. Zhao, Eur. J. Radiol., 2011, 78, 296–301 CrossRef PubMed.
- M. Kawashita, K. Kawamura and Z. Li, Acta Biomater., 2010, 6, 3187–3192 CrossRef CAS PubMed.
- Y. Ling, X. Tang, F. Wang, X. Zhou, R. Wang, L. Deng, T. Shang, B. Liang, P. Li, H. Ran, Z. Wang, B. Hu, C. Li, G. Zuo and Y. Zheng, RSC Adv., 2017, 7, 2913–2918 RSC.
- M. Harabech, N. R. Kiselovs, W. Maenhoudt, G. Crevecoeur, D. Van Roost and L. Dupre, AIP Adv., 2017, 7, 056704 CrossRef.
- M. Miola, A. Bellare, F. Laviano, R. Gerbaldo and E. Verne, Ceram. Int., 2019, 45, 14533–14545 CrossRef CAS.
- B. Ren, Z. Han, W. Li and J. Liu, Life-Basel, 2022, 12, 1342 CrossRef CAS PubMed.
- M. Kubota, T. Yokoi, T. Ogawa, S. Saito, M. Furuya, K. Yokota, H. Kanetaka, B. Jeyadevan and M. Kawashita, Ceram. Int., 2021, 47, 12292–12299 CrossRef CAS.
- K. Yu, B. Liang, Y. Zheng, A. Exner, M. Kolios, T. Xu, D. Guo, X. Cai, Z. Wang, H. Ran, L. Chu and Z. Deng, Theranostics, 2019, 9, 4192–4207 CrossRef CAS PubMed.
- K. M. Zekry, N. Yamamoto, K. Hayashi, A. Takeuchi, A. Z. A. A. Alkhooly, A. S. Abd-Elfattah, A. N. S. Elsaid, A. R. Ahmed and H. Tsuchiya, J. Orthop. Surg., 2019, 27 DOI:10.1177/2309499019832970.
- B. A. Hain and D. L. Waning, Curr. Osteoporosis Rep., 2022, 20, 433–441 CrossRef PubMed.
- V. Schirrmacher, Int. J. Oncol., 2019, 54, 407–419 CrossRef CAS PubMed.
- S. Prabakaran, M. Jeyaraj, A. Nagaraj, K. K. Sadasivuni and M. Rajan, Appl. Nanosci., 2019, 9, 1487–1500 CrossRef CAS.
- K. E. Marks, C. L. Nelson and E. P. Lautenschlager, J. Bone Joint Surgery. Am. Vol., 1976, 58, 358–364 CrossRef CAS.
- C. D. Pargas, A. H. Elhessy, M. Abouei, M. G. Gesheff and J. D. Conway, Antibiotics, 2022, 11, 336 CrossRef CAS PubMed.
- V. Wall, T.-H. Nguyen, N. Nguyen and P. A. Tran, Biomedicines, 2021, 9, 26 CrossRef CAS PubMed.
- F. G. Draenert and K. Draenert, Chemotherapy, 2008, 54, 412–416 CrossRef CAS PubMed.
- S. Decker, W. Winkelmann, B. Nies and F. van Valen, J. Bone Jt. Surg. Br. Vol., 1999, 81, 545–551 CrossRef CAS.
- E. Prochazka, T. Soukup, M. Hroch, L. Fuksa, E. Brcakova, J. Cermanova, G. Kolouchova, K. Urban, J. Mokry and S. Micuda, Int. Orthop., 2010, 34, 137–142 CrossRef PubMed.
- M. A. Rosa, G. Maccauro, A. Sgambato, R. Ardito, G. Falcone, V. De Santis and F. Muratori, J. Bone Jt. Surg. Br. B Vol., 2003, 85, 712–716 CrossRef CAS.
-
Y. Sa, F. Yang, Y. N. Wang, J. G. C. Wolke and J. A. Jansen, in Cutting-Edge Enabling Technologies for Regenerative Medicine, ed. H. J. Chun, C. H. Park, I. K. Kwon and G. Khang, 2018, vol. 1078, pp. 119–134 Search PubMed.
- H. Wang, W. Zhi, X. Lu, X. Li, K. Duan, R. Duan, Y. Mu and J. Weng, Acta Biomater., 2013, 9, 8413–8421 CrossRef CAS PubMed.
- S. V. Dorozhkin, Biomaterials, 2010, 31, 1465–1485 CrossRef CAS PubMed.
- M. Shi, J. D. Kretlow, P. P. Spicer, Y. Tabata, N. Demian, M. E. Wong, F. K. Kasper and A. G. Mikos, J. Controlled Release, 2011, 152, 196–205 CrossRef CAS PubMed.
- C. Chen, Z. Gao, X. Qiu and S. Hu, Molecules, 2013, 18, 7239–7252 CrossRef CAS PubMed.
- M. Gumińska, J. Ignacak and E. Wójcik, Pol. J. Pharmacol., 1996, 48, 495–501 Search PubMed.
- B.-M. Liu, M. Li, B.-S. Yin, J.-Y. Zou, W.-G. Zhang and S.-Y. Wang, PLoS One, 2015, 10, e0144407 CrossRef PubMed.
- E. L. Cyphert, N. Kanagasegar, N. Zhang, G. D. Learn and H. A. von Recum, Macromol. Biosci., 2022, 22, e2100415 CrossRef PubMed.
- S. Faruqi, C.-L. Tseng, C. Whyne, M. Alghamdi, J. Wilson, S. Myrehaug, H. Soliman, Y. Lee, P. Maralani, V. Yang, C. Fisher and A. Sahgal, Neurosurgery, 2018, 83, 314–322 CrossRef PubMed.
- A. E. Hirsch, B. S. Rosenstein, D. C. Medich, C. B. Martel and J. A. Hirsch, Pain Physician, 2009, 12, 887–891 Search PubMed.
- S. S. Danewalia and K. Singh, Mater. Today Bio, 2021, 10, 100100 CrossRef CAS PubMed.
- A. M. Derfus, G. von Maltzahn, T. J. Harris, T. Duza, K. S. Vecchio, E. Ruoslahti and S. N. Bhatia, Adv. Mater., 2007, 19, 3932–3936 CrossRef CAS.
- E. A. Perigo, G. Hemery, O. Sandre, D. Ortega, E. Garaio, F. Plazaola and F. J. Teran, Appl. Phys. Rev, 2015, 2, 041302 Search PubMed.
- P. B. Santhosh and N. P. Ulrih, Cancer Lett., 2013, 336, 8–17 CrossRef CAS PubMed.
- M. Bruno, M. Miola, O. Bretcanu, C. Vitale-Brovarone, R. Gerbaldo, F. Laviano and E. Verne, J. Biomater. Appl., 2014, 29, 254–267 CrossRef CAS PubMed.
- H. Huang, S. Delikanli, H. Zeng, D. M. Ferkey and A. Pralle, Nat. Nanotechnol., 2010, 5, 602–606 CrossRef CAS PubMed.
- B. Thiesen and A. Jordan, Int. J. Hyperthermia, 2008, 24, 467–474 CrossRef CAS PubMed.
-
T. J. Gutiérrez and V. A. Alvarez, in Handbook of Nanomaterials for Industrial Applications, ed. C. Mustansar Hussain, Elsevier, 2018, pp. 563–576 DOI:10.1016/B978-0-12-813351-4.00032-8.
- Z. Li, M. Kawashita, N. Araki, M. Mitsumori, M. Hiraoka and M. Doi, Mater. Sci. Eng., C, 2010, 30, 990–996 CrossRef CAS.
- M. Ma, Y. Wu, J. Zhou, Y. Sun, Y. Zhang and N. Gu, J. Magn. Magn. Mater., 2004, 268, 33–39 CrossRef CAS.
- Y. Xia, H. Wang, Y. Li and C. Fu, Front. Mater., 2022, 9, 929618 CrossRef.
- C. Wang, X. Wang, X.-L. Xu, X.-L. Yuan, W.-L. Gou, A.-Y. Wang, Q.-Y. Guo, J. Peng and S.-B. Lu, PLoS One, 2014, 9, 0096361 CrossRef PubMed.
- Y. Tamaki, K. Sasaki, A. Sasaki, Y. Takakubo, H. Hasegawa, T. Ogino, Y. T. Konttinen, J. Salo and M. Takagi, J. Biomed. Mater. Res. Part B Appl. Biomater., 2008, 84, 191–204 CrossRef PubMed.
- O. Demir-Oguz, A. R. Boccaccini and D. Loca, Bioact. Mater., 2023, 19, 217–236 CrossRef CAS PubMed.
- W. Liu, Z. Huan, C. Wu, Z. Zhou and J. Chang, Composites, Part B, 2022, 247, 110324 CrossRef CAS.
- Y. Liu, Z. Y. Jiang, S. Z. Tong, Y. F. Sun, Y. Zhang, J. Y. Zhang, D. Y. Zhao, Y. Z. Su, J. X. Ding and X. S. Chen, Adv. Mater., 2023, 35, 2203291 CrossRef CAS PubMed.
- Z. Y. Jiang, Y. Liu, R. Shi, X. R. Feng, W. G. Xu, X. L. Zhuang, J. X. Ding and X. S. Chen, Adv. Mater., 2022, 34, 2110094 CrossRef CAS PubMed.
- D. Li, S. Liu, Y. Ma, S. Liu, Y. Liu and J. Ding, Small Methods, 2023, 7, e2300204 CrossRef PubMed.
- J. Zhang, D. Tong, H. Song, R. Ruan, Y. Sun, Y. Lin, J. Wang, L. Hou, J. Dai, J. Ding and H. Yang, Adv. Mater., 2022, 34, e2202044 CrossRef PubMed.
|
This journal is © The Royal Society of Chemistry 2023 |
Click here to see how this site uses Cookies. View our privacy policy here.