DOI:
10.1039/D2TB01564A
(Review Article)
J. Mater. Chem. B, 2023,
11, 10-32
New aspects of lipopeptide-incorporated nanoparticle synthesis and recent advancements in biomedical and environmental sciences: a review
Received
23rd July 2022
, Accepted 2nd November 2022
First published on 7th November 2022
Abstract
The toxicity of metal nanoparticles has introduced promising research in the current scenario since an enormous number of people have been potentially facing this problem in the world. The extensive attention on green nanoparticle synthesis has been focussed on as a vital step in bio-nanotechnology to improve biocompatibility, biodegradability, eco-friendliness, and huge potential utilization in various environmental and clinical assessments. Inherent influence on the study of green nanoparticles plays a key role to synthesize the controlled and surface-influenced molecule by altering the physical, chemical, and biological assets with the provision of various precursors, templating/co-templating agents, and supporting solvents. However, in this article, the dominant characteristics of several kinds of lipopeptide biosurfactants are discussed to execute a critical study of factors affecting synthesis procedure and applications. The recent approaches of metal, metal oxide, and composite nanomaterial synthesis have been deliberated as well as the elucidation of the reaction mechanism. Furthermore, this approach shows remarkable boosts in the production of nanoparticles with the very less employed harsh and hazardous processes as compared to chemical or physical method-based nanoparticle synthesis. This study also shows that the advances in strain selection for green nanoparticle production could be a worthwhile and strong economical approach in futuristic medical science research.
1. Introduction
Nanoparticles (microporous) refer to an enormous class of materials with structural dimensions in the particular range of 1–100 nm in diameter.1–6 Generally, nanoparticles require high temperatures, pressure, and externally applied energy in synthesis procedures, which are the main causes of hazards to the environment.7 Recently, due to the unprecedented growth of industries, environmental pollutants have touched peak levels.8 Among these pollutants, the contamination in soil and water are major sources to disrupt the environmental balance. In the case of soil, the growth of soil microorganisms is concerned due to account contamination of sludge and plant tissue, which is also impaired due to the insignificant growth of grass seedlings by various nanoparticles, such as Ag, and ZnO.9,10 The negative impact of nanoparticles also poses an adverse impact on the aquatic ecosystem at very low concentrations, where the high concentrations of nanoparticles are enormously toxic for most mammals.11 However, water pollution plays a pivotal role to cause the environmental functioning via various chemical template-based nanoparticles, such as Ag, Au, mesoporous materials, ZnO, Fe3O4, minerals, graphene, fullerenes, TiO2, and MnO2, where the availability of enormous toxic organic dyes (e.g. safranine O, methylene blue, crystal violet, acid orange 7, acid red 265, rhodamine B, malachite green, etc.) are majorly responsible to reduce the purity of water.12 Among these, organic dye pollutants originate from the wastewater and travel into natural brooks and rivers through paper, food, textile, plastic, cosmetics, leather, paints, furniture industries, etc.13–15 and are treated by different processes such as hydrolysis, separation, biodegradation, ion-exchange, membrane filtration, oxidation, adsorption, by-product formation, coagulation, and electrolysis method.16
In general, nanoparticles are predominantly synthesized by two important approaches: top-down and bottom-up methods. Whereas the bottom-up method is found to be enormously advantageous over the top-down due to higher production of particles with fewer solid defects, well-ordered and substantial homogeneity.17 The most conventional techniques such as the sol–gel method and co-precipitation methods are mainly used to produce the nanoparticles, where the major negative impacts of these methods involve uncontrollable morphology of the particles at a large scale of production. Among all techniques, microemulsion methods have shown more promising and crucial ways for the synthesis of nanoparticles.18 Thus, the described chemical synthesis processes of nanoparticles may involve toxic chemicals and are less convenient.19 At the same time, these synthesis processes generally require high pressure, temperature, and energy, and possess the ultimate threat to the environment.20
Surfactants are predominantly used in detergents, wetting agents, emulsifiers, foaming agents, and dispersants, as well as in the food, cosmetics, and petroleum industries.21,22 There are two types of surfactants projected as biosurfactant and chemical-surfactant, where the biosurfactant (e.g. plant, bacteria, fungi, algae, etc.) are employed economically and environmentally friendly compared to the chemical surfactants.17,23,24 Specifically, the biosurfactants have been segmented into four major categories as glycolipid, lipopeptide, polymeric and particulate biosurfactants.25 Among the above-mentioned categories, the lipopeptide-produced biosurfactants have exhibited a promising outcome on account of their multifunctional nature, which have become a part of diverse biotechnological applications including biocontrol, drug delivery vehicles, enhanced oil recovery, and bioremediation.26 Therefore, the biosurfactants enlightened a green stabilizer in nanobiotechnology and are also expected to integrate the surface properties of multifunctional materials in the forthcoming generation.27
The synthesis of nanoparticles can be performed using various microorganisms such as bacteria, fungi, viruses, algae, and part of the organism (e.g., plant extracts) to reduce the toxicity of materials and balance environmental sustainability.28,29 Microorganism-based synthesized nanoparticles have been employed rapidly, with friendly cooperation with nature and more precise materials at the industrial levels. Green chemistry has instigated an alternative route for the synthesis of nanoparticles employing biosurfactants. To sustain the biodiversity and harmony of plants/living organisms in the environment, green synthesis has been recommended by most researchers in nanoparticle synthesis. Recently, researchers have principally focussed on the green development of materials due to the less toxic and more eco-friendly nature of green nanotechnology. Biosynthesized nanoparticles have been actively used in different applications potentially such as cancer treatment, gene therapy, biosensors, DNA analysis, MRI, and antibacterial agents. A most unique production of biosurfactant was implemented through mangrove isolation from soil remediation and, their effects were further studied by pre-treatment and direct application methods.30 Recently, the significant development of metallic, sulfide, oxide nanoparticles, and others have been highlighted in biosynthesis (green synthesis) production and used at a very large scale in bio-nanotechnological industries.27
Currently, lipopeptide-mediated nanomaterial synthesis has been used as an alternative material for implementation in research as antibiotic agents.31,32 The effects of green nanoparticles have shown influence in bactericidal against Gram-positive/negative bacteria and, fungal pathogens and gene therapy applications,33 however, most of the researchers have recommended the use of Gram-positive bacteria to produce the lipopeptides biosurfactants.34 Additionally, low toxicity and more biodegradability of lipopeptide biosurfactant-mediated nanomaterial synthesis have established eco-friendly materials with core effectiveness in the research and development sector.35
On the basis of previously-published studies, some knowledge gap still exists in the green synthesis of nanoparticles such as suitable bacterial strain selection, proper synthesis methodology, reducing the production cost, and their development in nanotechnologies to control environmental pollution, and toxicity. Besides, microbial surfactant-based nanomaterial synthesis has still not developed processes for producing materials with very small pore sizes and perfect shapes as with chemical surfactant-based materials. Thus, this review addresses the present and future prospective ideas to develop the synthesis procedure for nanomaterials using biosurfactants. Ongoing prime research in this field has mainly focused to control the shape and size of nanomaterials through biological templates and, improving the eco-friendliness of materials with less toxicity in the future. Recently, a new approach of surfactant was discovered to prevent respiratory distress syndrome in newborn babies, which has been reported as a very dangerous disease in the Neonatal Period.36
2. Historical perspective and recent advancements in lipopeptide biosurfactant in nanotechnology
The lipopeptide word exemplifies the incorporation of lipids with a peptide bond. The lipopeptide was isolated for the first time in the 1950 and 1960s and was linked between peptide and fatty acid chains,37 and Bacillus was subjected as the most prominent microorganism for lipopeptide biosurfactant production. The origin of the Bacillus word was specified as ‘bacilli’ by Christian Gottfried Ehrenberg in 1835, where the survival of bacteria (Bacillus) in an environment was recognized as Gram-positive in both the aerobic and anaerobic conditions and rod or, cylindrical shapes.38,39 A wide range of different bacterial strains was also reported in the soil and water samples of environment,40,41 and can easily be transformed into Gram-negative strains after the ageing of a few particular bacterial strains.42 Moreover, the influence of heat on these bacteria played a very important role and was the first time described by German botanist Ferdinand Cohn in 1877. Some bacteria exhibited heat resistance (endospores) and the highest growth rate naturally, whereas others required an external source of heat for their optimum growth. Pettersson et al.43 showed the highest heat resistance Bacillus species (i.e. Bacillus sporothermodurans M215). Furthermore, Berendsen et al.44 described two of the most highest heat-resistant Bacillus genus, i.e., Bacillus amyloliquefaciens and Bacillus licheniformis in food chains, nevertheless, the counterparts (negative) of these bacteria was stated as less heat-resistant. The other strains of Bacillus were also introduced, such as B. subtilis IF0 3039, IAM 1069, IAM 1213, IAM 1259, and IAM 1260, and have performed as efficient producers of biosurfactants in the lipopeptide group.45 Additionally, other types of microorganisms also produce the lipopeptide biosurfactants such as Pseudomonas fluorescens, Serratia marcescens, Arthrobacter sp., N. alba strain MSA10, and Inquilinus limosus KB3.26,46,47 Prominently, the most surface-active lipopeptide biosurfactant was proposed for the first time in 1968, naturally, comprising cyclic forms of peptide-linked fatty acids (tail portion). Interestingly, lipid chains of the fatty acids endure accountable to assemble with inorganic components (materials) and develop effective eco-friendly biomaterials. In the global market, the distribution of biosurfactants is still limited due to higher production and purification costs. Recently, the inherent engineering tools and sustainable acids are being used to reduce production costs and increase the percentage (%) yield through statistical methods.48 However, biosurfactants production in the international market has been growing continuously day by day, which is predicted to reach ∼USD 5.52 billion by the end of the year 2022, and the percentage (%) of CAGR (compound annual growth rate) was expected to grow up 5.6% from 2017 to 2022.17 Recently, the highest production of biosurfactants was mainly reported in the European region [Germany (Biotensidon, Evonik), France, Italy, Belgium (Ecover), UK, etc.], including India, Brazil, USA (Jeneil Biotech), Thailand, China, Japan, etc.17 Recently, some research groups have used biosurfactants as supporting agents to integrate the materials for quick and easy production of nanoparticles such as Ag, Au, and Fe3O4.
3. Categories of nanoparticle synthesis using lipopeptide biosurfactants
The lipopeptide biosurfactants are mainly categorized into six classes, i.e., surfactin, daptomycin, iturins, echinocandins, fengycin (or plipastatin), and kurstakins, as described further. However, different processes have been employed to synthesize nanoparticles, i.e., physical, chemical, and biological methods, as shown in Table 1. Remarkably, the biological method of synthesis is a rapid, efficient, and reasonable method compared to the other two processes. However, chemical surfactants have introduced some negative impacts on the environment, concerns such as the surfactants could penetrate the drinking water and have health issues for humans, animals, and aquatic life.49 In recent publications, the cause of surfactants in environmental and public threats to humans and ecosystems was reported, where the major issues observed in anionic surfactants, linear alkylbenzene sulfonates (LAS), cause pathological, biochemical, physiological, and has further impacts on terrestrial or, aquatic ecosystems.34,50,51 As an example, the LAS predominantly causes skin irritation, and respiratory syndrome problems diminish the aquatic biota's resistance to environmental stress, growth procedures, and reproduction. On the other hand, the integration of hydrophobicity and solubility through surfactants facilitates their toxicity and eutrophication.52 To overcome the cause of public health and environmental concern, biosurfactants have played a significant role in reducing toxicity, integrating biodegradability, effectiveness at higher temperatures or, pH values, digestibility, economical and biocompatibility. The higher production cost has been observed as a significant disadvantage in biosurfactants. However, recently, there have been some studies with lower-cost materials on pilot plants or, industrial scale.53
Table 1 Significant advantages and disadvantages of physical, chemical, and biological processes in nanomaterials synthesis
Methods |
Synthesis |
Advantages |
Disadvantages |
Physical process |
Top-down approach |
Crystallinity controllable |
High capital cost |
Uniform particle shape |
Enormous energy consumption |
No chemical purification required |
Less economical |
A high percentage of purity |
Material abrasion |
Chemical process |
Bottom-up approach |
Large-scale production |
Less eco-friendly |
Size controllable |
Energy intensive process |
Reproducible |
Hazardous chemicals as reducing agent |
Biological process |
Bottom-up approach |
Less expensive source |
Expensive process |
Negligible toxicity |
Aseptic cultivation required |
Easily available |
|
Eco-friendly |
|
3.1. Surfactin
Surfactin (molecular weight: 1036 Da) is the most innovative and efficient biosurfactant in the current research arena of nanobiotechnology due to lower surface and interfacial tension (Fig. 1). The surfactin comprises both the hydrophilic and hydrophobic properties with structural elucidation of the cyclic form (protein: 13%, lipid: 17%), which is interlaced to peptide bonds and fatty acid.54,55 Due to the efficient production of biosurfactants in industries, it is greatly used as a template and stabilizing agent in the green synthesis of nanoparticles via easy, eco-friendly, less expensive, and less time-consuming processes and is deliberated as a good catalyst-like material. Additionally, surfactin was subjected as the most dominant biosurfactant to reduce the surface tension at a very low concentration (∼0.05%).56 Recently, the highest surfactin (Bacillus amyloliquefaciens) production was introduced as 4.93–7.15 g L−1 in the flask and 5.94–9.18 g L−1 in a bioreactor by Yang et al.57 The surfactin was not only able to increase the potentiality of nanoparticles but also the nanoparticles could increase the amount of surfactin production, such as Fe-nanoparticles. However, in the conclusion of their study, they found an approximately 50% higher production rate for surfactin in the presence of Fe-nanoparticles. Additionally, the authors have also demonstrated the improvement of cell membrane permeability and secretion, which would be able to boost the metabolites of microbes for integration in green nanoparticle synthesis.57 Furthermore, Geissler et al.58 deliberated the higher production and efficacy of surfactin compared to other biosurfactant-mediated nanomaterials such as iturins, mycosubtilin, fengycin, plipastatin, kurstakins, locillomycin, daptomycin, echinocandins, lichenysin, viscosin, amphisin, polymyxin, pseudofactin-II, where the proficiency of surfactin and their applications were significantly better compared to others, such as de-emulsification, oil recovery, and foaming capacity.59–61 Reddy et al.62 reported an eco-friendly synthesis of silver nanoparticles through Bacillus subtilis produced biosurfactant (surfactin). In this study, silver nitrate (precursor) and sodium borohydride was used as reducing agent at very low temperatures (4 °C), where the physicochemical properties of the produced nanoparticles were predominantly influenced by their morphology and size due to altering of the pH and temperature in the synthesis procedure. Subsequently, in the same year, Reddy et al.63 used the same type of biosurfactant to synthesize gold nanoparticles using HAuCl4 as a precursor and reducing agent NaBH4, which was observed as a new highly stable green nanoparticle. The gold nanoparticles of 4.70 nm in pore diameter were observed with a more uniform and unique spherical shape/size at standard pH 7. The progression of nanoparticles was accomplished under neutral conditions at room temperature. The authors concluded that the lipopeptide biosurfactant was predominantly effective whilst the synthesis of green nanoparticles in the absence of chemical activating agents and in the presence of biological activating agents. A few years later, Singh et al.64 introduced a biocompatible and inexpensive stabilizing agent-based synthesized green CdS nanomaterial using surfactin (Bacillus amyloliquifaciens KSU-109). The particles were observed to be very stable at standard neutral pH 7.2 with cubic/hexagonal shapes in the pore size range of 3–4 nm at ambient temperature. The authors strongly suggested applying nanotechnological applications due to non-hazardous and ecologically compatible nanoparticles. In the meantime, Maity et al.23 produced a very stable nanocrystalline brushite composite material using the reverse microemulsion method at room temperature. It was observed that the calcined brushite particles comprised nanosphere, oval, and nanorods in shape. However, the green material was observed with different particle diameter ranges 50–200 nm, ∼300 nm, and 200–400 nm. Regardless of the green nanoparticle synthesis, the higher surface energy, pores size, dispersibility, conservation of colloidal stability, and aggregation/agglomeration problem have signposted a great negative impact on the proposed nanoparticles. Gradually, Maity et al.65 introduced another interesting publication, where a novel adsorbent hydroxyapatite nanoparticle was synthesized using surfactin as a templating agent by the hydrothermal method. Moreover, the control of pore size and morphology of nanoparticles in this work shows a significant benefit to improve the surface properties. In this case, the produced rod-shaped nanoparticles gave the best results at 90 °C (pH ∼ 11) with the a pore size of 20–30 nm. Another important character was also detected whilst varying the temperature from 90–150 °C, where the particle size was increased from 78–124 nm and the shape of the particle transformed from short to long rod shape. To control or, significantly reduce the nanoparticle pore size, Bezza et al.66 projected a colloidally-stable and ultra-small size of silver nanoparticles using a very expedient synthesis method (reverse micelle microemulsion method/chemical reduction method). The produced monodisperse spherical shaped nanoparticles have remarkable and enduring stability with a smaller particle diameter range of 20–22 nm, as compared to the chemically synthesized polydisperse silver nanoparticles. Moreover, this unique nanoparticle was applied in biomedical science to explore the bactericidal properties. In a recent year study, Sharma et al.17 described the eco-friendly and less toxic BMSN (biologically synthesized mesoporous silica nanoparticles) material using the sol–gel method at room temperature; they observed regular arrangement of particles with less agglomeration/aggregation of perfectly spherical shaped at 550 °C calcination temperature. Furthermore, the authors suggested using the materials for various applications, such as catalysis, bio/gas-separation, drug delivery systems, diagnostics, and cosmetics.
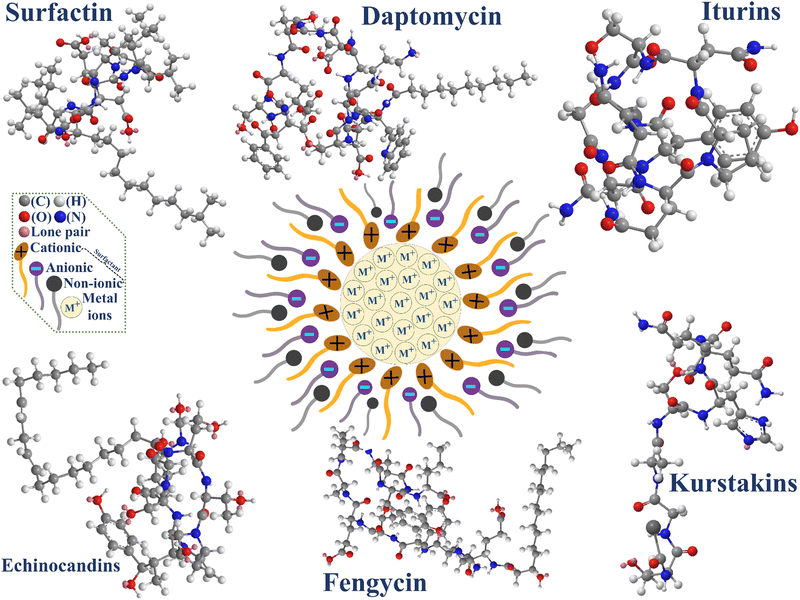 |
| Fig. 1 Lipopeptide impregnated nanoparticles molecular diagram. | |
3.2. Daptomycin
Daptomycin (1619.709 Da) biosurfactant was discovered for the first time in the 1980s by a research group at the Eli Lilly company (Fig. 1); it was reported as an alternative biosurfactant of vancomycin to treat the harmful bacterial infected part of the body easily and quickly as compared to vancomycin. In most cases, when vancomycin fails to kill the Gram-positive bacteria of the living organism, the daptomycin biosurfactant shows an entire activity to kill the Gram-positive bacteria easily.67 It has a unique structure as a cyclic lipopeptide, produced using the core part of 13-amino acids and the tail part of the decanoyl side chain.68 In the case of green nanoparticle synthesis, the produced organic templates using bacteria, fungi, plants, etc. from natural resources were implemented to control the nucleation and crystalline growth of nanoparticles. Most of the natural biomolecules are easily assembled in chemical precursors and produce low toxic green nanoparticles,69 which were exhibited as more suitable to control the morphology.70,71 The research on nanoparticle synthesis with the daptomycin biosurfactant was rarely employed due to the loss of critical concerns and eventual course compared to others. The development of the most dominant green silver nanocluster was reported by Zheng et al.72 using daptomycin (antibiotics) as a supportive mediator. In that work, daptomycin played a very important role to reduce the particle size with the combination of silver precursor and increase the efficacy of antimicrobial activity and was significantly employed to enhance the killing effect of the bacterial cell. However, the incessant localization of daptomycin in silver nanoclusters generated a high level of ROS concentration in bacteria, which allowed them to damage the DNA and increase the antibacterial efficiency. Another research group, Chakravarty et al.,73 explored bi-metallic green nanoparticle synthesis using daptomycin. In this case, the author executed two different precursors to form the Ag–Au bimetallic green nanoparticles with a particle size range of 25–40 nm, where the crystalline nature of the material was observed with the face-centered cubic symmetry. Thus, suitable protocols for low toxic and green bi-metallic materials are still not developed, which can entirely control the enormous physicochemical properties of nanomaterials and lessen the cost. Recently, gold nanoflower composite materials were used to enhance the stability and activity of daptomycin, which showed antitumor and antibacterial activity in living organisms.74 Remarkably, daptomycin has also been used to ensure the specificity of targets by loading daptomycin in coated polydopamine on gold nanocages (therapeutic agent), which improved multidrug resistance treatment in medical technology.75 Furthermore, daptomycin has developed a new potential to resist cancer and immunomodulatory bacterial activities.76 In one of the very recent publications, the authors have addressed a successfully fabricated nanomaterial graphene oxide membrane-daptomycin/epidermal growth factor to treat the wound healing disease. However, it could be capable of integrating the stability of graphene oxide membranes and their therapeutic effects against Gram-negative bacteria.77
3.3. Iturins
The structure of Iturins was revealed for the first time by Peypoux et al.78 (Fig. 1). Primarily, the Iturins biosurfactant was used as antibiotic and antifungal activities.79 The development of biosurfactant-mediated nanoparticles has mainly been categorized into six parts, iturin A (1043.2 Da), iturin C (1044.2 Da), bacillomycin D (1030 Da), bacillomycin F (1101.3 Da), bacillomycin L (1034.54 Da), and mycosubtilin (1199.4 Da). The heptapeptide cyclic structure with alkyl groups of iturins was proposed in 1978, which was generally combined with β-amino fatty acids.78,80 Some of the vital mitigations in the production of Iturins have been reported using BW (brewery wastewaters), APE (apple peel extract), and CPE (carrot peel extract) media, which was prepared from the extraction of vegetables.81 The iturins have been predominantly applicable in the cosmetics industry (detergents), anti-wrinkle agents (anti-ageing products), and whitening agents (cosmetics), which were further used to preserve the biological products in the food industry.82,83 Zhao et al.84 developed a highly stable and very unique pore size (20 nm; spherical) in green silver nanoparticles, introduced with the help of iturins biosurfactant as a mediator, where it was instituted as more selective and sensitive nanoparticles for various reaction systems. Most importantly, the green nanomaterial was synthesized using the UV-irradiation method, which was a less time-consuming and easy process in the presence of green templates. Silver nanoparticle synthesis has been observed to have a good potential to improve the efficiency in food preservation and antifungal and antibacterial effects in biomedical science. However, the application of silver nanoparticles has been noted as still limited due to very less support in food and medical science application at high concentrations. The lower concentration of nanoparticles could be a reasonable way to use in these applications so that the food or, medical products could be less/not toxic to human health. In another study, Zhao et al.85 emphasized an attractive and more significant silver nanoparticle synthesis using biosurfactant iturin A and, irradiated using UV radiation for 30 minutes to complete the chemical reaction and formation of green nanoparticles (pore size: 10–30 nm). It was observed that the proposed results have enlightened significant advantages for biomedical applications and control of human health problems, and also used to prevent food from bacterial and fungal infection. In the same year, a (biosurfactant) iturin-mediated silver nanoparticles were produced to investigate the antifungal activity and a nanocomposite material was further prepared by incorporating iturin-mediated silver nanoparticles with chitosan to improve the efficacy in the treatment of wound healing of mice.86 Progressively, Zhou et al.87 in the same year, reported the preparation of chitosan-incorporated iturin–silver nanoparticles again to use and verify their stable application in wound healing care. The author profoundly observed that the eco-friendly prepared materials exhibited a great potential to inhibit bacterial infections and provided an effective treatment of wound healing at negligible toxicity to entire organs in mice. Another important study of silver nanoparticles was reported by Yuan et al.,88 where the surface area and encapsulation effects were upgraded with the help of iturin A. In this study, the preparation method was implemented similarly to that in the previous report (microwave irradiation method) and a novel nanocomposite sub-micron chitosan-modified material was produced using the templating agent iturin A, stepped up the stability of materials at flexible pH and the optimal time to integrate the antifungal activity by controlling the iturin A release. However, further research is still needed to improve their productivity for future applications. On the other hand, the improvement of iturin lipopeptides production was reported by Ravi et al.89 and the ZnO nanoparticle were driven as supplementation to improve the substantial production of iturin.
3.3.1. Mycosubtilin.
Mycosubtilin biosurfactant embodied-family member of iturins biosurfactant (1949) was prepared for the first time in a broth medium using Bacillus sp. at the western regional laboratory, USA.90 It was arranged in LDDLLDL sequence of α-amino acids to form the organic chemical structure,91 where it was involved in four different open reading frames, i.e., fenF, mycA, mycB and mycC, were controlled using the same promoter (Pmyc).92 The synthesis of nanoparticles with mycosubtilin biosurfactant has still not been reported in the nanotechnological field. However, remarkably, the presence of ergosterol in mycosubtilin microorganisms was used to affect the specific target over the sterol group.93
3.4. Echinocandins
The echinocandins biosurfactant is an antifungal cyclic hexapeptide molecule, isolated in 1974, used against fungal infection by Sandoz94 (Fig. 1). Furthermore, the three derivatives of echinocandins were reported as anidulafungin, caspofungin, and micafungin to develop the fungicidal activities against the pathogenic fungi in humans.95 The direct synthesis of nanoparticles with echinocandins has not been reported, which could be due to its less binding capacity with inorganic precursors/less efficient/very high production cost, or low availability of echinocandins. Nevertheless, the synthesized nanoparticles with the help of echinocandins could be effective in the advancement of medical nanotechnologies, such as for the combination of echinocandins and AgNPs introduced to reduce cytotoxicity of mammalian cells.96 The complexed nanoparticle was further suggested for use as an antifungal for clinical treatment or, new nano-drug development in drug delivery and antimicrobial activity.97 In the last few decades, there have been reports on the semisynthetic properties that execute great success in the development of clinical applications.95 Moreover, the echinocandins biosurfactants have also been used to prevent from candida biofilm, it is possible due to advancement of medical equipment.98
3.5. Fengycin and plipastatin
The fengycin biosurfactant was discovered by a research group of German scientists, whereas the plipastatin was revealed by a research group of Japanese scientists in the same year (1986)99 (Fig. 1). Precisely, in elemental analysis, fengycin possesses only glutamic acid (no glutamine) in lipopeptide biosurfactant,100 wherein both biosurfactants (fengycin and plipastatin) fashioned misperception due to the same group of biosurfactant and approximately similar physicochemical properties were differentiated through the encoding of genes and their molecular weights. In fact, both microorganisms fengycin and plipastatin associated with D-tyrosine in the peptide linkage of lipopeptides were encoded using two different genes i.e. fen B and pps D.101 To elevate the production of biosurfactant, a specific design was introduced for fengycin using Bacillus megaterium microorganisms via the impact of nitrogen and oxygen sources.102
A few years later, Rangarajan et al.103 introduced a new idea about green silver nanoparticle synthesis using the incorporation of fengycin (40% w/w) and surfactin (60% w/w) as capping and stabilizing agents, respectively. The pore size and morphology of nanoparticles principally depended upon the initial concentration of biosurfactant and synthesis methods. It was observed that the increment in the surfactant concentration changed the nano-sphere nanoparticles to varied shapes and detached from the vesicles at last. The stabilization of nanoparticles was predominantly observed for 6 months with no lacking of solvation via the suspension in the sodium chloride solution. Fengycin was still not entirely developed in the biosynthesis of nanoparticles. In one of the recent publications, the authors have proposed the formation of AgCl-NPs due to C
O and –OH functional groups on amino acid residue in fengycin (Thr and Tyr) lipopeptide biosurfactant via the electrostatic interactions.104 It was subjected to improve the synthesis of fengycin-mediated nanoparticles due to easy impregnation of C
O and –OH functional groups with metal precursors.
3.6. Kurstakins
In the year 2000, the Bacillus thuringiensis was used for the first time to produce the kurstakins biosurfactant as Thr–Gly–Ala–Ser–His–Gln–Gln sequence, where the threonine linked with fatty acids in the presence of lactone linkage105 (Fig. 1). The scattering of Bacillus thuringiensis in insects expressed a great asset of kurstakins, such as protection from fungi against Stachybotrys chartarum, absorption on the surface of spores to kill the inhibited or, grown part through the cell wall, where this bacterium disseminates with nature economically.106,107 The structural diversity of kurstakins biosurfactant developed by Bumpus et al.,108 which was ensured through a particular advanced analytical instrument MALDI-TOF-MS (Matrix-Assisted Laser Desorption/Ionization-Time of Flight Mass Spectrometry) analysis. Furthermore, Hathout et al.106 employed the antifungal activity against a specific fungi Stachybotrys charatum in the presence of purified kurstakins biosurfactant, whereas Abderrahmani et al.109 debated that other types of bacteria were unable to exhibit the antifungal activity due to the formation of pores with a limited range of activity. As a major disadvantage of this biosurfactant is that research groups are not concentrating on this biosurfactant significantly. However, the most interesting functioning, the presence of histidine (amino acid) in kurstakins, was employed in secreted molecules to improve the affinity of the membrane, which could be accomplished to incorporate with the inorganic precursor and produce green nanoparticles.110 It could be also predicted that the kurstakins was still not entirely developed in the biosynthesis of nanoparticles, which might be due to lack of a scientific approach or, less significant in the synthesis of nanoparticles (Table 2).
Table 2 List of green nanoparticle synthesis using different biosurfactants and conditions
Microbes |
Media |
Biosurfactant type |
Precursor |
Metal NPs |
Temperature (°C) |
NPs shape |
Pore size (nm) |
Proposed applications |
Ref. |
Bacillus subtilis (BBK006) |
M9 |
Surfactin |
HAuCl4 |
Au |
4 & RT (room temperature) |
Spherical |
4–13 |
Antibacterial and antiviral |
63
|
Bacillus subtilis (BBK006) |
M9 |
AgNO3 |
Ag |
4 & RT |
Spherical |
4.3–17.8 |
— |
62
|
Bacillus amyloliquifaciens KSU-109 |
LB |
Cd(NO)3 |
CdS |
RT |
Cubic/hexagonal |
3–4 |
Bioremediation and mineral exploration |
64
|
Bacillus subtilis (BBK006) |
M9 |
Ca(NO3)2·4H2O |
Brushite |
RT |
Nanosphere, oval and nanorods |
50–400 |
Environmental and biomedical sciences |
23
|
Bacillus subtilis (BBK006) |
M10 |
Ca(NO3)2·4H2O & (NH4)2HPO4 |
Hydrox-yapatite |
90–150 |
Rod |
78–124 |
Fluoride removal and environmental pollution |
65
|
Bacillus subtilis CN2 |
Mineral salt medium |
AgNO3 |
Ag |
RT |
Spherical |
21 ± 2 |
Antibacterial activity and treatment of multidrug-resistant bacteria |
66
|
Bacillus subtilis (BBK006) |
M9 |
Si(OC2H5)4 |
BMSN |
RT |
Spherical |
14 |
Antimicrobial activity, drug delivery, CMC, (anticancer activity) and oil spill management |
17
|
— |
— |
Daptomycin |
AgNO3 |
D-AgNCs |
RT |
— |
|
Antimicrobial activity |
72
|
Trichoderma reesei (NCIM 992) |
Mold growth medium |
HAuCl4 & AgNO3 |
Ag–Au bimetallic |
28 |
— |
25–40 |
Antimicrobial activity, therapeutic, and skin infection |
73
|
— |
— |
Ag nanocubes and HAuCl4 |
AuNC@PDA |
4 |
— |
38–55 |
Multidrug resistance treatment |
75
|
— |
— |
Graphene oxide-dopamine |
Graphene oxide membrane-daptomycin |
37 |
— |
— |
Wound healing disease |
77
|
Bacillus subtilis CCTCCM207209 |
— |
Iturins |
AgNO3 |
Ag |
RT |
Spherical |
20 |
Food preservation, antifungal and antibacterial |
111
|
Bacillus subtilis CCTCCM207209 |
Mineral salt solution |
Iturin A |
AgNO3 |
Iturin-AgNPs |
RT |
Spherical |
10–30 |
Protection from bacterial and fungal contamination in food |
85
|
Bacillus subtilis CCTCCM207209 |
Mineral salt solution |
Chitosan and AgNO3 |
Iturin-AgNP CS |
RT |
Sponge-like |
20 ± 10 |
Antibacterial and wound healing-promoting effect |
87
|
— |
— |
Chitosan and succinaldehydic acid |
SAC-CS-IA |
70–130 |
Spherical |
200–300 |
Agriculture and food industries |
88
|
C. albicans CICC32380 |
Potato dextrose broth |
Iturin |
Chitosan and AgNO3 |
Iturin-AgNP CS |
25 |
Spherical |
20 ± 10 |
Antifungal activity and skin wound healing |
86
|
— |
— |
Echinocandins |
AgNO3 |
Echinocandins-AgNPs |
RT |
Spherical |
80 |
Clinical treatment of infections and new drug development |
96
|
Bacillus
|
— |
Fengycin and surfactin |
AgNO3 |
Fengycin and surfactin-AgNPs |
4 |
Spherical |
22 ± 4.4 |
Antimicrobial activity |
103
|
Rhizospheric bacteria
|
Nutrient broth |
Fengycin |
AgNO3 |
Fengycin-AgCl NPs |
30 |
Spherical and oval |
5–35 |
Development of promising nano pesticides against phytopathogen plant disease management |
104
|
4. Biosynthesis of nanoparticles and their reaction mechanism
Microorganisms generally require carbon (C) and nitrogen (N) sources for their cellular growth and production of biosurfactants at optimum pH and temperature. The biosurfactant (lipopeptide) production was frequently controlled at the optimum pH value using different solvents such as HCl and NaOH. The green nanoparticle synthesis was pointedly prepared via the interaction of the metal precursor with capping or, stabilizing agent in the presence/absence of catalytic components, where various types of biological capping or, stabilizing agents comprise N and C sources such as proteins, glycosides, peptides, prokaryotic cell, eukaryotic cell or, plant extracts.112 There are numerous methods proposed for nanoparticle syntheses, such as precipitation, the reduction reaction, and complex formation reaction mechanism,113 where the major key point of nanoparticle synthesis is to reduce the charged ions of a precursor using microorganism/chemical components.114 In recent years, a large amount of nanomaterials production has been reported using suitable and low amounts/number of microbes via a less time-consuming process, such as for Au, Ag, and Zn nanoparticles.115 It accomplished a strong connection between nanotechnology and biotechnology and implemented a great impression in green chemistry.2,116 A great selectivity of biological raw materials (natural resources) has been introduced as a most common option in the synthesis of metal nanoparticles due to its economical, dominant, less expensive, and eco-friendly nature.117–119 Alternatively, the green nanoparticles are also produced directly using the supernatant of bacteria, yeast, marine algae, viruses, actinomycetes, and fungi.120,121 Moreover, it was accumulated in the elemental form through a bio-reducing agent with the help of microorganism cell metabolic properties. The physicochemical properties of synthesizing nanoparticles (e.g., morphology, structural elucidation, nature of particles, etc.) are primarily subjected to the temperature, reaction time, pH, and carbon chain length of biosurfactant.121–123 Lipopeptide (biosurfactant) is stimulated through extracellular and intracellular activities in the formation of nanoparticles, where the extracellular synthesized nanoparticles are usually indicated by less pore size as compared to intracellular mediated nanoparticles.124–126 Remarkably, intracellular synthesis required more additional steps to produce the nanoparticles than extracellular synthesis such as ultrasonication or, using appropriate detergents.127,128 However, a wide range of mechanisms for the biosynthesis of metal nanoparticles has still not been proposed. The predicted mechanisms of extracellular and intracellular synthesis of green nanoparticles are shown in the following Fig. 2.
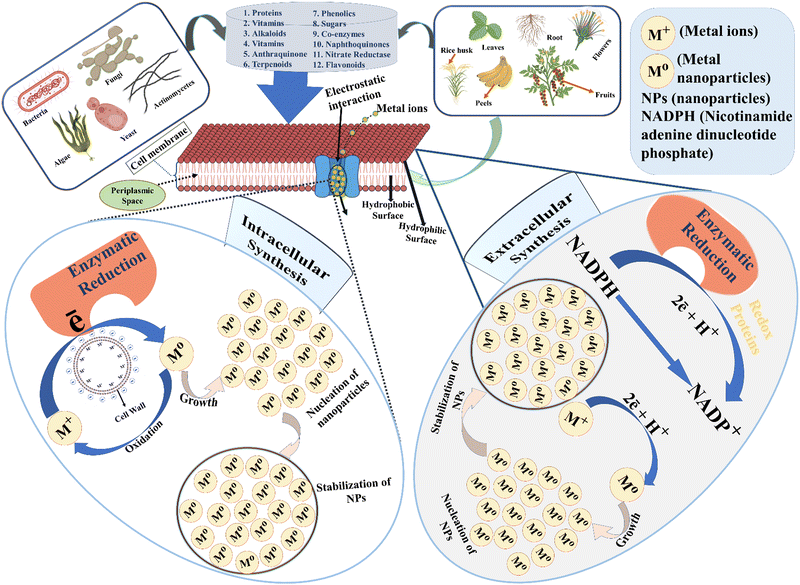 |
| Fig. 2 Reaction mechanism of green nanoparticles synthesis using biosurfactants. | |
4.1. Extracellular synthesis
Extracellular biosynthesis occurs outward of the bacterial cell wall via extracted cell-free or, supernatant of cultured bacteria, the biomass of microbes.129 In this case, the microbes were inoculated in a sterilized plate or, flask to uninterruptedly grow the bacteria. Approximately, two more/fewer day cultures of microorganisms were located in an incubator to attain optimal conditions at the highest optical density (controlling the temperature, medium, and pH) and further centrifuged to eliminate the biomass from the microbial surface.120 In the presence of extracellular enzymes, biosynthesis of nanoparticles from lipopeptide biosurfactant furnished bio-reduction of metal ions to metal nanoparticles.130,131 This type of metallic nanoparticle synthesis is responsible for electrostatic interaction with proteins and DNA, which occurs at the overside or, secretion of enzymes.132,133 Moreover, the enzymatic reduction was further processed to aggregate the nanoparticles through the nucleation process due to the existence of a condensation reaction. The stabilization of the formed nanomaterials has been employed through extraction or, purification process, where the filtration (membrane) and detergents are mainly used for their purification and, also possible to recycle the microorganisms in this biosynthesis.134 Recently, most of the researchers have focused on the green synthesis of silver nanoparticles through extracellular activities due to the advancement of the surface properties and their massive applications in nanobiotechnology, such as Bacillus sp. GP-23 executed the biosynthesis of spherical-shaped AgNPs using a marine soil source and also deliberated the fungicidal effect towards Fusarium oxyporum (disease of tomato, sweet potatoes, banana, cucurbits, tobacco, and legumes).135 Various strains of Bacillus have been isolated and employed in the synthesis of AgNPs, such as B. pumilus, B. persicus, and B. licheniformis. In this case, primarily, the hydroxyl group participated to oxidize and form the nanoparticles due to the reduction of silver/metal precursor ions.136 Further studies have also been carried out on lipopeptide biosurfactant-based synthesis of various nanoparticles, such as Au, ZnO, TiO2, Fe2O3, Cr(III), CuO, and CdS NPs.137–144
4.2. Intracellular synthesis
The intracellular synthesis of green nanoparticles using biological entities recognized emerging ways of progression in green chemistry. In this case, the intracellular synthesis occurs inside the bacterial cell wall, where intracellular enzymes and functional groups of the positive surface come close to attract metal ions via electrostatic interactions and reduction occurs in the cell membrane of the periplasmic space.126,129,145 A new type of microbial synthesis of nanoparticles by the intracellular method was proposed in the 20th century by Singh et al.146 The nanoparticle synthesis using lipopeptide biosurfactants was restricted to the pH value range, e.g., Bacillus subtilis used the pH ∼ 7 for the optimal growth of bacteria. This method comprises the interaction mechanism between the metal (cationic form) as a precursor and the cell wall as a ligand (anionic form).147 In the case of the intracellular procedure, the biosynthesis of nanoparticles was exhibited in two ways: (a) biomass-mediated nanoparticle synthesis (b) supernatant mediated nanoparticles synthesis; some research groups have reported that the biomass was collected and sterilized to reduce the external unwanted effects, and was incubated in a metal precursor solution to produce nanoparticles. On the other hand, the supernatant of the culture medium plays an important role in nanoparticle synthesis. There have been two processes developed in green nanoparticle synthesis using supernatant: (a) direct use of supernatant (b) supernatant to biosurfactant production; the direct immersion of supernatant comprises appropriate components of enzymes and other essential reducing agents, which further emerged in metal solution and, stirred at optimum temperature and pH to synthesize the nanoparticles. However, the potential reaction mechanism is difficult to elaborate on due to the significant unrecognizable amino acids in this process. In the provision of supernatant for biosurfactant production, the microorganisms are removed through the centrifugation process from the culture medium and resuspended biomolecules in the supernatant by emerging different appropriate solvents via the specific control of pH using an acid or, base. The resuspended biomolecules are centrifuged and extracted for further drying for biosurfactant production in a freeze dryer. The formed lipopeptide biosurfactants were optimized by controlling different parameters of operating conditions, such as medium composition, agglomeration, the solubility of chemical components, temperature, pH, and salinity.148 Most notably, some of the chemical components reacted with each other and produced precipitation in the media solution, which rapidly restricted the growth of the bacteria. In the case of nanoparticle synthesis, the produced lipopeptide biosurfactants emerged in a metal precursor solution to reduce the pore size and advanced their morphology. In addition, the resultant metal ions are condensed into their lower oxidation states with increasing of stirring time as well. In this approach, the mixed solutions are generally stirred longer to enhance the homogeneity of particles as the higher production of silver nanoparticles was successfully employed through the intracellular method with a spherical crystalline structure using microalgae, which produces a reasonable amount of biomass in this microbial synthesis.149 Recently, intracellular synthesis has also been enlightened severely due to its very useful and eco-friendly nature in some specific synthetic parts of living organisms, such as AgNPs (Lactobacillus casei), Au, ZnO, Ti, TiO2, CdS, Al2O3, CdSe and Se NPs.150–158
5. Factors influencing microbial nanoparticle synthesis
5.1. Surface and interfacial tension
The surface and interfacial tension effect play a very crucial role in the biosynthesis of microbial-impregnated nanoparticles. Generally, a good biosurfactant always upsurges the surface area to diminish surface tension at the interface of liquids, where the non-miscible oil and water molecule comprise approximately 1 and 30 mN m−1 in a solution to sustain the surface effectiveness.159 The most prominent example of low surface tension of soap nut extract has been reported by Potočnik et al.,160 where the surface tension was detected at 22 mN m−1 at a higher concentration (10%). In addition, other natural plant extracts have been testified and produced the lowest value of surface tension such as quillaja bark extract, sapogenin, escin (23 mN m−1), and standard quillaja saponin, the horse chestnut extract (25 mN m−1). Nevertheless, the lowest value of the surface tension demonstrates that the natural plant extracts could have a better potential for surfactant and nanoparticle synthesis in the future. In this case, the biosurfactant (lipopeptide) mediated nanoparticles could be more efficient in diminishing the surface tension. The surface tension was significantly observed through the specific analytical instrument stalagmometer. The surface tension of the supernatant was calculated using the following formula:
where, γ0 & γ indicate the surface tension of the liquid sample and supernatant, n is the number of drops of the liquid sample, and n0 = the number of drops of supernatant.161,162
In recent research, most research laboratories prefer the Wilhelmy plate method to analyze the surface tension of liquid samples through a Krüsh K11 tensiometer at room temperature.163 The critical concentration and particle size of nanoparticles have been executing a significant effect due to the integration of surface tension. Besides, the heightening of surface tension is mainly responsible for the van der Waals interaction between the interfaces of gas or, liquid, and is also accountable to increase the surface free energy. Desai et al.164 suggested that a highly efficient lipopeptide biosurfactant could easily reduce the surface tension more than two times in the case of water molecules. Furthermore, Novikova et al.165 investigated the substantial effects of surface tension in nanoparticle synthesis, where they designed the most important method for producing a porous matrix. This method deliberated to grow the isolated particles, allowed as a driving force to reduce the aggregation of nanoparticles (e.g. CuNPs). Progressively, Chinnam et al.166 revealed another mitigation option in the acceleration of temperature and fraction of volume that could diminish the surface tension of nanoparticles, such as SiO2, Al2O3, ZnO, TiO2, etc. An alternative report by Binks et al.167 suggested the irreversible adsorption process, emphasizing maintaining the liquid–liquid interfaces for the adsorption of nanoparticles rather than the solid–liquid interface, which generally requires higher surface energy to control their interfaces. However, neutral and moisturized particles in the liquid–liquid phase will not be directly influenced by surface tension. In this case, the surface energy will be disturbed and surface tension will be ineffective. To control the morphology and efficiency of the synthesized nanoparticles, these types of substantial and influential methods could nourish a great imprint with low surface tension in recent research fields.
5.2. Critical micellar concentration (CMC)
The critical micelle concentration (CMC) is defined as a minimal concentration in biosurfactants, where the micelle formation occurs over the accumulation of biosurfactant molecules at the interface of samples.168,169 The non-polar (hydrophobic) part of the biosurfactant encapsulates the oil droplet in the central region of the micelle, whereas, the polar (hydrophilic) part of the biosurfactant molecule possesses the outer shell of water to sustain the promising interaction with water in its substantial growth of micelle formation.170 In nanotechnology, CMCs are directly associated with surface tension at their liquid interface. The significant property of the critical micelle concentration is to enhance the solubilization capacity and detergency on the liquid surface.171 Theoretically, the efficiency of the molecule is inversely proportional to the critical micelle concentration, and highly efficient molecules of the sample solution require a very less amount of the biosurfactant to diminish the surface tension.172 In a recent publication, Su et al.173 introduced the aggregation and dissociation factors during the nanoparticle synthesis technique (liquid state complexation) through reflection priority in molecular building blocks. The threshold concentration in the particle synthesis signified an important character for controlling the aggregation and formation of well-distinct structures. The optimum or less value of threshold concentration fashioned less agglomerated particles with well-organized nanostructures, whereas the higher threshold concentration improved the aggregation of particles to form stable and less reactive molecules. On the other hand, the critical micelle concentration encompassed a great disadvantage due to sudden variability in physical properties such as turbidity, density, osmotic pressure, conductivity, viscosity, and chemical shifts of the sample solution. The CMC in lipopeptide biosurfactants (surfactin, iturin A, and fengycin) have significantly been described as more interesting and resourceful than other biosurfactants in the petrochemical industries. Interestingly, the surfactin was sustained 10–40 times with lower CMC at minor purity compared to the chemical surfactant.174
5.3. Hydrophilic–lipophilic balance (HLB)
The HLB is another type of important character, which is implemented in the specific selection of biosurfactant.175 In fact, it demonstrated the properties of the biosurfactant in the form of lipophilicity or, hydrophilicity176 and its value/number is principally accountable upon the emulsion (water in oil and oil in water), where oil in water is thermodynamically unstable.177 As a result, oil in water has a more favorable high HLB value, whereas water in oil has a low value in HLB. The lower values (3–6) of HLB are generally preferred for W/O (W-water; O-oil) due to the greater stability of lipopeptide biosurfactant production. Conversely, the higher values (8–18) of HLB are needed for the formation of O/W microemulsion, which are less considered in the production of lipopeptide biosurfactants with less stability. To overcome the higher HLB values, an additional co-surfactant is required to diminish the HLB value for better emulsification.177,178 Vital attention to HLB value in nanoparticle synthesis influences on the size variation, which is considered to be a substantial impact on the relative affinity of surfactants in both the phases O/W or, W/O.179 In the case of the self-assembly method, the structure and morphology of materials were pointedly biased in self-assembled particles/polymers due to HLB values.180–183 In between, Zhang et al.184 introduced an important platinum plate method to evaluate the surface tension of nanofluid materials. The authors concluded that water played a very crucial role to interrupt the surface tension while being positively/negatively adsorbed on the surface of hydrophilic–lipophilic nanofluids materials. Gradually, Lian et al.185 generated informative data on HLB in the case of composite films using an oil/water emulsifier, where the HLB was not only influenced by the pore size of nanoparticles but was also influenced by antioxidative activity, physical properties, antimicrobial activity, and their shape of developed nanoparticles. Remarkably, the modification of HLB values in nanomaterials synthesis was implemented to boost the production of oil recovery and contact angle in water.186 In the same year, Abdul Karim et al.187 observed and studied the micro-explosion occurrence of numerous size emulsion droplets through HLB values. The dosage of surfactants was mainly accountable on waiting time of this process. The HLB values of surfactant are shown in Fig. 3.
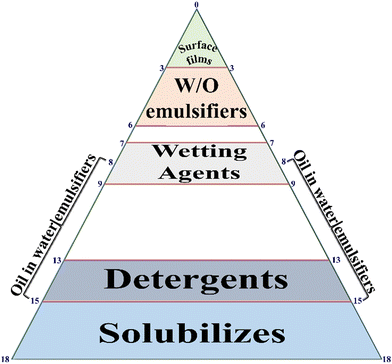 |
| Fig. 3 HLB value diagram. | |
Griffin et al.188 established the various kinds of substrate molecules using a significant formula to calculate the theoretical value of HLB such as:
(a) Polyoxyethylene alkyl ethers and polyoxyethylene esters
where,
E is the mass (or, weight) percentage of oxyethylene content.
(b) Polyhydric alcohol fatty acid esters
where,
S is the saponification value of the ester and
A is the acid value of acid.
(c) Polyoxyethylene chains and polyhydric alcohols
where,
E is the mass percentage of oxyethylene content and
P is the mass percentage of a polyhydric alcohol.
(d) In the case of a mixture solvent, the HLB value is investigated using the mass percentage (%) of solvents (such as surfactants). The HLB values were explored using the following formula;
where HLB
x = low HLB surfactant, HLB
y = high HLB surfactant,
x% and
y% = mass percentage
5.4. pH and temperature possessions
The influence of pH and temperature is exhibited as one of the most important aspects in biosurfactant production and nanoparticle synthesis using microbial surfactants (lipopeptide). In most cases, the value of pH and temperature is not affected by the change in environmental conditions.189 However, the cellular growth and production of lipopeptide biosurfactants have been varied at significant pH and temperature values, where the functional variability depends upon the selectivity of strain-to-strain for specific growth. Qin et al.190 and Jahan et al.191 introduced the effects of pH on the most desirable silver nanoparticles, which influenced their shape and particle size distribution due to the alteration in pH values. Generally, the production of lipopeptide biosurfactants was suggested with pH and temperature range of 3–8 and 25–37 °C for higher production rate192,193 such as surfactin production using Bacillus amyloliquefaciens cultured at optimal temperature 30 °C,57Bacillus subtilis TD 7 cultured at 37 °C194 and Bacillus subtilis 168 cultured at 37 °C.195 Additionally, some of the lipopeptide biosurfactants were also produced at more than 37 °C temperature, such as thermophilic bacillus spp. (40 °C).196 However, the optimum temperature range was exhibited 25–37 °C for the active and precise growth of microbes encompassing the high population. The production of biosurfactants is also subjected to the total solvent accessible surface area, where, in general, the surface area of surfactin is inversely proportional to the growth temperature.197 Conversely, the intramolecular and intermolecular hydrogen bondings of the surfactin biosurfactant (lipopeptide) are usually interrupted at high temperature.
5.5. Emulsification and de-emulsification
A process of two different immiscible liquids (emulsion: volatile poorly water-soluble or, insoluble, and aqueous solvent) are mixed together to evaluate the emulsification index, such as in water and oil.198 Researchers have executed several types of stabilizers in water to enhance emulsification. In fact, when the polar solvent in a polymer solution and the aqueous phase are mixed together, the emulsifying drop and aqueous phase are dispersed through the polar solvent. As a result, the polymer and active constituents are precipitated out easily in the form of nanoporous.199 However, in the formation of the microemulsion, the biosurfactants have the capability the provision non-ionic and double-chain hydrocarbons, whereas single-chain hydrocarbon requires co-surfactants supported biosurfactants.200 The emulsification index (E24) formula was used to calculate the emulsification capacity of various hydrophobic substrates, which is given as follows:201
where, HEL: is the height of the emulsion layer and Hx: the height of the total liquid column.
In the current research arena, nanoemulsion and membrane emulsion have been predicted as potential ways to signify the effects on nanoparticle synthesis. Alliod et al.202 introduced the membrane microemulsion to control the particle size distribution through Ostwald ripening process. Progressively, the authors also reported that the premix and their storage in stress conditions have revealed better stability than the parental nanoemulsion mixture. Alternatively, most pharmaceutical industries prefer the reverse emulsification process due to easy control of the size of emulsion droplets and the less time-consuming process.203 Almost all industries are preferring and challenge nanoparticle synthesis at a very low cost, the easiest way, and with green approaches. In the meantime, Kaltsa et al.204 stated the homogeneous emulsification effects in the nanomaterials study by reducing the concentration of the oil phase, employing the surfactants/co-surfactants, intensifying the stirring rate, implementing high pressure, etc. Conversely, de-emulsification is the process to separate the mixture of two immiscible liquids into functional biosurfactants,205 where it is mainly used in the recovery of products206,207 such as electrical treatment, heat treatment, oil-containing centrifugation, chemical extract holding fatty acids, soaps and long chain alcohols.208 Moreover, the de-emulsification activity of floating cells was observed to be higher than that of pelleted cells due to the hydrophobic character at the oil-water interface.209,210 Recently, the de-emulsification process has been employed in the food, pharmaceutical, cosmetic,159 and petroleum industries.211 The influences of de-emulsification not only comprise the recovery rate but also advanced magnetic properties and high surface area.212
5.6. Toxicity
A unique property (physical/chemical) of green synthesized materials is its emergence as an excellent technique to be useful in various medical, and also in environmental applications to improve their popularity in scientific research. The implementation study and reports on toxicity suggested that green nanoparticles have still caused adverse properties to humans as well as the environment. De Souza et al.213 introduced the synthesis of green nanoparticles using plants, bacteria, and fungi, demonstrating that these are vital and innovative ways to control the toxicity, which can produce a large-scale of nanoparticles (e.g. Ag NPs) at the industrial level and, minimize the adverse effect of toxicity on humans and the environment. In the case of lipopeptide biosurfactants, it was observed less toxic with greater surface activity and are highly environmentally favorable than chemical surfactants to synthesize the nanoparticles.17 The periodic dose of chemicals and their response study was only the key option to govern the suitable amount of toxicity in materials over a period of time. The inappropriate drug amount, material size, shape, and specific density caused the heightening of agglomeration and aggregation of nanoparticles, which are responsible for interfering with the proper diffusion of membrane for leading the target sites of cells/organs in living organisms (e.g. humans or, animals). Apart from that, the overdose of drugs impregnated with nanoparticles at the targeted sites concerned enhanced the aggregation/agglomeration and their toxicity, where the drugs and vaccines accomplished a contradiction in the toxicity between each other. These drugs are used in multiple doses to decrease the transformation of infectious diseases from one organism to another. Conversely, the vaccines are generally used as a single dose to reduce the transformation of infectious diseases with lower toxicity than drug toxicity.214 Recently, most industries have been recently using biosurfactants to reduce toxicity, such as the food, pharmaceutical, and cosmetics industries. In the current research arena, most biosurfactants are formed from industrial waste to diminish the pollution (e.g. water) in the environment.159 Furthermore, Oberdörster et al.215 proposed another most important reason for the toxicity hypothetically and experimentally, where the dimensions of the surface area and surface energy could be responsible for elevating the toxicity, and hypothetically, it could not be unreasonable that an unprotected surface reaction with cell/organs stepped up the toxicity. In this case, the decrement in the particle size or, increment in the individual particle concentration would be accountable to integrate the toxicity at a significant position. However, some important toxicity types have been proposed in the synthesis of nanoparticles, such as nephrotoxicity, cardiotoxicity, neurotoxicity, cytotoxicity, myelotoxicity, and genotoxicity.216–221
6. Current applications in biomedical and environmental nanotechnology
The development of pharmaceutical and environmental studies exhibited an indiscriminate way to support the scientific community and industrialization over the years. In the recent nanobiotechnological field, the development of green nanoparticles has received substantial consideration to exhibit cost reduction, ecofriendliness, lesser toxicity, flexibility in biodegradability, and easy availability, as compared to chemical components synthesized nanomaterials in biomedical and environmental applications. The green nanomaterials also exploited an exceptional surface activity and sensing ability by controlling the pore size, surface area, and toxicity using catalysts or incorporation of metal nanoparticles in environmental remediation. The most crucial current biomedical and environmental applications in nanotechnology through lipopeptide-mediated nanoparticles are shown in Fig. 4.
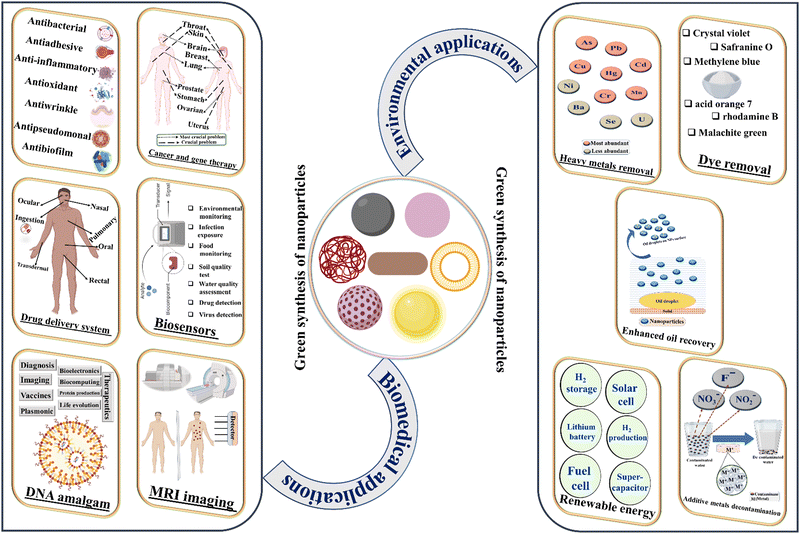 |
| Fig. 4 Recent applications in biomedical and environmental sciences. | |
6.1. Biomedical applications
The surfactin-mediated synthesized nanoparticles were used in different applications such as drug delivery systems, biosensors, MRI imaging, and cancer treatment.84 Moreover, the incorporated surfactin and nanoparticles are used as good carriers in drug delivery applications due to the efficient binding of materials, where the surface was modified with the help of directing ligands and significantly delivered with a higher amount of surfactin to the targeted site of cancer cells.55 Particularly, the sustainability of biosurfactant-mediated particle synthesis was dominated in medical applications such as anti-oxidant, antiwrinkle, moisturization, antibacterial, anti-inflammatory, anti-mycoplasmal, anti-adhesive, cytotoxicity.82,222–224 In recent decades, researchers are focusing on the most relevant biosurfactant (surfactin) to defend the surface of biomedical devices and biomaterials, such as surgical instruments, where this instrument is greatly suitable for antibiofilm and antiadhesive in the biomedical science.225 Moreover, the surfactin-based nanomaterials were used to treat the periodontitis disease at a specifically targeted position efficiently and with less tissue damage.226 The various types of surfactin-mediated nanomaterials were introduced in medical science applications, which demonstrated a high demand in the global market.227
6.1.1. Antimicrobial and anti-inflammatory activity.
The resistance of bacteria has shown to be a critical problem due to improper explanation of the reaction mechanism of antimicrobial activity and unsuitable selection of nanomaterials in the medical field.228 There have three major models exhibited, i.e., oxidative stress induction, metal ion release, and non-oxidative process. An exclusive example of chronic diseases reported a major issue due to bacterial infections, where a lot of researchers are working to produce highly efficient and less expensive antibiotics. Gradually, the involvement of surfactants as catalysts in nanoparticle synthesis improved the antimicrobial effects in the treatment of chronic and acute diseases. However, still, the efficiency of the nanoparticles was not improved to the optimum position and comprises toxicity behavior. In the current research arena, several studies have been also directed to produce biosurfactants to downgrade the toxicity and proliferate the efficiency of antibacterial activity. On the other hand, green synthesis of nanoparticles using biosurfactants has been providing a wide range of and more significant antimicrobial activity and has endeavored to the emergence of multidrug-resistant bacterial strains. Silver nanoparticles were synthesized using the Iturins biosurfactants by Zhao et al.,111 where the formed nanoparticles have a strong influence on inhibiting bacterial growth and potential to treat wound-healing diseases in vivo. The nanoparticles were also implemented for the treatment of wound healing on the back side of rats. Bezza et al.,66 in a recent study, the microbial synthesis of Ag nanoparticles was reported using the lipopeptide biosurfactant through the reverse micelle method and was shown to possess a more significant antimicrobial character as compared to bare silver nanoparticles. Furthermore, the metal-based microbial biosynthesized nanomaterials instituted a wide range of customs in biomedical science and engineering. On the other hand, the production of these bio-nanomaterials (AgNPs, AuNPs, ZnONPs)229 and CuONPs has advanced in the global market due to better substantial antimicrobial outcomes against Gram-positive and Gram-negative bacteria in the last few years.230 However, the synthesis of nanoparticles using biological surfactants is still not fully developed to understand clearly the interaction between particles and bacterial infection cells in physiochemical and antimicrobial properties.
A lot of research has been focused on alternative medical remedies to control the inflammatory cytokines or, inflammatory intermediaries.231–233 The development of the inflammation method has received considerable attention to cure chronic disease, tissue damage, muscle contusions, or mutual muscle problems during sports activity.234,235 Consequently, some of the anti-inflammatory drugs could relieve the symptoms for a short time and have substantial side effects during treatment, which is predicted to be inadequate for chronic diseases. Further studies on green nanomaterials have targeted to reduce these extensive problems and received a more convenient way of treatment with insignificant side effects. Recently, Johnson et al.226 reported that surfactin-loaded κ-carrageenan oligosaccharides-linked cellulose nanofibers nanoparticles diminished the biofilm development and metabolic bacterial activity, confirmed through crystal violet and MTT assay. The author also observed the inhibition effects with two different bacteria Fusobacterium nucleatum and Pseudomonas aeruginosa, which led to an increase in the oxidative stress in the bacteria. Most importantly, the ROS production and transcription factor was diminished in the case of surfactin and nanoparticle-mediated anti-inflammatory agents. However, this type of nanomaterial is certainly supported as a potential candidate to treat the most dangerous diseases such as periodontitis. Gradually, eco-friendly research has been regulating the current era of industrial life to reduce these types of uncontrollable diseases.
In the current research field, an anti-pseudomonal is one of the stimulating classifications of antimicrobial activity and has been distributed into two major classes, i.e., antipseudomonal penicillins and antipseudomonal antibiotics.236,237 Krishnan et al.238 introduced the surfactin-mediated silver nanoparticles/nanocubes synthesis to study the in vivo properties of anti-pseudomonal and anti-endotoxic effects. The authors mentioned that there is no appropriate treatment available for diabetic foot infection, where the synthesized green nanoparticle deliberated the degree of wound infection area (e.g., studied details in case of diabetic mice). In addition, the wound repair properties were also observed through the observation of mRNA expressions, bacterial counts, and histopathology. The results showed that the novel treatment of diabetic wound infection accredited well cured and immense suggestions for further innovative research with prepared nanomaterials.
There are several applications of synthesized material by daptomycin, for example, ocular treatment of the eye,239 inducing the destruction of DNA, antimicrobial, drug delivery,240 Gram-positive bacterial infection,241 treatment of MRSA (methicillin-resistant Staphylococcus aureus) infection31 and artificial grafting of bones.242 Furthermore, it has been reported in the bio-nanotechnology field that Staphylococcus aureus aids in the bovine mastitis treatment,243 treatment of bacterial infection via biofilms,244 polydopamine coating,245 and wound dressing material.246 Alternatively, Kundu et al.247 focused on the study of bactericidal properties against E. coli bacteria and their cytotoxicity, conclusively, cell death was observed at approximately 80% at higher concentrations of nanopolymers (i.e., 300 μg mL−1) and around 45% of haemolysis at a very high concentration 500 μg mL−1.
6.1.2. Antioxidant activity.
Currently, the antioxidant employed plays an important role in all living organisms as an oxygen-repellent or, hydrogen donor in the cellular body. A few years ago, a species of lipopeptide category Bacillus methylotrophicus DCS1, was synthesized to be used as an antioxidant to inhibit the β-carotene bleaching through linoleic acid. Furthermore, the DPPH (2,2-diphenyl-1-picrylhydrazyl) was employed to encounter the hydrogen donor and scavenged the radical via the peroxidation process. However, the Bacillus methylotrophicus DCS1 is reported as an efficient antioxidant, where the development of the biofilm and the adhesion of microbes are diminished by DCS1 in the field of biomedical science and food chemistry.248 Progressively, the Bacillus subtilis RW-I-based lipopeptide biosurfactant was reported as an alternative antioxidant molecule, which could accomplish a better antioxidant capacity in future study.249 Skorochod et al.250 reported one of the most important applications using nano-SiO2, where the 4.5–11.8% of antioxidant potential was diminished through nano-SiO2 of Bacillus subtilis IMV B-7023. On the other hand, the green nanoparticles showed a significant equilibrium amongst antioxidative protection of cells and boost the antioxidant potential through oxidative stress.251 The cause of the oxidant revealed a positive reduction potential and integrate cellular death, where the antioxidant was implemented to prevent cellular death by inhibiting the oxidation of used substrates. The reactive oxygen species (e.g. ˙OH, O2˙−, non-radicals: H2O2, organic peroxides) and reactive nitrogen species (NO˙, NO2˙, etc.) embodied the most significant groups in oxidants group.252–254 In the upcoming research scope, the biosurfactant mediated nanoparticles could show entire performance to improve the antioxidant activity, which would be a great achievement in medical applications.
6.1.3. Antitumor and cytotoxicity activity.
Generally, the antitumor activity has been used to reduce tumor growth through the direct/indirect killing of tumor cells in living organisms. Recent studies have specified that the formulated metal nanoparticles reported a good antitumor activity against many cancer cell lines such as DOX@SUR (doxorubicin@surfactin) nanoparticles have been used as good antitumor to decrease human breast cancer, where these nanoparticles reported to grow the anticancer potential of DOX and SUR.255 Furthermore, lipopeptide-coated iron oxide nanoparticles have been synthesized in the form of antigen, which was used as the transporter for the MUC1-based (MUC indicates mucin-1 peptide) cancer vaccine. This anticancer vaccine introduced a great potential to decline the tumor cells of cancers part.256 Apart from this, Dong et al.257 represented the interesting applications of lipopeptide-mediated nanoparticles, which have been used as a carrier for specific siRNA delivery, and could be significantly useful in the treatment of genetic disorder problems (e.g. liver genetic disorders). Gradually, the antitumor activity of green nanoparticles is still unclear with lipopeptide biosurfactants. Recently, there is a great demand for microbial-based nanomaterials in the global market because these materials are highly favorable for green nanotechnology and easily decrease the tumor cells with less toxicity/time/expensive or, biodegradable, great optimization, etc. Interestingly, an important study has exposed the effective development of green nanoparticle Mn2+ synthesis, where the metal ion Mn2+ influenced the cellular activities of bacteria through different parameters, such as antibiotic production, mobility, and the formation of endospore and biofilm.258–260 In recent and next-generation applications in nanobiotechnology and the biomedical field, the great potentiality and stability of the promoter agent as biosurfactant-mediated nanoparticles was deliberated to implement in theranostic treatment. The theranostic treatment is one of the innovative and simultaneous treatments in therapeutics and diagnostics, leads by nanosensors and nanomedicine. Moreover, the advancement of theranostic treatment was suggested to use as a radioactive drug delivery by encoding and modification of circulation time, tissue-specific growth, and cell diffusion on the surface of nanoparticles.261,262
6.1.4. Inhibition of NDMs.
The NDMs (New-Delhi metallo-beta-lactamases) are one of the most unsafe bacterial-resistant enzymes, belonging to the antibiotics family of Carbapenems, and were detected first time in 2008 (India). Later on, in 2010, a Belgian person died due to bacterial expression to NDMs enzyme, and other people also died simultaneously due to this uncontrollable infection.263–266 The gene of NDMs can easily spread from one strain of bacteria to another strain via horizontal gene transfer.267 Recently, Kumar et al.268 reported novel bio-surfactin-stabilized silver nanoparticles to inhibit the NDMs harbouring cells. The author purified the NDMs to carry the metallo-beta-lactamases, which augmented the synergistic effects in their sensitivity. Results indicated that the carrying of NDMs with metallo-beta-lactamases was less efficient to inhibit through chemically synthesized silver nanoparticles, where the surfactin-modified nanoparticles were attributed to easily inhibit this complexed enzyme at very reasonable concentration due to a possible combination of thiol (–SH) group of cysteine in NDMs to non-activated enzymes.
6.2. Environmental applications
The immense evolution of biosurfactant production has increased advancement through recent studies of green nanotechnological industries; still, the development of biosurfactant-supported nanoparticle synthesis and their applications are limited. The green nanomaterials are not only implemented in environmental remediation such as wastewater treatment, polluted air treatment, or, toxicity removal from plant/soil and living organisms or human beings, but also enhance the amount of particle yield, improve the quality, and greatly useful in renewable energy at very low cost/amount by taking care of human health issues.269
Interestingly, catalysis assumes a focal job in the chemical reaction and lies at the core of incalculable synthetic conventions, from academic's research to the chemical industry level. Heightening the rate of reaction is generally caused by enzymes, or, other types of organic and inorganic catalysts. The enzyme activity plays a very important role to improve the rate of the reaction by controlling the pH and temperature effects on chemical reactions. The involvement of bacterial species in lipopeptide exhibited to produce the amylase in a short period of time and higher productivity is vastly required in various manufacturing industrial applications such as paper, detergent, food, textile, brewing, and saccharification industries.270 Commonly, the catalytic activity of lipopeptide molecules was specified better in aqueous media than in organic media.271 Therefore, in aqueous media, it is easier to prepare micelles and they can be used to increase the catalytic activity in a suitable physicochemical environment such as a specific temperature, rotation speed, and growth time. The mutual combination of micelles and self-assembled peptides chiral molecule implemented to improve the efficiency of catalytic properties of nanoparticles, such as Au nanoparticles (good packing of chiral molecules with gold nanoparticles via significant orientation). As a result, the packing materials are ideally useful to improve the enantioselectivity and diastereoselectivity in organic chemistry.272–274 Furthermore, Shamsuzzaman et al.139 reported a novel ZnO nanoparticle synthesis using green capping and reducing agents, which was subjected to less expensive, more efficient, and simple preparation using a one-pot synthesis method. Most prominently, the produced nanoparticles were shown a better catalytic activity. The authors also suggested further use in the pharmaceutical industries, light-emitting diodes/lasers, and conducting polymers. Consistently, Behera et al.275 represented a novel copper oxide nanoparticle using biosurfactant, which the authors recommended for use in photocatalytic reactions to reduce the reaction time. In recent years, Rajaboopathi et al.276 synthesized the Ag/ZnO green nanoparticles using biosurfactant, which has been recommended for a great catalytic performance and also examined under the sunlight to ensure the influence of produced material in photocatalytic degradation. Additionally, the inhibition zone of bacteria on their surface was observed higher as compared to those without biosurfactant-based synthesized nanoparticles. In recent research, the combination of gold nanoparticles with graphitic carbon nitride nanocomposite material was successfully formed using biosurfactant and has been further used to accelerate the catalysis in between organic reactions. It is mainly happening due to the synergic effects between the induction bands of both the combined nanoparticles and diminish the band gap between them.277
Unique research from Reddy et al.278 emphasizes the preparation of rose-like ZnO green nanoparticles using surfactin (templating agent) at low temperatures. The interesting data of the morphological study was observed whilst altering the surfactin concentration in the synthesis procedure. Apart from this, the depth observation of the nanoparticle structure by the authors led to a remarkable influence on their environmental application as a photocatalytic depletion study of methylene blue. In this case, the distinguished loosely-spread petals or, plate-like ZnO structures have demonstrated better photocatalytic degradation, as compared to the dense rose-like structure, where the different shape of particles was controlled through a change in concentration of precursor. In one research article, the authors reported that the synthesis of nanoparticles not only depended upon the concentration of precursor but the characteristics of the particle also depend upon the concentration of catalyst and water solution.279
A few years later, another important and exclusive study was proposed by Kundu et al.,247 which introduced eco-friendly, less expensive, and easy polymerization poly(methyl methacrylate)-surfactin green nanoparticles with the help of ultrasound-assisted emulsion polymerization method. The prepared nanoparticles were able to remove the selective heavy metal ions from water, where the affinity of metal adsorption was mainly dependent on chelating complex or, electrostatic interactions of nanoparticles with metal ions in water: Co2+ > Zn2+ > Ni2+ > Cr3+ > Fe2+ > Cu2+ > Cd2+ > Pb2+. The key benefits of this green nanopolymer were suggested for use at the industrial level due to its less expensive and no significant loss of their adsorption capacity until the four adsorption–desorption cyclization process. Moreover, the author also recommended the use of a good adsorbent for the removal of organic pollutants (i.e. phenol and β-naphthol) in this study.
One of the most interesting examples regarding surfactin-mediated novel hydroxyapatite nanoparticles was reported by Maity et al.,65 who synthesized nanoparticles using calcium nitrate and diammonium hydrogen phosphate as suitable precursors. Most importantly, the feasibility of nanoparticles was observed at three various temperatures to form a better crystal surface morphology and higher surface area. The particle size and shape were controlled by altering the synthesis temperature and pH using the hydrothermal method. Moreover, the controlled and highly pure nanoparticles were significantly used to remove the fluoride from water, which showed great potential with maximum adsorption capacity, which also indicated spontaneous and endothermic reactions. However, this nanoparticle could be the most important for environmental remediation in the upcoming research field.
In some of the recent studies, the mutual interactions between lipopeptide biosurfactants and nanoparticles have been reported as an attractive mediator to integrate the hydrophobicity and reduce the interfacial tension compared to biosurfactants.280 It was considered that the improvement of hydrophobicity could be very useful in enhanced oil recovery. Due to the significant heightening of oil recovery using this nanoparticle, Amani et al.280 have recommended their ex situ use at the industrial level, where the production rate of oil displacement using nanoparticles with microbial surfactants was almost double that of the initial aqueous solution of biosurfactants. Nanobiotechnology has been directed toward enhanced oil recovery from different resources using green nanoparticles, some of the nanoparticles have employed a very good hydrophobic behavior,281 which is greatly helpful to control the volume of oily wastewater to decrease the significant environmental issues globally.
In most of the research arena, researchers are mainly concentrating on reducing the pore size, agglomeration, and increasing the surface area of nanoparticles; however, diminishing the toxicity of nanomaterials should also be focusing upon. However, for the environmental concern, the utilization of analytical instruments, temperature, pressure, and production time must be also emphasized. Moreover, the increment of the surface area of particles can easily amalgamate with the surface of macromolecules and it might disturb the regulatory system of the body, such as an enzyme or, proteins.281 Therefore, the bioavailability of some foods is not only advantageous for well-being but also could have a negative impact on human health.282 Apart from that, the industries should also be concerned about using nanoparticles, which could be more or, less toxic than their initial properties, care should be implemented before a large scale of production and their applications.
7. Conclusions and future prospective
The tremendous development of lipopeptide biosurfactants has turned out to be promising research in the field of nanotechnology, where the natural occurrence of microorganisms, such as bacteria, algae, fungi, actinomycetes, and plants have been observed as a feasible and traditional concern for their relative ultimate production and substitution of toxic chemicals in nanoparticle synthesis. There have been two major methods developed to synthesize the biocompatible nanoparticles as extracellular or, intracellular biosynthesis methods. According to most of the research outcomes, it has been accredited that the extracellular synthesis of nanoparticles has numerous advantages such as control of the pore size, modest downstream processing, cheaper, less time consumption, convenience for industrial-level production, and support for the quick formation of nanoparticles. However, the mechanism of nanoparticle biosynthesis is still not understood accurately to specifically control the shape and size of nanoparticles. The predicted reaction mechanism was introduced in this review to understand the incorporation of precursor with biosurfactant. Besides this, the distinction in the physical and chemical properties of nanoparticles is mainly accountable to their factors affecting behavior such as surface/interfacial tension, CMC, HLB, pH, temperature, emulsification/de-emulsification, and toxicity. Correspondingly, the bio-reducing agents (template) are also responsible for the accumulation of nanoparticle production from metal ion precursor, where the cells metabolic properties played a crucial role to improve the activation power of the templating agent. The reliability, eco-friendliness, and economical biosynthesis of nanoparticles have employed a great optimistic impact on various applications in the world, especially in biomedical and environmental fields. The best availability and great production of surfactin have been regulated as the key source for nanoparticle synthesis. In this review, the incredible roles of numerous categories of specific lipopeptide biosurfactants in nanoparticle synthesis were deliberated to understand the incorporation effects of each other and study their recent applications in biomedical and environmental science. However, there are still obvious shortcomings of eco-friendly mesoporous materials to implement in commercial applications due to inadequate material performance. Therefore, some of the serious issues are still needed to resolve:
(i) The limitation of materials in industries is the biggest challenge in recent nanobiotechnology. Recently, there has been the synthesis of various types of nanomaterials using biological entities. Mass production with eco-friendly and high-quality materials is still an eventual challenge in industries. Therefore, it is required to optimize the synthesis for mass production.
(ii) Most researchers have been addressing the synthesis and properties of nanoparticles and, used in various kinds of biomedical and environmental applications. However, there are numerous obstacles hindering the control of the morphology, pore size, and boosting the surface area, which could be due to insignificant methods or, the improper proportion of chemical components in the synthesis. Therefore, the accurate distribution of shape and size of the materials on the surface should be facilitated via the impregnation of a suitable catalyst and a deep understanding of the mechanism of incorporating the precursor with biosurfactants.
(iii) The biosurfactant has emerged in a crucial role to modify the surface of nanoparticles in recent days and is executed in different applications. The collective information regarding this material has been applied in some biomedical applications at a very low level of research. The modified lipopeptide-mediated nanoparticles could be very innovative and influential with lower toxicity to use at the broad level of industrial applications.
(iv) It is recommended that the lipopeptide biosurfactant incorporated nanoparticles could show better activity in optical engineering, cosmetics, and textile applications, predicting collective demand for this type of biomaterials for other significant broad prospect applications.
(v) The main crucial problem of this material is the lower recovery rate and recyclization, which have shown hierarchal issues in the current generation of life in the research arena. The development of recovery and reactivation rates can be significantly beneficial in the conservation of natural resources, enhance economic security and save applied energy. The recovery and reactivation rate could be boosted by the modification of characteristics and surface properties, which is possible after applying particular additional surface-active and catalyzing agents.
Author contributions
R. K. S. prepared the manuscript; J. P. M., G. D., and P. B. revised and gave necessary inputs. G. D., P. B., and K. D. revised and gave suggestions on the manuscript. R. K. S., C. M. L., S. C. W., N. C., and J. A. S. revised the manuscript, tables, and figures.
Conflicts of interest
There are no conflicts of interest.
Acknowledgements
R. K. S. has been supported by Overseas Research Scholarships (ORS) from National Chung Cheng University (Taiwan). The authors would like to thank the Ministry of Science and Technology (Taiwan) for financial support (MOST 109-2811-M-194-502; MOST 108-2811-M-194-510).
Notes and references
- A. Saxena, R. Tripathi and R. Singh, Dig. J. Nanomater. Bios., 2010, 5, 427–432 Search PubMed.
- G. A. Płaza, J. Chojniak and I. M. Banat, Int. J. Mol. Sci., 2014, 15, 13720–13737 CrossRef PubMed.
- M. Sabet, S. Hosseini, A. Zamani, Z. Hosseini and H. Soleimani, Defect Diffus. Forum, 2016, 149–156 Search PubMed.
- A. Arshad, M. Jabbal, Y. Yan and D. Reay, J. Mol. Liq., 2019, 279, 444–484 CrossRef CAS.
- H. Kesarwani, S. Sharma and A. Mandal, ACS Omega, 2021, 6, 11327–11339 CrossRef CAS PubMed.
- D. Honecker, M. Bersweiler, S. Erokhin, D. Berkov, K. Chesnel, D. A. Venero, A. Qdemat, S. Disch, J. Jochum and A. Michels, Nanoscale Adv., 2022, 4, 1026–1059 RSC.
- Y. Song, B. Li, S. Yang, G. Ding, C. Zhang and X. Xie, Sci. Rep., 2015, 5, 1–9 Search PubMed.
- T. Rasheed, S. Shafi, M. Bilal, T. Hussain, F. Sher and K. Rizwan, J. Mol. Liq., 2020, 318, 113960 CrossRef CAS.
- L. Yin, Y. Cheng, B. Espinasse, B. P. Colman, M. Auffan, M. Wiesner, J. Rose, J. Liu and E. S. Bernhardt, Environ. Sci. Technol., 2011, 45, 2360–2367 CrossRef CAS PubMed.
- G. M. Shah, H. Ali, I. Ahmad, M. Kamran, M. Hammad, G. A. Shah, H. F. Bakhat, A. Waqar, J. Guo and R. Dong, Environ. Pollut., 2022, 293, 118559 CrossRef CAS PubMed.
- O. Bondarenko, K. Juganson, A. Ivask, K. Kasemets, M. Mortimer and A. Kahru, Arch. Toxicol., 2013, 87, 1181–1200 CrossRef CAS PubMed.
- U. Shanker, M. Rani and V. Jassal, Environ. Chem. Lett., 2017, 15, 623–642 CrossRef CAS.
- A. Khataee and M. B. Kasiri, J. Mol. Catal. A: Chem., 2010, 328, 8–26 CrossRef CAS.
- N. Dasgupta, S. Ranjan, D. Mundekkad, C. Ramalingam, R. Shanker and A. Kumar, Food Res. Int., 2015, 69, 381–400 CrossRef.
- S. Megala, A. Silambarasan, S. Kanagesan, M. Selvaraj, P. Maadeswaran, R. Ramesh, M. M. Alam and M. A. Assiri, J. Mol. Struct., 2022, 1252, 132149 CrossRef CAS.
- P. Nidheesh, M. Zhou and M. A. Oturan, Chemosphere, 2018, 197, 210–227 CrossRef CAS PubMed.
- R. K. Sharma, S.-C. Wang, J. P. Maity, P. Banerjee, G. Dey, Y.-H. Huang, J. Bundschuh, P.-G. Hsiao, T.-H. Chen and C.-Y. Chen, RSC Adv., 2021, 11, 32906–32916 RSC.
- J. Eastoe, M. J. Hollamby and L. Hudson, Adv. Colloid Interface Sci., 2006, 128, 5–15 CrossRef PubMed.
- M. Shah, D. Fawcett, S. Sharma, S. K. Tripathy and G. E. J. Poinern, Materials, 2015, 8, 7278–7308 CrossRef CAS PubMed.
- S. Taheriniya and Z. Behboodi, Int. J. Pharm. Sci. Res., 2016, 7, 4927 CAS.
- F. Md, J. Pet. Environ. Biotechnol., 2012, 3, 124 CAS.
- M. Hassanzadeh, M. Kambarani, L. Tayebi and F. Yazdian, J. Surfactants Deterg., 2012, 15, 551–556 CrossRef CAS.
- J. P. Maity, T.-J. Lin, H. P.-H. Cheng, C.-Y. Chen, A. S. Reddy, S. B. Atla, Y.-F. Chang, H.-R. Chen and C.-C. Chen, Int. J. Mol. Sci., 2011, 12, 3821–3830 CrossRef CAS PubMed.
- S. Shekhar, A. Sundaramanickam and T. Balasubramanian, Crit. Rev. Environ. Sci. Technol., 2015, 45, 1522–1554 CrossRef CAS.
- M. Healy, C. Devine and R. Murphy, Resour., Conserv. Recycl., 1996, 18, 41–57 CrossRef.
- M. M. Usman, A. Dadrasnia, K. T. Lim, A. F. Mahmud and S. Ismail, AIMS Bioeng., 2016, 3, 289–304 CAS.
- X. Li, D.-e Yin, S.-Z. Kang, J. Mu, J. Wang and G. Li, Colloids Surf., A, 2011, 384, 749–751 CrossRef CAS.
-
S. P. Goutam, G. Saxena, D. Roy, A. K. Yadav and R. N. Bharagava, Bioremediation of industrial waste for environmental safety, Springer, 2020, pp. 349–379 Search PubMed.
- A. K. Mittal, Y. Chisti and U. C. Banerjee, Biotechnol. Adv., 2013, 31, 346–356 CrossRef CAS PubMed.
- R. Parthasarathi and P. Sivakumaar, Soil Sediment Contam., 2011, 20, 892–907 CrossRef CAS.
- Y. Li, T. Su, Y. Zhang, X. Huang, J. Li and C. Li, Drug Delivery, 2015, 22, 627–637 CrossRef CAS PubMed.
- B. Bahrami-Teimoori, H. R. Pourianfar, M. Akhlaghi, A. Tanhaeian and M. Rezayi, Biotechnol. Appl. Biochem., 2019, 66, 900–910 CrossRef CAS PubMed.
- T. C. Dakal, A. Kumar, R. S. Majumdar and V. Yadav, Front. Microbiol., 2016, 1831 Search PubMed.
-
E. Pessione and R. Garcia-Contreras, Encyclopedia of Infection and Immunity, Elsevier, 2021, pp. 586–607 DOI:10.1016/B978-0-12-818731-9.00136-1.
- E. J. Gudiña, J. A. Teixeira and L. R. Rodrigues, Mar. Drugs, 2016, 14, 38 CrossRef PubMed.
- N. Kültürsay, Ö. Uygur and M. Yalaz, Turk. Arch. Pediatr./Turk. Pediatri. Ars., 2014, 49, 1 Search PubMed.
- N. Walia and S. S. Cameotra, J. Microb. Biochem. Technol., 2015, 7, 103–107 CAS.
- G. Sun, E. Sharkova, R. Chesnut, S. Birkey, M. F. Duggan, A. Sorokin, P. Pujic, S. D. Ehrlich and F. M. Hulett, J. Bacteriol., 1996, 178, 1374–1385 CrossRef CAS PubMed.
- P. Lin, H. Yuan, J. Du, K. Liu, H. Liu and T. Wang, Appl. Microbiol. Biotechnol., 2020, 104, 2319–2331 CrossRef CAS PubMed.
- J. Xie, K. Cheng, D. Zhao, G. Yang, Z. Qiao, S. Qiu, X. Yu, H. Liu, T. Li and H. Feng, Int. J. Syst. Evol. Microbiol., 2020, 70, 3406–3412 CrossRef CAS PubMed.
- X. Zheng, G. Liu, Z. Wang, J. Wang, H. Zhang and B. Liu, Curr. Microbiol., 2020, 77, 2049–2055 CrossRef CAS PubMed.
-
J. H. Jorgensen, K. C. Carroll, G. Funke, M. A. Pfaller, M. L. Landry, S. S. Richter and D. W. Warnock, Manual of Clinical Microbiology, Wiley Online Library, 11th edn, 2015, DOI:10.1128/9781555817381.
- B. Pettersson, F. Lembke, P. Hammer, E. Stackebrandt and F. G. Priest, Int. J. Syst. Evol. Microbiol., 1996, 46, 759–764 CAS.
- E. M. Berendsen, M. H. Wells-Bennik, A. O. Krawczyk, A. de Jong, A. van Heel, S. Holsappel, R. T. Eijlander and O. P. Kuipers, Genome Announc., 2016, 4, e00105–e00116 Search PubMed.
- K. Arima, A. Kakinuma and G. Tamura, Biochem. Biophys. Res. Commun., 1968, 31, 488–494 CrossRef CAS PubMed.
-
H. B. Sobrinho, J. M. Luna, R. D. Rufino, A. Porto and L. A. Sarubbo, Recent Advances in Food Biotechnology, 2013, vol. 11, pp. 1–29 Search PubMed.
- M. Aryal, M. G. Ziagova and M. Liakopoulou-Kyriakides, Water, Air, Soil Pollut., 2012, 223, 5119–5130 CrossRef CAS.
- N. Sakthipriya, G. Kumar, A. Agrawal, M. Doble and J. S. Sangwai, Energy Fuels, 2021, 35, 9883–9893 CrossRef.
- M. Bautista-Toledo, J. Méndez-Díaz, M. Sánchez-Polo, J. Rivera-Utrilla and M. Ferro-García, J. Colloid Interface Sci., 2008, 317, 11–17 CrossRef CAS PubMed.
- B. Petrie, R. Barden and B. Kasprzyk-Hordern, Water Res., 2015, 72, 3–27 CrossRef CAS PubMed.
- F.-J. Zhu, W.-L. Ma, T.-F. Xu, Y. Ding, X. Zhao, W.-L. Li, L.-Y. Liu, W.-W. Song, Y.-F. Li and Z.-F. Zhang, Ecotoxicol. Environ. Saf., 2018, 153, 84–90 CrossRef CAS PubMed.
- A. Zanoletti, S. Federici, L. Borgese, P. Bergese, M. Ferroni, L. E. Depero and E. Bontempi, J. Cleaner Prod., 2017, 141, 230–236 CrossRef CAS.
-
C. I. Sáenz-Marta, M. de Lourdes Ballinas-Casarrubias, B. E. Rivera-Chavira and G. V. Nevárez-Moorillón, Advances in Bioremediation of Wastewater and Polluted Soil, 2015, vol. 5, 94–109 Search PubMed.
- R. S. Makkar and S. S. Cameotra, J. Surfactants Deterg., 1999, 2, 367–372 CrossRef CAS.
- Y.-S. Wu, S.-C. Ngai, B.-H. Goh, K.-G. Chan, L.-H. Lee and L.-H. Chuah, Front. Pharmacol., 2017, 8, 761 CrossRef PubMed.
- P. K. Rahman and E. Gakpe, Biotechnology, 2008, 7, 360–370 CrossRef CAS.
- N. Yang, Q. Wu and Y. Xu, ACS Omega, 2020, 5, 6321–6329 CrossRef CAS PubMed.
-
M. Geissler, K. M. Heravi, M. Henkel and R. Hausmann, Biobased surfactants, Elsevier, 2019, pp. 205–240 Search PubMed.
- F. Hu, Y. Liu and S. Li, Microb. Cell Fact., 2019, 18, 1–13 CrossRef PubMed.
- P. S. Kumar and P. T. Ngueagni, J. Hazard. Mater., 2021, 407, 124827 CrossRef PubMed.
-
P. Jain, P. K. Yadav and S. Raghav, Green Sustainable Process for Chemical and Environmental Engineering and Science, Elsevier, 2021, pp. 235–254 Search PubMed.
- A. S. Reddy, C.-Y. Chen, S. C. Baker, C.-C. Chen, J.-S. Jean, C.-W. Fan, H.-R. Chen and J.-C. Wang, Mater. Lett., 2009, 63, 1227–1230 CrossRef CAS.
- A. S. Reddy, C.-Y. Chen, C.-C. Chen, J.-S. Jean, C.-W. Fan, H.-R. Chen, J.-C. Wang and V. R. Nimje, J. Nanosci. Nanotechnol., 2009, 9, 6693–6699 CrossRef CAS PubMed.
- B. R. Singh, S. Dwivedi, A. A. Al-Khedhairy and J. Musarrat, Colloids Surf., B, 2011, 85, 207–213 CrossRef CAS PubMed.
- J. P. Maity, C.-M. Hsu, T.-J. Lin, W.-C. Lee, P. Bhattacharya, J. Bundschuh and C.-Y. Chen, Environ. Nanotechnol., Monit. Manage., 2018, 9, 18–28 Search PubMed.
- F. A. Bezza, S. M. Tichapondwa and E. M. Chirwa, J. Hazard. Mater., 2020, 393, 122319 CrossRef CAS PubMed.
- R. M. Humphries, S. Pollett and G. Sakoulas, Clin. Microbiol. Rev., 2013, 26, 759–780 CrossRef CAS PubMed.
- H. Jiang, M. Xiong, Q. Bi, Y. Wang and C. Li, Acta Pharm. Sin. B, 2016, 6, 319–328 CrossRef PubMed.
- J. D. Hartgerink, E. Beniash and S. I. Stupp, Science, 2001, 294, 1684–1688 CrossRef CAS PubMed.
- N. S. Abadeer and C. J. Murphy, J. Phys. Chem. C, 2016, 120, 4691–4716 CrossRef CAS.
- J.-J. Hu, Y.-J. Cheng and X.-Z. Zhang, Nanoscale, 2018, 10, 22657–22672 RSC.
- K. Zheng, M. I. Setyawati, T.-P. Lim, D. T. Leong and J. Xie, ACS Nano, 2016, 10, 7934–7942 CrossRef CAS PubMed.
- I. Chakravarty, P. Narasimha, S. Singh, K. Kundu, P. Singh and S. Kundu, Int. J. Pharm. Sci. Res., 2018, 9, 1788–1796 CAS.
- J. Wang, J. Zhang, K. Liu, J. He, Y. Zhang, S. Chen, G. Ma, Y. Cui, L. Wang and D. Gao, Int. J. Pharm., 2020, 580, 119231 CrossRef CAS PubMed.
- L. Mocan, F. A. Tabaran, T. Mocan, T. Pop, O. Mosteanu, L. Agoston-Coldea, C. T. Matea, D. Gonciar, C. Zdrehus and C. Iancu, Int. J. Nanomed., 2017, 12, 2255 CrossRef CAS PubMed.
- Y. Ye, Z. Xia, D. Zhang, Z. Sheng, P. Zhang, H. Zhu, N. Xu and S. Liang, BioMed Res. Int., 2019, 2019, 8609218, DOI:10.1155/2019/8609218.
- D. Zhou, P. Tian, D. Li, J. Li and T. Zhang, Mater. Res. Express, 2021, 9, 095003, DOI:10.1088/2053-1591/abe24a.
- F. Peypoux, M. Guinand, G. Michel, L. Delcambe, B. C. Das and E. Lederer, Biochemistry, 1978, 17, 3992–3996 CrossRef CAS PubMed.
- R. Maget-Dana, L. Thimon, F. Peypoux and M. Ptak, Biochimie, 1992, 74, 1047–1051 CrossRef CAS PubMed.
- D. B. Tripathy, A. Mishra, J. Clark and T. Farmer, C. R. Chim, 2018, 21, 112–130 CrossRef CAS.
- K. Paraszkiewicz, P. Bernat, A. Kuśmierska, J. Chojniak and G. Płaza, J. Environ. Manage., 2018, 209, 65–70 CrossRef CAS.
- M. Kanlayavattanakul and N. Lourith, Int. J. Cosmetic Sci., 2010, 32, 1–8 CrossRef CAS PubMed.
- M. Lukic, I. Pantelic and S. Savic, Tenside, Surfactants, Deterg., 2016, 53, 7–19 CrossRef CAS.
- X. Zhao, L. Yan, X. Xu, H. Zhao, Y. Lu, Y. Wang, C. Jiang, D. Shao, J. Zhu and J. Shi, Appl. Microbiol. Biotechnol., 2019, 103, 6319–6332 CrossRef CAS PubMed.
- X. Zhao, K. Wang, C. Ai, L. Yan, C. Jiang and J. Shi, Food Packag. Shelf Life, 2021, 28, 100669 CrossRef CAS.
- L. Zhou, X. Zhao, M. Li, Y. Lu, C. Ai, C. Jiang, Y. Liu, Z. Pan and J. Shi, Appl. Microbiol. Biotechnol., 2021, 105, 3759–3770 CrossRef CAS PubMed.
- L. Zhou, X. Zhao, M. Li, L. Yan, Y. Lu, C. Jiang, Y. Liu, Z. Pan and J. Shi, Int. J. Biol. Macromol., 2021, 181, 1183–1195 CrossRef CAS PubMed.
- B. Yuan, H. Jia, W. Bu, T. Yang, L. Han-Meng, X.-Y. Ju and R.-P. Li, Int. J. Biol. Macromol., 2020, 159, 995–1003 CrossRef CAS PubMed.
- A. Ravi, V. V. T. Nandayipurath, S. Rajan, S. A. Salim, N. K. Khalid, C. T. Aravindakumar and R. E. Krishnankutty, Pest Manage. Sci., 2021, 77, 1035–1041 CrossRef CAS PubMed.
- R. B. Walton and H. B. Woodruff, J. Clin. Invest., 1949, 28, 924–926 CrossRef CAS PubMed.
- J. M. Bland, J. Org. Chem., 1996, 61, 5663–5664 CrossRef CAS.
- V. Leclère, M. Béchet, A. Adam, J.-S. Guez, B. Wathelet, M. Ongena, P. Thonart, F. Gancel, M. Chollet-Imbert and P. Jacques, Appl. Environ. Microbiol., 2005, 71, 4577–4584 CrossRef PubMed.
- M. N. Nasir and F. Besson, Biochim. Biophys. Acta, Biomembr., 2012, 1818, 1302–1308 CrossRef CAS PubMed.
- J. M. Balkovec, D. L. Hughes, P. S. Masurekar, C. A. Sable, R. E. Schwartz and S. B. Singh, Nat. Prod. Rep., 2014, 31, 15–34 RSC.
- T. Emri, L. Majoros, V. Tóth and I. Pócsi, Appl. Microbiol. Biotechnol., 2013, 97, 3267–3284 CrossRef CAS.
- H. Li, L. Wang, Y. Chai, Y. Cao and F. Lu, Nanotoxicology, 2018, 12, 1230–1240 CrossRef CAS PubMed.
- A. D. Burchardt, R. N. Carvalho, A. Valente, P. Nativo, D. Gilliland, C. P. Garcìa, R. Passarella, V. Pedroni, F. O. Rossi and T. Lettieri, Environ. Sci. Technol., 2012, 46, 11336–11344 CrossRef CAS PubMed.
- C. Chang, M. Slavin and S.-A. Chen, Arch. Toxicol., 2017, 91, 1613–1621 CrossRef CAS PubMed.
- L. Volpon, F. Besson and J.-M. Lancelin, FEBS Lett., 2000, 485, 76–80 CrossRef CAS PubMed.
- N. Vanittanakom, W. Loeffler, U. Koch and G. Jung, J. Antibiot., 1986, 39, 888–901 CrossRef CAS PubMed.
- W. Hussein, BioTechnologia, 2019, 100, 47–55 CrossRef CAS.
- V. Rangarajan, G. Dhanarajan and R. Sen, Biochem. Eng. J., 2015, 99, 147–155 CrossRef CAS.
- V. Rangarajan, G. Dhanarajan, P. Dey, D. Chattopadhya and R. Sen, Appl. Nanosci., 2018, 8, 1809–1821 CrossRef CAS.
- A. Alamer, I. Sabah, A. A. Tomah, T. Ahmed, B. Li and J. Zhang, Molecules, 2022, 27, 224 CrossRef PubMed.
- D. Biria, E. Maghsoudi, R. Roostaazad, H. Dadafarin, A. Sahebghadam Lotfi and M. Amoozegar, World J. Microbiol. Biotechnol., 2010, 26, 871–878 CrossRef CAS.
- Y. Hathout, Y.-P. Ho, V. Ryzhov, P. Demirev and C. Fenselau, J. Nat. Prod., 2000, 63, 1492–1496 CrossRef CAS PubMed.
- T. Dubois, K. Faegri, S. Perchat, C. Lemy, C. Buisson, C. Nielsen-LeRoux, M. Gohar, P. Jacques, N. Ramarao and A.-B. Kolstø, PLoS Pathog., 2012, 8, e1002629 CrossRef CAS PubMed.
- S. B. Bumpus, B. S. Evans, P. M. Thomas, I. Ntai and N. L. Kelleher, Nat. Biotechnol., 2009, 27, 951–956 CrossRef CAS PubMed.
- A. Abderrahmani, A. Tapi, F. Nateche, M. Chollet, V. Leclère, B. Wathelet, H. Hacene and P. Jacques, Appl. Microbiol. Biotechnol., 2011, 92, 571–581 CrossRef CAS PubMed.
- M. Béchet, T. Caradec, W. Hussein, A. Abderrahmani, M. Chollet, V. Leclère, T. Dubois, D. Lereclus, M. Pupin and P. Jacques, Appl. Microbiol. Biotechnol., 2012, 95, 593–600 CrossRef PubMed.
-
X. Zhao, L. Zhou, C. Ai, K. Wang, L. Yan, X. Xu, C. Jiang and J. Shi, in BIBE 2019; The Third International Conference on Biological Information and Biomedical Engineering, VDE, 2019, pp. 530–533 Search PubMed.
- J. M. Palomo and M. Filice, Nanomaterials, 2016, 6, 84 CrossRef PubMed.
- A. H. Tanzil, S. T. Sultana, S. R. Saunders, L. Shi, E. Marsili and H. Beyenal, Enzyme Microb. Technol., 2016, 95, 4–12 CrossRef CAS PubMed.
- Z. Sadowski, I. Maliszewska, B. Grochowalska, I. Polowczyk and T. Kozlecki, Mater. Sci.-Pol., 2008, 26, 419–424 CAS.
- J. Feng, X. Guo, N. Ramlawi, T. V. Pfeiffer, R. Geutjens, S. Basak, H. Nirschl, G. Biskos, H. W. Zandbergen and A. Schmidt-Ott, J. Mater. Chem. A, 2016, 4, 11222–11227 RSC.
- S. Sun, C. B. Murray, D. Weller, L. Folks and A. Moser, Science, 2000, 287, 1989–1992 CrossRef CAS PubMed.
- M. Ovais, A. Raza, S. Naz, N. U. Islam, A. T. Khalil, S. Ali, M. A. Khan and Z. K. Shinwari, Appl. Microbiol. Biotechnol., 2017, 101, 3551–3565 CAS.
- M. Ovais, A. T. Khalil, N. U. Islam, I. Ahmad, M. Ayaz, M. Saravanan, Z. K. Shinwari and S. Mukherjee, Appl. Microbiol. Biotechnol., 2018, 102, 6799–6814 CrossRef CAS PubMed.
- A. H. Nurfarahin, M. S. Mohamed and L. Y. Phang, Molecules, 2018, 23, 1049 CrossRef PubMed.
- P. Singh, Y.-J. Kim, D. Zhang and D.-C. Yang, Trends Biotechnol., 2016, 34, 588–599 CrossRef CAS PubMed.
- G. Gahlawat and A. R. Choudhury, RSC Adv., 2019, 9, 12944–12967 RSC.
- K. Simeonidis, S. Mourdikoudis, M. Moulla, I. Tsiaoussis, C. Martinez-Boubeta, M. Angelakeris, C. Dendrinou-Samara and O. Kalogirou, J. Magn. Magn. Mater., 2007, 316, e1–e4 CrossRef CAS.
- A. Albanese, P. S. Tang and W. C. Chan, Annu. Rev. Biomed. Eng., 2012, 14, 1–16 CrossRef CAS PubMed.
- A. Yadav, K. Kon, G. Kratosova, N. Duran, A. P. Ingle and M. Rai, Biotechnol. Lett., 2015, 37, 2099–2120 CrossRef CAS PubMed.
- K. B. Narayanan and N. Sakthivel, Adv. Colloid Interface Sci., 2010, 156, 1–13 CrossRef CAS PubMed.
- K. N. Thakkar, S. S. Mhatre and R. Y. Parikh, Nanomed.: Nanotechnol., Biol. Med., 2010, 6, 257–262 CrossRef CAS PubMed.
- K. Kalimuthu, R. S. Babu, D. Venkataraman, M. Bilal and S. Gurunathan, Colloids Surf., B, 2008, 65, 150–153 CrossRef CAS PubMed.
- M. G. Babu and P. Gunasekaran, Colloids Surf., B, 2009, 74, 191–195 CrossRef CAS PubMed.
- M. Ovais, A. T. Khalil, M. Ayaz, I. Ahmad, S. K. Nethi and S. Mukherjee, Int. J. Mol. Sci., 2018, 19, 4100 CrossRef PubMed.
- S. Anil Kumar, M. K. Abyaneh, S. Gosavi, S. K. Kulkarni, R. Pasricha, A. Ahmad and M. Khan, Biotechnol. Lett., 2007, 29, 439–445 CrossRef CAS PubMed.
- M. Iqtedar, M. Aslam, M. Akhyar, A. Shehzaad, R. Abdullah and A. Kaleem, Prep. Biochem., 2019, 49, 136–142 CrossRef CAS PubMed.
- C. A. Mirkin, R. L. Letsinger, R. C. Mucic and J. J. Storhoff, Nature, 1996, 382, 607–609 CrossRef CAS PubMed.
- M. P. Patil and G.-D. Kim, Colloids Surf., B, 2018, 172, 487–495 CrossRef CAS.
- G. Grasso, D. Zane and R. Dragone, Nanomaterials, 2020, 10, 11 CrossRef CAS PubMed.
- V. Gopinath and P. Velusamy, Spectrochim. Acta, Part A, 2013, 106, 170–174 CrossRef CAS PubMed.
- E. K. Elbeshehy, A. M. Elazzazy and G. Aggelis, Front. Microbiol., 2015, 6, 453 Search PubMed.
- N. Nadaf and S. Kanase, Dig. J. Nanomater. Biostruct., 2015, 10, 1189–1199 Search PubMed.
- N. Y. Nadaf and S. S. Kanase, Arabian J. Chem., 2019, 12, 4806–4814 CrossRef CAS.
- A. A. Shamsuzzaman, M. Asif, A. Mashrai and H. Khanam, Eur. Chem. Bull., 2014, 3, 939–945 Search PubMed.
- R. Khan and M. Fulekar, J. Colloid Interface Sci., 2016, 475, 184–191 CrossRef CAS PubMed.
- P. A. Sundaram, R. Augustine and M. Kannan, Biotechnol. Bioprocess Eng., 2012, 17, 835–840 CrossRef CAS.
- A. Kanakalakshmi, V. Janaki, K. Shanthi and S. Kamala-Kannan, Artif. Cells, Nanomed., Biotechnol., 2017, 45, 1304–1309 CrossRef CAS PubMed.
- M. Taran, M. Rad and M. Alavi, Pharm. Sci., 2017, 23, 198–206 CrossRef.
- A. Shivashankarappa and K. Sanjay, Nanosci. Nanotechnol. Res., 2015, 3, 6–15 CAS.
- P. Dauthal and M. Mukhopadhyay, Ind. Eng. Chem. Res., 2016, 55, 9557–9577 CrossRef CAS.
- S. Singh, M. Kowalczewska, S. Edouard, C. Eldin, C. Perreal, P. Weber, S. Azza and D. Raoult, J. Clin. Microbiol., 2013, 51, 2599–2607 CrossRef CAS PubMed.
- N. I. Hulkoti and T. Taranath, Colloids Surf., B, 2014, 121, 474–483 CrossRef CAS PubMed.
- X. Hu, C. Wang and P. Wang, Front. Microbiol., 2015, 6, 976 Search PubMed.
- P. D. Shankar, S. Shobana, I. Karuppusamy, A. Pugazhendhi, V. S. Ramkumar, S. Arvindnarayan and G. Kumar, Enzyme Microb. Technol., 2016, 95, 28–44 CrossRef CAS PubMed.
- H. Korbekandi, S. Iravani and S. Abbasi, J. Chem. Technol. Biotechnol., 2012, 87, 932–937 CrossRef CAS.
- B. Nair and T. Pradeep, Cryst. Growth Des., 2002, 2, 293–298 CrossRef CAS.
- E. Selvarajan and V. Mohanasrinivasan, Mater. Lett., 2013, 112, 180–182 CrossRef CAS.
- K. Prasad, A. K. Jha and A. Kulkarni, Nanoscale Res. Lett., 2007, 2, 248–250 CrossRef CAS.
- A. K. Jha, K. Prasad and A. Kulkarni, Colloids Surf., B, 2009, 71, 226–229 CrossRef CAS PubMed.
- K. Prasad and A. K. Jha, J. Colloid Interface Sci., 2010, 342, 68–72 CrossRef CAS PubMed.
- S. Pakrashi, S. Dalai, D. Sabat, S. Singh, N. Chandrasekaran and A. Mukherjee, Chem. Res. Toxicol., 2011, 24, 1899–1904 Search PubMed.
- Z.-Y. Yan, C.-X. Yao, D.-Y. Wan, L.-L. Wang, Q.-Q. Du, Z.-Q. Li and S.-M. Wu, Enzyme Microb. Technol., 2018, 119, 37–44 CrossRef CAS.
- P. Srivastava and M. Kowshik, Enzyme Microb. Technol., 2016, 95, 192–200 CrossRef CAS PubMed.
- D. K. F. Santos, R. D. Rufino, J. M. Luna, V. A. Santos and L. A. Sarubbo, Int. J. Mol. Sci., 2016, 17, 401 CrossRef PubMed.
- A. Potočnik and M. Oder, Lwt, 2020, 122, 109018 CrossRef.
- P. Singh and B. N. Tiwary, Bioresour. Bioprocess., 2016, 3, 1–16 CrossRef.
- P. Radha, P. Suhazsini, K. Prabhu, A. Jayakumar and R. Kandasamy, J. Surfactants Deterg., 2020, 23, 119–135 CrossRef CAS.
- T. A. Wagay, M. A. Shergujri and H. Askari, J. Dispersion Sci. Technol., 2021, 42, 813–823 CrossRef CAS.
- J. D. Desai and I. M. Banat, Microbiol. Mol. Biol. Rev., 1997, 61, 47–64 CAS.
- S. A. Novikova, G. Y. Yurkov and A. B. Yaroslavtsev, Mendeleev Commun., 2010, 20, 89–91 CrossRef CAS.
- J. Chinnam, D. K. Das, R. S. Vajjha and J. R. Satti, Int. J. Therm. Sci., 2015, 98, 68–80 CrossRef CAS.
- B. P. Binks, Curr. Opin. Colloid Interface Sci., 2002, 7, 21–41 CrossRef CAS.
- M. Behpour, S. Ghoreishi, N. Soltani and M. Salavati-Niasari, Corros. Sci., 2009, 51, 1073–1082 CrossRef CAS.
- Z. Tao, S. Zhang, W. Li and B. Hou, Corros. Sci., 2009, 51, 2588–2595 CrossRef CAS.
- I. Mnif and D. Ghribi, Pept. Sci., 2015, 104, 129–147 CrossRef CAS PubMed.
- G.-G. Ying, Environ. Int., 2006, 32, 417–431 CrossRef CAS PubMed.
- G. Seydlová and J. Svobodová, Cent. Eur. J. Med., 2008, 3, 123–133 Search PubMed.
- H. Su, F. Wang, W. Ran, W. Zhang, W. Dai, H. Wang, C. F. Anderson, Z. Wang, C. Zheng and P. Zhang, Proc. Natl. Acad. Sci. U. S. A., 2020, 117, 4518–4526 CrossRef CAS PubMed.
- F. Anjum, G. Gautam, G. Edgard and S. Negi, Bioresour. Technol., 2016, 213, 262–269 CrossRef CAS PubMed.
- G. P. Kumar and P. Rajeshwarrao, Acta Pharm. Sin. B, 2011, 1, 208–219 CrossRef.
-
M. Halecký and E. Kozliak, Advanced nano-bio technologies for water and soil treatment, Springer, 2020, pp. 495–526 Search PubMed.
- Y. Li and D. Xiang, PLoS One, 2019, 14, e0213189 CrossRef CAS PubMed.
- L. R. Rodrigues, J. Colloid Interface Sci., 2015, 449, 304–316 CrossRef CAS PubMed.
- Y. Dong, Y. Chang, Q. Wang, J. Tong and J. Zhou, Bull. Mater. Sci., 2016, 39, 35–39 CrossRef CAS.
- X. Wu, Y. Qiao, H. Yang and J. Wang, J. Colloid Interface Sci., 2010, 349, 560–564 CrossRef CAS PubMed.
- V. Yesilyurt, R. Ramireddy, M. A. Azagarsamy and S. Thayumanavan, Chem. – Eur. J., 2012, 18, 223–229 CrossRef CAS PubMed.
- M. R. Molla, P. Prasad and S. Thayumanavan, J. Am. Chem. Soc., 2015, 137, 7286–7289 CrossRef CAS PubMed.
- P.-P. Yang, X.-X. Zhao, A.-P. Xu, L. Wang and H. Wang, J. Mater. Chem. B, 2016, 4, 2662–2668 RSC.
- S. Zhang, X. Han, Y. Tan and K. Liang, Chem. Phys. Lett., 2018, 691, 135–140 CrossRef CAS.
- H. Lian, Y. Peng, J. Shi and Q. Wang, Food Hydrocolloids, 2019, 97, 105213 CrossRef CAS.
- X. Tao, S. Guo, P. Liu, X. Li and Z. Zhang, NANO, 2019, 14, 1950020 CrossRef CAS.
- Z. Abdul Karim, M. Y. Khan and A. R. A. Aziz, J. Energy Resour. Technol., 2019, 141, 102204, DOI:10.1115/1.4043553.
- W. C. Griffin, J. Soc. Cosmet. Chem., 1954, 5, 249–256 Search PubMed.
- A. P. Karlapudi, T. Venkateswarulu, J. Tammineedi, L. Kanumuri, B. K. Ravuru, V. Ramu Dirisala and V. P. Kodali, Petroleum, 2018, 4, 241–249 CrossRef.
- Y. Qin, X. Ji, J. Jing, H. Liu, H. Wu and W. Yang, Colloids Surf., A, 2010, 372, 172–176 CrossRef CAS.
- R. Jahan, A. M. Bodratti, M. Tsianou and P. Alexandridis, Adv. Colloid Interface Sci., 2020, 275, 102061 CrossRef CAS PubMed.
- W.-C. Chen, R.-S. Juang and Y.-H. Wei, Biochem. Eng. J., 2015, 103, 158–169 CrossRef CAS.
-
A. Jozala, Fermentation processes, BoD – Books on Demand, 2017 Search PubMed.
- D. Fei, F.-F. Liu, H.-Z. Gang, J.-F. Liu, S.-Z. Yang, R.-Q. Ye and B.-Z. Mu, Process Biochem., 2020, 94, 164–171 CrossRef CAS.
- F. Hu, Y. Liu, J. Lin, W. Wang and S. Li, Appl. Microbiol. Biotechnol., 2020, 104, 4017–4026 CrossRef CAS PubMed.
- I. M. Banat, Biotechnol. Lett., 1993, 15, 591–594 CrossRef CAS.
- A.-Q. She, H.-Z. Gang and B.-Z. Mu, J. Phys. Chem. B, 2012, 116, 12735–12743 CrossRef CAS PubMed.
- U. Lambrich and H. Schubert, J. Membr. Sci., 2005, 257, 76–84 CrossRef CAS.
- V. Mohanraj and Y. Chen, Trop. J. Pharm. Res., 2006, 5, 561–573 Search PubMed.
-
J. Eastoe, Surfactant Chemistry, Julian Eastoe Research Group, Bristol UK, 2003, vol. 12 Search PubMed.
- D. G. Cooper and B. G. Goldenberg, Appl. Environ. Microbiol., 1987, 53, 224–229 CrossRef CAS PubMed.
- O. Alliod, E. Almouazen, G. Nemer, H. Fessi and C. Charcosset, J. Pharm. Sci., 2019, 108, 2708–2717 CrossRef CAS PubMed.
- Q. Lin, N. Ji, M. Li, L. Dai, X. Xu, L. Xiong and Q. Sun, Food Hydrocolloids, 2020, 104, 105760 CrossRef CAS.
- O. Kaltsa, C. Michon, S. Yanniotis and I. Mandala, Ultrason. Sonochem., 2013, 20, 881–891 CrossRef CAS PubMed.
- S. K. Satpute, A. G. Banpurkar, P. K. Dhakephalkar, I. M. Banat and B. A. Chopade, Crit. Rev. Biotechnol., 2010, 30, 127–144 CrossRef CAS PubMed.
- M. Soleimani, A. Bassi and A. Margaritis, Biotechnol. Adv., 2007, 25, 570–596 CrossRef CAS PubMed.
- J. Yang, Y. Hu, D. Zhao, S. Wang, P. C. Lau and I. W. Marison, Biochem. Eng. J., 2007, 37, 212–218 CrossRef CAS.
- A. Singh, B. Singh and O. Ward, Biodegradation, 2012, 23, 865–880 CrossRef CAS PubMed.
- Y. Wen, H. Cheng, L.-J. Lu, J. Liu, Y. Feng, W. Guan, Q. Zhou and X.-F. Huang, Bioresour. Technol., 2010, 101, 8315–8322 CrossRef CAS PubMed.
- J. Liu, X.-F. Huang, L.-J. Lu, M.-X. Li, J.-C. Xu and H.-P. Deng, J. Hazard. Mater., 2011, 190, 214–221 CrossRef CAS PubMed.
- M. Pacwa-Płociniczak, G. A. Płaza, Z. Piotrowska-Seget and S. S. Cameotra, Int. J. Mol. Sci., 2011, 12, 633–654 CrossRef PubMed.
- A. A. Adewunmi, M. S. Kamal and T. I. Solling, J. Pet. Sci. Eng., 2021, 196, 107680 CrossRef CAS.
- T. A. J. de Souza, L. R. R. Souza and L. P. Franchi, Ecotoxicol. Environ. Saf., 2019, 171, 691–700 CrossRef PubMed.
- G. Zhao, S. Chandrudu, M. Skwarczynski and I. Toth, Eur. Polym. J., 2017, 93, 670–681 CrossRef CAS PubMed.
- G. Oberdörster, E. Oberdörster and J. Oberdörster, Environ. Health Perspect., 2005, 113, 823–839 CrossRef PubMed.
-
I. Arany and R. L. Safirstein, Seminars in nephrology, Elsevier, 2003, pp. 460–464 Search PubMed.
- L. Gianni, E. H. Herman, S. E. Lipshultz, G. Minotti, N. Sarvazyan and D. B. Sawyer, J. Clin. Oncol., 2008, 26, 3777 CrossRef PubMed.
- S. Amptoulach and N. Tsavaris, Chemother. Res. Pract., 2011, 2011, 843019, DOI:10.1155/2011/843019.
- C. Hazra, D. Kundu, A. Chatterjee, A. Chaudhari and S. Mishra, Colloids Surf., A, 2014, 449, 96–113 CrossRef CAS.
- Y. Zhu and L. Liao, J. Nanosci. Nanotechnol., 2015, 15, 4753–4773 CrossRef CAS PubMed.
- A. K. Zhanataev, E. A. Anisina, A. V. Kulakova, I. P. Shilovskiy, A. A. Lisitsyn, O. O. Koloskova, M. R. Khaitov and A. D. Durnev, Toxicol. Lett., 2020, 328, 1–6 CrossRef CAS PubMed.
- H. B. Ayed, S. Bardaa, D. Moalla, M. Jridi, H. Maalej, Z. Sahnoun, T. Rebai, P. Jacques, M. Nasri and N. Hmidet, Process Biochem., 2015, 50, 1023–1030 CrossRef.
- N. S. Shaligram and R. S. Singhal, Food Technol. Biotechnol., 2010, 48, 119–134 CAS.
- M. Ohadi, H. Forootanfar, G. Dehghannoudeh, T. Eslaminejad, A. Ameri, M. Shakibaie and A. Najafi, Bionanoscience, 2020, 10, 899–908 CrossRef.
- M. Moryl, M. Spętana, K. Dziubek, K. Paraszkiewicz, S. Różalska, G. A. Płaza and A. Różalski, Acta Biochim. Pol., 2015, 62, 725–732 CrossRef CAS PubMed.
- A. Johnson, F. Kong, S. Miao, S. Thomas, S. Ansar and Z.-L. Kong, Nanomaterials, 2021, 11, 356 CrossRef CAS PubMed.
- V. S. V. Santos, E. Silveira and B. B. Pereira, J. Toxicol. Environ. Health, Part B, 2018, 21, 382–399 CAS.
- L. Wang, C. Hu and L. Shao, Int. J. Nanomed., 2017, 12, 1227 CrossRef CAS PubMed.
- P. P. Mahamuni-Badiger, P. M. Patil, M. V. Badiger, P. R. Patel, B. S. Thorat-Gadgil, A. Pandit and R. A. Bohara, Mater. Sci. Eng., C, 2020, 108, 110319 CrossRef CAS PubMed.
- E. Sánchez-López, D. Gomes, G. Esteruelas, L. Bonilla, A. L. Lopez-Machado, R. Galindo, A. Cano, M. Espina, M. Ettcheto and A. Camins, Nanomaterials, 2020, 10, 292 CrossRef PubMed.
- K. Srinivasan, S. Muruganandan, J. Lal, S. Chandra, S. Tandan and V. R. Prakash, J. Ethnopharmacol., 2001, 78, 151–157 CrossRef CAS PubMed.
- A. G. Guimarães, M. A. Xavier, M. T. de Santana, E. A. Camargo, C. A. Santos, F. A. Brito, E. O. Barreto, S. C. Cavalcanti, A. R. Antoniolli and R. Oliveira, Naunyn-Schmiedeberg's Arch. Pharmacol., 2012, 385, 253–263 CrossRef PubMed.
- M. da Silva Lima, L. J. Quintans-Júnior, W. A. de Santana, C. M. Kaneto, M. B. P. Soares and C. F. Villarreal, Eur. J. Pharmacol., 2013, 699, 112–117 CrossRef PubMed.
- L. C. Almekinders, Sports Med., 1999, 28, 383–388 CrossRef CAS PubMed.
- R. Medzhitov, Nature, 2008, 454, 428–435 CrossRef CAS PubMed.
- J. S. Tan and T. M. File Jr, Med. Clin. North Am., 1995, 79, 679–693 CrossRef CAS PubMed.
- H. Giamarellou and A. Antoniadou, Med. Clin. North Am., 2001, 85, 19–42 CrossRef CAS PubMed.
- N. Krishnan, B. Velramar, R. Pandiyan and R. K. Velu, Artif. Cells, Nanomed., Biotechnol., 2018, 46, 488–499 CrossRef CAS PubMed.
- N. C. Silva, S. Silva, B. Sarmento and M. Pintado, Drug Delivery, 2015, 22, 885–893 CrossRef CAS PubMed.
-
B. Ghosh and T. K. Giri, Polysaccharide-Based Nano-Biocarrier Drug Delivery, 2018, p. 63 Search PubMed.
- C. Tong, L. Li, F. Xiao, J. Fan, X. Zhong, X. Liu, B. Liu, Z. Wu and J. Zhou, Biomater. Sci., 2019, 7, 5097–5111 RSC.
- M. I. González-Sánchez, S. Perni, G. Tommasi, N. G. Morris, K. Hawkins, E. López-Cabarcos and P. Prokopovich, Mater. Sci. Eng., C, 2015, 50, 332–340 CrossRef PubMed.
- S. A. Algharib, A. Dawood and S. Xie, Drug Delivery, 2020, 27, 292–308 CrossRef CAS PubMed.
- D. G. Meeker, T. Wang, W. N. Harrington, V. P. Zharov, S. A. Johnson, S. V. Jenkins, S. E. Oyibo, C. M. Walker, W. B. Mills and M. E. Shirtliff, Int. J. Hyperthermia, 2018, 34, 209–219 CrossRef CAS PubMed.
- D. He, T. Yang, W. Qian, C. Qi, L. Mao, X. Yu, H. Zhu, G. Luo and J. Deng, Appl. Mater. Today, 2018, 12, 415–429 CrossRef.
- M. A. Matica, F. L. Aachmann, A. Tøndervik, H. Sletta and V. Ostafe, Int. J. Mol. Sci., 2019, 20, 5889 CrossRef CAS PubMed.
- D. Kundu, C. Hazra, A. Chatterjee, A. Chaudhari, S. Mishra, A. Kharat and K. Kharat, RSC Adv., 2016, 6, 80438–80454 RSC.
- N. Jemil, H. Ben Ayed, A. Manresa, M. Nasri and N. Hmidet, BMC Microbiol., 2017, 17, 1–11 CrossRef PubMed.
- E. Yalcin and K. Cavusoglu, Turk. J. Biochem., 2010, 35, 243–247 CAS.
- I. O. Skorochod, A. O. Roy and I. K. Kurdish, Nanoscale Res. Lett., 2016, 11, 1–6 CrossRef CAS PubMed.
- L. Azeez, A. Lateef and S. A. Adebisi, Appl. Nanosci., 2017, 7, 59–66 CrossRef CAS.
- O. Greguška, Rheumatologia, 1997, 11, 161–166 Search PubMed.
- Z. Li, H. Jiang, C. Xu and L. Gu, Food Hydrocolloids, 2015, 43, 153–164 CrossRef CAS.
- R. A. Apak, M. Özyürek, K. Güçlü and E. Çapanoğlu, J. Agric. Food Chem., 2016, 64, 997–1027 CrossRef CAS PubMed.
- W. Huang, Y. Lang, A. Hakeem, Y. Lei, L. Gan and X. Yang, Int. J. Nanomed., 2018, 13, 1723 CrossRef CAS PubMed.
- S. Sungsuwan, Z. Yin and X. Huang, ACS Appl. Mater. Interfaces, 2015, 7, 17535–17544 CrossRef CAS PubMed.
- Y. Dong, K. T. Love, J. R. Dorkin, S. Sirirungruang, Y. Zhang, D. Chen, R. L. Bogorad, H. Yin, Y. Chen and A. J. Vegas, Proc. Natl. Acad. Sci. U. S. A., 2014, 111, 3955–3960 CAS.
- E. Mhatre, A. Troszok, R. Gallegos-Monterrosa, S. Lindstädt, T. Hölscher, O. P. Kuipers and Á. T. Kovács, Microbiology, 2016, 162, 1468–1478 CrossRef CAS PubMed.
- S. Stoeckel, S. Meisel, R. Boehme, M. Elschner, P. Roesch and J. Popp, J. Raman Spectrosc., 2009, 40, 1469–1477 CrossRef CAS.
- Z. V. Feng, B. R. Miller, T. G. Linn, T. Pho, K. N. L. Hoang, M. N. Hang, S. L. Mitchell, R. T. Hernandez, E. E. Carlson and R. J. Hamers, Environ. Sci.: Nano, 2019, 6, 305–314 RSC.
-
Z. H. Bhuiyan, C. F. Anderson and H. Cui, Self-assembling Biomaterials, Elsevier, 2018, pp. 533–561 Search PubMed.
-
M. Khan, M. A. Hanif, M. A. Ayub, M. I. Jilani and S. A. S. Chatha, Medicinal Plants of South Asia, Elsevier, 2020, pp. 587–600 Search PubMed.
-
S. Smith, The Boston Globe, 2010, pp. 45–49.
- E. Bhaskar, Lancet Infect. Dis., 2010, 10, 749 CrossRef PubMed.
-
S. Yuasa, The Boston Globe, Associated Press, (8 September 2010), 2010.
- D. M. Ghaith, Z. K. Mohamed, M. G. Farahat, W. A. Shahin and H. O. Mohamed, Arab J. Gastroenterol., 2019, 20, 19–22 CrossRef PubMed.
- C. M. Hudson, Z. W. Bent, R. J. Meagher and K. P. Williams, PLoS One, 2014, 9, e99209 CrossRef PubMed.
- G. Kumar N, G. Kumar, S. Mallick, S. K. Ghosh, P. Pramanick and A. S. Ghosh, FEMS Microbiol. Lett., 2019, 366, fnz118 Search PubMed.
- F.-L. Fabian, Biosci., Biotechnol. Res. Asia, 2019, 16, 693–695 Search PubMed.
- D. Divakaran, A. Chandran and R. Pratap Chandran, Braz. J. Microbiol., 2011, 42, 1397–1404 CrossRef CAS PubMed.
-
A. M. Aguilar, B. M. Soares, J. N. Pelin, B. B. Gerbelli and W. A. Alves, Peptide-based Biomaterials, 2020, pp. 126–173 Search PubMed.
- C. Gautier and T. Bürgi, ChemPhysChem, 2009, 10, 483–492 CrossRef CAS PubMed.
- E. Gross, J. H. Liu, S. Alayoglu, M. A. Marcus, S. C. Fakra, F. D. Toste and G. A. Somorjai, J. Am. Chem. Soc., 2013, 135, 3881–3886 CrossRef CAS PubMed.
- B. B. Gerbelli, S. V. Vassiliades, J. E. Rojas, J. N. Pelin, R. S. Mancini, W. S. Pereira, A. M. Aguilar, M. Venanzi, F. Cavalieri and F. Giuntini, Macromol. Chem. Phys., 2019, 220, 1900085 CrossRef.
- M. Behera and G. Giri, Mater. Sci.-Pol., 2014, 32, 702–708 CrossRef CAS.
- S. Rajaboopathi and S. Thambidurai, Mater. Chem. Phys., 2019, 223, 512–522 CrossRef CAS.
- A. P. Devi, D. K. Padhi, P. M. Mishra and A. K. Behera, J. Ind. Eng. Chem., 2022, 118–129 CrossRef CAS.
- A. S. Reddy, Y.-H. Kuo, S. B. Atla, C.-Y. Chen, C.-C. Chen, R.-C. Shih, Y.-F. Chang, J. P. Maity and H.-J. Chen, J. Nanosci. Nanotechnol., 2011, 11, 5034–5041 CrossRef CAS PubMed.
- K. S. Rao, K. El-Hami, T. Kodaki, K. Matsushige and K. Makino, J. Colloid Interface Sci., 2005, 289, 125–131 CAS.
- H. Amani, J. Surfactants Deterg., 2017, 20, 589–597 CrossRef CAS.
- S. Sabir, Crit. Rev. Environ. Sci. Technol., 2015, 45, 1916–1945 CrossRef CAS.
- M. Cushen, J. Kerry, M. Morris, M. Cruz-Romero and E. Cummins, Trends Food Sci. Technol., 2012, 24, 30–46 CAS.
|
This journal is © The Royal Society of Chemistry 2023 |