DOI:
10.1039/D3SD00044C
(Paper)
Sens. Diagn., 2023,
2, 1236-1248
Effect of doping mediated oxygen vacancies on the charge transfer ability of zinc oxide nanosheets for electrochemical glucose sensing†
Received
20th February 2023
, Accepted 30th June 2023
First published on 3rd July 2023
Abstract
We reported on hydrothermally synthesized zinc oxide (ZnO) nanosheets modified by doping with silver (Ag: 1, 2, 3, 4, and 5%) to improve their electrochemical properties for glucose sensing with and without an enzyme. SEM, XRD, EDX, and FTIR were used to investigate the microstructural, chemical, and optical properties of pristine ZnO and Ag-doped ZnO. XPS confirms that silver acts as an effective oxygen vacancy suppressor. Cyclic voltammetry studies revealed that the 5% Ag-doped ZnO electrode has a higher anodic current than the pristine ZnO and Ag (1, 2, 3, 4%)-doped ZnO electrodes. Because of the higher anodic current, the 5% Ag-doped ZnO electrode was used for sensing glucose with and without an enzyme. The 5% Ag-doped ZnO nanosheet-based electrode without an enzyme exhibited enhanced sensitivity (∼104.7 μA mM−1 cm−2), lower detection limits (∼0.06 mM), higher selectivity, practical repeatability & reproducibility, and good stability compared to the 5% Ag-doped ZnO nanosheet-based electrode with an enzyme (sensitivity ∼98.3 μA mM−1 cm−2 and LOD ∼0.098 mM), with each having a response time of ∼5 s.
1. Introduction
Increased sugar consumption in the diet has been linked to a number of serious illnesses, such as cardiovascular diseases (e.g.: stroke or heart attack, heart failure), sleep apnea, type 2 diabetes, metabolic syndrome, and obesity.1,2 Diabetes is a metabolic condition that results in an elevated blood sugar level, which triggers multiple metabolic pathways linked to inflammation and apoptosis.3 There is currently no cure for this disease, so diabetic individuals must constantly monitor their blood glucose level in order to avoid health problems. As a result, a biosensor that can detect glucose quickly, accurately, and consistently is required.4 In this regard, surface plasmon resonance (SPR), fluorescence, colorimetric, and surface enhanced Raman spectroscopy (SERS) technique-based sensors have gained appreciable attention for glucose detection.5 For example, Hu et al.5 recently reported a cost-effective cadmium telluride (CdTe) quantum dot (QD) based visual sensor for glucose detection (limit of detection (LOD) ∼ 5 nm) based on a colorimetric technique. A nanoceria-based glucose sensor was reported by Liu et al.6 based on a fluorescence technique. The sensor exhibits a lower LOD of ∼8.9 μM in buffer. He et al.7 reported a sensitive optical sensor for glucose monitoring using Ag/Au bimetallic nanoshells, which detected 4.4 ± 0.4 × 10−3 M glucose in a serum sample. Interestingly, there has been a significant shift in glucose detection using electrochemical techniques, which are efficient and flexible methods for enabling real-time and on-site measurement in different fields. So, in recent times, electrochemical glucose sensors have attracted lots of attention due to their low cost, simple fabrication technique, high accuracy, selectivity and sensitivity.8 It is well known that the typical blood glucose concentration range in the human body is 110 ± 25 mg dl−1 (approximately 4.7–7.5 mM). However, diabetes patients have substantially higher blood glucose concentrations (>20 mM).9 Most glucose biosensors need to use enzymes to improve sensitivity and selectivity when measuring blood glucose levels. The literature suggests that glucose oxidase (GOx) is a promising enzyme for the detection of glucose due to its higher selectivity towards glucose.10–12 However, the activity of enzyme immobilized glucose sensors has limitations, like poor long-term stability and reproducibility. Further, GOx is vulnerable to a variety of experimental conditions such as humidity, temperature, pH, ionic detergents, and hazardous compounds. Consequently, a non-enzymatic glucose biosensor that is highly sensitive and selective and can be made at a low cost is required.13 Many transition metal oxides including CuO, NiO, ZnO, Co3O4, and MnO2 have recently made significant contributions to non-enzymatic sensors.14,15 Among these, ZnO has several unique features such as a wide band gap, excellent chemical characteristics, superior biocompatibility,16 high isoelectric point (IEP),17 non-toxicity,18 fast electron transfer capability,16,19etc., along with optical,17,20 electrical21 and piezoelectric22 and pyroelectric23 properties. In spite of all these properties, the sensing properties of pristine ZnO are found to be inadequate. Earlier studies state that modifying ZnO by either doping or functionalizing catalysts, such as noble metals, transition metals, carbon nanotubes, graphene, etc., with unique nanostructures could alleviate this problem and thus improve biosensor performances.24–31 For example, Raza et al. reported a non-enzymatic glucose sensor screen printed electrode based on Fe-doped zinc oxide (Fe@ZnO) nanoparticles synthesized by a simple stirring method, which showed a good LOD of 0.30 μM.25 Luo et al. described the development of a high-performance (sensitivity of 7.184 MHz mM−1) Mn-doped ZnO multilayer structure Love mode surface acoustic wave (SAW) biosensor for continuous glucose monitoring.26 Shukla et al. also synthesized Mn-doped ZnO nanopencils for an enzymatic glucose biosensor and found a 17-fold increase in sensitivity for Mn-doped ZnO in comparison to pristine ZnO.27 Ghosh et al. reported an Al-doped ZnO thin film for label-free glucose detection based on fluorescence quenching which has a very high sensitivity with a LOD of 20 μM.28 Vijayaprasath et al. studied the glucose-sensing behavior of Co-doped ZnO nanoparticles made by co-precipitation and found that the constructed biosensor is extremely selective towards glucose.29 In addition, Mahmoud et al. developed an impedimetric non-enzymatic sensor based on Cu-doped ZnO nanoparticles, which exhibited a greater sensitivity with a good LOD in comparison to pristine ZnO.30 Peng et al.31 reported a non-enzymatic glucose sensor based on Ga-doped ZnO nanorods, which exhibited a sensitivity of 33.4 μA mM−1 cm−2 with good stability and excellent anti-interference ability. Chakraborty et al.32 developed C-doped ZnO nanorod arrays for non-enzymatic glucose sensing which showed a sensitivity of 13.66 μA mM−1 cm−2 with a linear range of 0.7–14 mM. The literature suggests that there are several reports available regarding transition metal doped ZnO for applications in glucose sensing, but few are reported for noble metals. Ag is a noble metal with strong electrical conductivity and chemical stability.33 Ag-doped ZnO can be easily synthesized under simple circumstances and has already been used to prevent the recombination of photo-induced electron–hole pairs in photo-degradation reactors and dye-sensitized solar cells.34,35 Therefore, doping ZnO with Ag has the potential to boost the transfer of redox electrons from ZnO to the electrode of a glucose sensor.36 A Ag-doped ZnO nanorod-based enzymatic glucose sensor has been reported by Fan Zhou et al.,36 which showed a sensitivity of 3.85 μA mM−1 cm−2 with a detection limit of 1.5 μM. Further, newly developed morphologies (e.g., nanorings, nanocombs, nanoflakes, nanorods, nanobelts, nanoworms, thin films, etc.) are beneficial for sensing applications because of their larger specific surface areas and surface activities.37–46 To date, great initiatives have been taken to create an exceptional ZnO nanostructure that maximizes the number of exposed sites. There are several techniques, such as chemical vapour deposition, spray pyrolysis, sol–gel methods, hydrothermal methods, etc., available for synthesizing such ZnO nanostructures.47–49 Among them, the hydrothermal process has attracted many researchers due to its specific characteristics, such as simple equipment, environment friendliness, moderate preparation conditions, and low cost.50 Here, we aimed to fabricate ZnO with a unique nanostructure through a hydrothermal method and to improve its properties with Ag doping for electrochemical glucose sensing. Besides, several standard characterization techniques have been performed to show and determine the microstructural, surface, and electrochemical properties of the prepared pristine and Ag-doped ZnO matrices. Our findings manifest that (i) the hydrothermally synthesized pristine ZnO has a nanosheet-like structure and with an increase in Ag doping concentration, the size of the prepared ZnO nanosheets decreases; (ii) the charge transfer characteristics have been enhanced for Ag-doped ZnO samples, and (iii) a non-enzymatic electrode based on the Ag-doped ZnO nanosheets has good glucose sensing performance (sensitivity ∼104.7 μA mM−1 cm−2) compared to a prepared enzymatic electrode (sensitivity ∼98.3 μA mM−1 cm−2).
2. Experimental
2.1 Materials and methods
Zinc nitrate hexahydrate (Zn(NO3)2·6H2O) (Merck), glucose oxidase (GOx) (Aspergillus niger RM7064-10000U), phosphate buffered saline (PBS) (0.01 M, pH 7.4) (HIMEDIA), potassium ferrocyanide trihydrate (K4[Fe(CN)6]·3H2O) (Merck), potassium ferricyanide (K3[Fe(CN)6]) (Merck), sodium hydroxide pellets (NaOH) (Merck), silver nitrate (AgNO3) (Merck), potassium bromide (KBr) (Merck), uric acid (C5H4N4O3) (Loba Chemie), ascorbic acid (C6H8O6) (Merck), cholesterol (C27H46O) (SRL) and methanol (CH3OH) (Merck) were purchased from Zenith India. Anhydrous D-(+)-glucose (C6H12O6) (Merck) was purchased from North-East Chemical Corporation, India.
The surface morphological and structural properties of the pristine and Ag-doped ZnO samples were investigated using a field emission scanning electron microscope (FESEM, Ultra plus Carl Zeiss) and an X-ray diffractometer (XRD, D8 Advance Eco-Bruker), respectively. Furthermore, energy dispersive X-ray spectroscopy (EDX, Oxford Instruments), attached to the FESEM, was used to determine the elemental composition of the samples. X-ray photoelectron spectroscopy (XPS, PHI Versa Probe III electron spectrometer) was used to determine the chemical states of the pristine and Ag-doped ZnO samples. The Fourier transform infrared (FTIR) spectra of the samples were recorded using an FTIR spectrometer (Thermo Scientific Nicolet iS5) with KBr pellets.
The electrochemical characteristics of the prepared electrodes were studied and glucose-sensing measurements were performed by using an electrochemical workstation (Gamry Reference 3000) at room temperature with a three-electrode system in 0.01 M PBS (pH 7.4) solution containing 5 mM [Fe(CN)6]3−/4−. In the electrochemical workstation, the prepared electrodes, a Pt wire, and Ag/AgCl with a saturated KCl solution were used as a working electrode, a counter electrode, and a reference electrode, respectively. The electron transfer properties between the electrode surface and electrolyte were investigated with electrochemical impedance spectroscopy (EIS) using an alternating voltage of 10 mV with a frequency ranging from 0.2 Hz to 100 kHz. Cyclic voltammetry was used to explore the electrochemical characteristics of the prepared electrodes, along with quantifying the glucose concentration in the sample solution. The anti-interference ability and effect of temperature on the prepared electrodes were studied using square wave voltammetry (SWV).
2.2 Fabrication of electrodes
In this work, pristine ZnO, 1% Ag-doped ZnO (ZnO:Ag1), 2% Ag-doped ZnO (ZnO:Ag2), 3% Ag-doped ZnO (ZnO:Ag3), 4% Ag-doped ZnO (ZnO:Ag4) and 5% Ag-doped ZnO (ZnO:Ag5) were synthesized using a hydrothermal method (ESI,† section S1.1). As shown in Fig. 1, for the application of nonenzymatic and enzymatic glucose sensors, pristine and Ag-doped ZnO nanosheet based sensing electrodes were fabricated on ITO (indium tin oxide) coated glass substrates without and with glucose oxidase (GOx) enzyme immobilization on the surface of the prepared nanosheets, respectively (ESI,† sections S1.2 and S1.3).
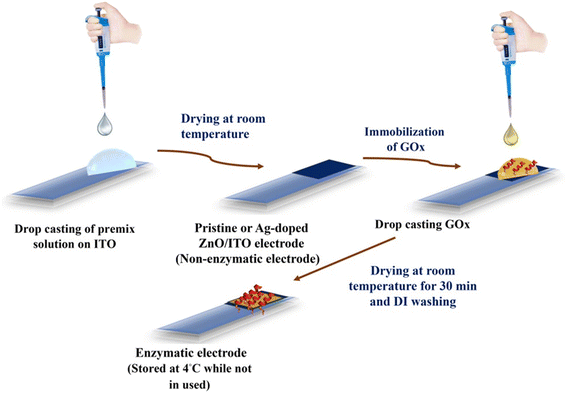 |
| Fig. 1 Sequential schematic diagram to fabricate enzymatic and non-enzymatic electrodes for glucose sensing. | |
3. Material characterization and analysis
In this work, field emission scanning electron microscopy (FESEM), X-ray diffraction (XRD), X-ray photoelectron spectroscopy (XPS), energy dispersive X-ray spectroscopy (EDX), and Fourier transform infrared (FTIR) spectroscopy were used to investigate the microstructural, chemical, and surface properties of pristine ZnO and Ag-doped ZnO nanosheets. As shown in Fig. 2(a–f), the FESEM images display the growth of nanosheet-like structures for all the prepared pristine ZnO and Ag-doped ZnO samples (ESI,† section S2.1).
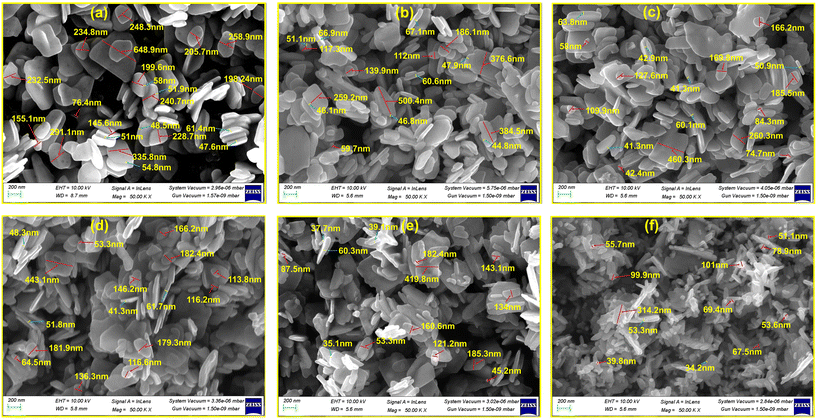 |
| Fig. 2 FESEM micrographs of the pristine-ZnO (a), ZnO:Ag1 (b), ZnO:Ag2 (c), ZnO:Ag3 (d), ZnO:Ag4 (e), and ZnO:Ag5 (f) nanosheets. | |
The crystal structure of the pristine and Ag-doped ZnO nanosheets was studied by the X-ray diffraction (XRD) technique. XRD patterns of the pristine and Ag-doped ZnO samples are displayed in Fig. 3. The X-ray diffractogram of pristine ZnO demonstrated multiple diffraction peaks at 2θ ∼ 31.74°, 34.41°, 36.24°, 47.53°, 56.56°, and 62.83° corresponding to the crystallographic planes (100), (002), (101), (102), (110), and (103), respectively, signifying the formation of a hexagonal wurtzite structure.51 Also, the XRD diffractogram of Ag-doped ZnO nanosheets revealed additional diffraction peaks of the (111), (200), and (220) planes (marked with “*” in Fig. 3), matching with the face-centered-cubic (fcc) phase of metallic Ag,52 which indicates the formation of crystalline silver clusters in the Ag-doped ZnO.53 In addition, a small shift in XRD peak position towards a higher angle for ZnO:Ag1, ZnO:Ag2, and ZnO:Ag5 was observed compared to that for ZnO:Ag3 and ZnO:Ag4. The observed shift in XRD peak position towards a higher angle for ZnO:Ag1, ZnO:Ag2, and ZnO:Ag5 suggests that Ag ions occupied the interstitial sites of ZnO.54 However, the absence of such a shift in XRD peaks indicates the segregation of Ag particles over the grain boundaries of ZnO or incorporation of only a modest quantity of Ag ions in the substitutional Zn2+ sites. Due to the difference in ionic radii between Zn2+ and Ag+, the Ag particles preferentially segregate near the ZnO grain boundaries, making the latter implausible.54,55
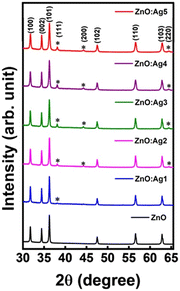 |
| Fig. 3 The XRD diffractograms of pristine and (1–5%) Ag-doped ZnO nanosheets. | |
Further, the XPS survey scans of the pristine ZnO and ZnO:Ag5 nanosheets are displayed in Fig. S2 (ESI†). The survey scan of pristine ZnO revealed characteristic peaks corresponding to C1s, Zn2p and O1s, which indicates the formation of ZnO. The survey scan of ZnO:Ag5 (Fig. S2†) demonstrated characteristic peaks corresponding to C1s, Zn2p, O1s, and Ag3d, indicating the successful incorporation of Ag ions in ZnO:Ag5. The presence of carbon in the samples is most likely due to the carbon adsorption process under ambient conditions. Here, the measured XPS data were calibrated with respect to the adventitious carbon C1s peak (284.8 eV).56Fig. 4(a) shows the high-resolution XPS (HRXPS) spectra of Zn2p for pristine ZnO and ZnO:Ag5 nanosheets, where the Zn2p peak splits into Zn2p3/2 and Zn2p1/2 due to spin–orbit interaction with a doublet peak energy separation of around 23.0 eV,57 confirming the Zn2+ state in the ZnO lattice.58 For pristine ZnO, the split Zn2p peaks were found at ∼1021.15 eV (Zn2p3/2) and ∼1044.15 eV (Zn2p1/2). These values vary from those of stoichiometric ZnO (1022.1 eV for Zn2p3/2 and 1045.1 eV for Zn2p1/2), which can be ascribed to the charge transfer variation from Zn2+ to O2− due to the presence of vacancies.59 However, for the ZnO:Ag5 sample, the Zn2p3/2 (∼1020.92 eV) and Zn2p1/2 (∼1043.92 eV) peaks were found to shift slightly towards a lower binding energy, suggesting the presence of oxygen vacancies.59Fig. 4(b) and (c) show the HRXPS spectra for the O1s core level of pristine ZnO and ZnO:Ag5 nanosheets, respectively. The deconvoluted peaks for pristine ZnO (Fig. 4(b)) were located at binding energies of ∼529.97 eV, ∼531.24 eV, and ∼531.90 eV, corresponding to O(I), O(II), and O(III), respectively. The lower binding energy peak O(I) centered at ∼529.97 eV is due to lattice oxygen (OL), which contributes to the ZnO lattice's perfect hexagonal wurtzite structure.60,61 The middle peak O(II) at a binding energy position of ∼531.24 eV is attributed to the presence of oxygen vacancies (Vos) in the ZnO lattice.61,62 The higher binding energy peak O(III) centered at ∼531.90 eV is ascribed to chemisorbed oxygen species, such as OH− and –CO3, and adsorbed H2O.63 In addition, the O1s spectrum is also shifted towards lower binding energy for ZnO:Ag5 (Fig. 4(c)), which again confirms the presence of oxygen vacancies caused by Ag doping.49 In order to assess the relative amounts of oxygen vacancies, the ratios of the respective O(II)/[O(I) + O(II)] sub-peak areas are determined, as listed in Table S1 (ESI†). Because of the strong binding energies between silver and oxygen, the ratio of O(II)/[O(I) + O(II)] drops for ZnO:Ag5, indicating that silver acts as an effective oxygen vacancy suppressor,46 which is also corroborated by measured EDX results (ESI,† section S2.1). As can be seen from the HRXPS spectrum for Ag3d of ZnO:Ag5 (Fig. 4(d)), the difference between the peaks of Ag3d5/2 (368.81 eV) and Ag3d3/2 (374.81 eV) is 6.0 eV, confirming the presence of the purely metallic Ag state in ZnO:Ag5.64–67 Moreover, the FTIR spectra (Fig. S3†) confirmed the formation of pristine ZnO and the successful incorporation of Ag ions into the ZnO host matrix, as well as the successful immobilization of GOx on the surface of the pristine and Ag-doped ZnO nanosheets (ESI,† section S2.1).
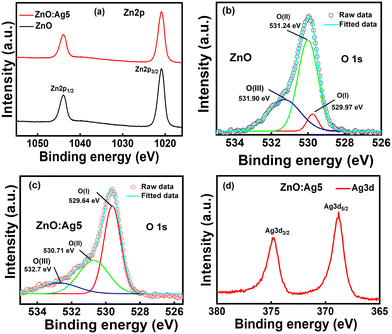 |
| Fig. 4 HRXPS spectra of Zn2p (a), O1s (b and c), and Ag3d (d) for the pristine-ZnO and ZnO:Ag5 nanosheets. | |
4. Electrochemical analysis of electrodes
Electrochemical impedance spectroscopy (EIS) was performed to study the electron transfer properties between the electrode surface and electrolyte. Fig. 5(a) shows the Nyquist plots for the pristine ZnO and Ag-doped ZnO electrodes prepared on ITO substrates. The resulting data were then fitted to an equivalent electrical circuit (EEC) that provides quantitative information regarding the processes happening at the electrode surface. Here, the observed data were fitted to a Randles equivalent circuit model as shown in Fig. 5(a)(inset), where Rs, Cdl, Rct, and Zw correspond to the solution (or ionic) resistance of the electrolyte, double layer capacitance, charge-transfer resistance, and Warburg resistance, respectively. As listed in Table S2 (ESI†), the decreasing value of Rct revealed the enhancement of electron transfer between the solution and the electrodes, suggesting that doping may enhance the charge transfer ability of ZnO.65
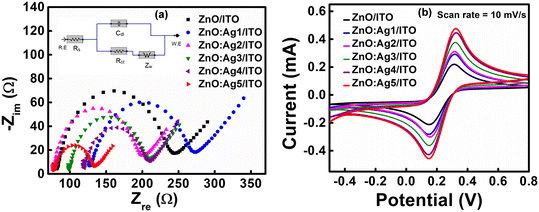 |
| Fig. 5 (a) Nyquist plots for the pristine and Ag-doped ZnO/ITO electrodes, and Randles circuit (corresponding inset). (b) Cyclic voltammograms (CVs) obtained for pristine and Ag-doped ZnO/ITO electrodes at a scan rate of 10 mV s−1. | |
Further, as presented in Fig. 5(b), cyclic voltammograms (CVs) of the pristine and Ag-doped ZnO electrodes prepared on ITO substrates were recorded at a scan rate (ν) of 10 mV s−1. The CVs revealed that the anodic (oxidation) and cathodic (reduction) peak currents of the electrodes are at ∼0.32 V and ∼0.14 V, respectively. Also, it was observed that the magnitude of the anodic and cathodic peak currents increases as the concentration of the Ag dopant increases in the ZnO host matrix, reaching a maximum value of ∼0.541 mA and ∼0.492 mA, respectively, for the ZnO:Ag5/ITO electrode. Here, the introduction of defect states by Ag doping in the ZnO host matrix (EDX & XPS results) and the improved surface area (SEM results) are the two main factors responsible for the rapid charge transfer rate between the electrode and the electrolyte, resulting in an enhancement in the electrochemical response of the electrodes.66 Next, CV measurements were also performed at different scan rates (ν = 10, 20, 40, 60, 80, and 100 mV s−1) to investigate the kinetics and transfer characteristics of the prepared pristine and Ag-doped ZnO electrodes (ESI,† Fig. S4(a–f)). From Fig. S4(a–f),† it was observed that the anodic peak current (Ipa) and cathodic peak current (Ipc) are shifted towards positive and negative potentials, respectively, with increasing scan rate. Also, the ratio of peak currents (i.e., anodic to cathodic) for all the electrodes (Table S3†) was found to be larger than unity. These results suggest that the pristine and Ag-doped ZnO electrodes have a quasi-reversible nature.67,68 As shown in Fig. S5(a–f), it was observed that Ipa and Ipc for all the electrodes increase linearly with the scan rate and the square root of the scan rate, respectively, indicating that the electrodes undergo a combination of surface and diffusion controlled electrochemical processes.69 The diffusion coefficient (D) of redox species from the electrolyte to electrodes and the surface concentration of electroactive sites (I*) were calculated using the Randles–Sevcik equation, Ipa = (2.69 × 105)AeD1/2n3/2ν1/2C, and Brown Anson model, Ipa = n2F2νAI*/4RT, respectively,70,71 where Ipa, Ae, n, ν, C, F, R, and T, are the anodic peak current, geometrical surface area of the electrode, number of electrons transferred, scan rate, redox species concentration, Faraday constant, universal gas constant, and absolute temperature, respectively. Further, using the calculated D and Randles–Sevcik equation, the electroactive surface area (Ae) of all the electrodes was calculated (ESI,† Table S3), and found that Ag-doped ZnO electrodes provide increased electroactive surface area for the loading of enzymes than that of pristine ZnO electrodes. According to Hrapovic et al.,72 nanomaterials with a larger electroactive surface area have higher electrocatalytic activity, resulting in increased sensitivity.
Furthermore, as shown in Fig. 6(A)(a)–(c), it was observed that the magnitude of the peak current significantly decreases when GOx is immobilized onto the surface of the ZnO/ITO, ZnO:Ag2/ITO, and ZnO:Ag5/ITO electrodes. This is because of the insulating nature of the GOx layer, which acts as a barrier between the electrode surface and the redox species in the buffer solution, limiting charge transfer.73 In addition, CV measurements were also performed at different scan rates for the GOx/ZnO/ITO, GOx/ZnO:Ag2/ITO, and GOx/ZnO:Ag5/ITO samples (ESI,† Fig. S4(g–i)), indicating the quasi-reversible nature of the GOx immobilized electrodes. As listed in Table S3 (ESI†), it can be seen that the calculated values of the surface concentration of the GOx/ZnO/ITO, GOx/ZnO:Ag2/ITO and GOx/ZnO:Ag5/ITO electrodes differ from those of the ZnO/ITO, ZnO:Ag2/ITO, and ZnO:Ag5/ITO electrodes. All these results confirm the successful immobilization of GOx onto the surface of the ZnO/ITO, ZnO:Ag2/ITO, and ZnO:Ag5/ITO samples,72,73 which is also supported by the FTIR results (Fig. S3†).
5. Sensing measurements
Electrochemical sensors are an important subclass of chemical sensors in which an electrode is used as a transduction element. It works on the principle that an electrical current passes through a sensing electrode, which is produced by an electrochemical reaction that takes place at the electrode's surface.74 In the present work, we have investigated the electrochemical response of both prepared enzymatic and non-enzymatic electrodes for glucose sensing.
5.1 Enzymatic glucose sensing
Fig. 6(B)(a)–(c) show the CVs of the fabricated GOx/ZnO/ITO, GOx/ZnO:Ag2/ITO and GOx/ZnO:Ag5/ITO sensing electrodes in the presence of a glucose concentration of 0–8 mM. It was observed that the response current increases with increasing glucose concentration (corresponding inset of Fig. 6(B)), and saturates beyond a glucose concentration of 3 mM, 3 mM, and 4 mM for the GOx/ZnO/ITO, GOx/ZnO:Ag2/ITO and GOx/ZnO:Ag5/ITO sensing electrodes, respectively. The sensitivity and the LOD of the fabricated electrodes were calculated using eqn (S.1) and (S.2),† respectively, and are described in Table S3 (ESI†). It was observed that the GOx/ZnO:Ag5/ITO electrode exhibits excellent glucose-sensing characteristics (high sensitivity ∼98.3 μA mM−1 cm−2 and low LOD ∼0.098 mM) compared to the GOx/ZnO:Ag2/ITO (sensitivity ∼15.3 μA mM−1 cm−2 and LOD ∼0.451 mM) and GOx/ZnO/ITO (sensitivity ∼37.5 μA mM−1 cm−2 and LOD ∼0.204 mM) electrodes. The reason for the higher achieved sensitivity and lower LOD for the sample GOx/ZnO:Ag5/ITO is the incorporation of an optimum amount of Ag ions in the ZnO host matrix, resulting in a rapid electron transfer rate between the sensing electrode surface and electrochemically active species, as well as the improved catalytic character of GOx towards glucose.36 Further, the apparent Michaelis–Menten constant (Kappm) was calculated to examine the biological activity of immobilized GOx using the Lineweaver–Burk relation (eqn (1)),75 where Ipa, Ipa(max), and Cg are the anodic current, maximum anodic current, and concentration of glucose, respectively. | 1/Ipa = (Kappm/Ipa(max))(1/Cg) + 1/Ipa(max) | (1) |
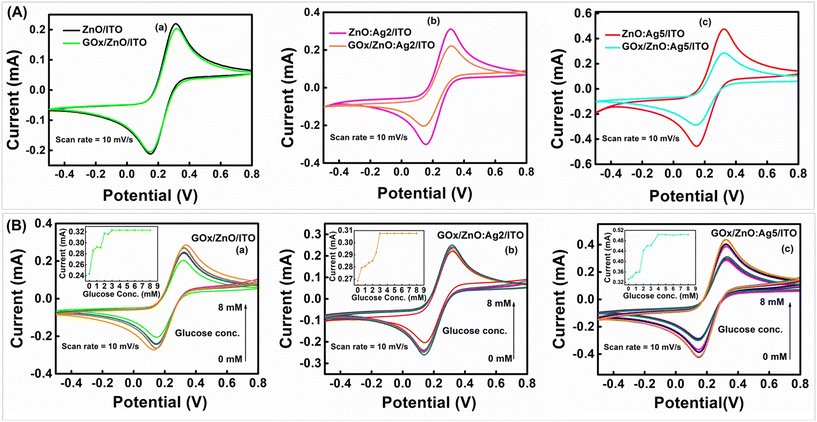 |
| Fig. 6 (A) CVs obtained for (a) ZnO/ITO and GOx/ZnO/ITO electrodes, (b) ZnO:Ag2/ITO and GOx/ZnO:Ag2/ITO, and (c) ZnO:Ag5/ITO and GOx/ZnO:Ag5/ITO electrodes at a scan rate of 10 mV s−1. (B) CVs of the prepared enzymatic sensing electrodes (a) GOx/ZnO/ITO, (b) GOx/ZnO:Ag2/ITO, (c) and GOx/ZnO:Ag5/ITO in the presence of 0–8 mM glucose at a scan rate of 10 mV s−1. The corresponding insets show the current variation obtained as a function of glucose concentration. | |
The value of Kappm for the GOx/ZnO/ITO, GOx/ZnO:Ag2/ITO, and GOx/ZnO:Ag5/ITO sensing electrodes was estimated to be ∼0.07, ∼0.04 and ∼0.26 mM, respectively. A smaller Kappm value indicates that the immobilized GOx has high enzymatic activity, and thus has a higher affinity for glucose for the fabricated sensing electrodes.76 However, enzymatic biosensors are unstable (influenced by a variety of parameters such as temperature, pH, etc.) and quite expensive.77,78 Therefore, in this work, we have also fabricated non-enzymatic electrodes based on the Ag-doped ZnO nanosheets for glucose sensing.
5.2 Non-enzymatic glucose sensing
In this study, our objective was to develop a non-enzymatic glucose sensor, which was evaluated based on the performance of Electrochemical Impedance Spectroscopy (EIS) (refer to Fig. 5(a)) and Cyclic Voltammetry (CV) (refer to Fig. 5(b)) for all the samples. With results shown in Fig. 5(a) and (b), it was observed that the 5% Ag-doped ZnO nanosheets (ZnO:Ag5/ITO) displayed excellent electrochemical characteristics, which is favorable for designing non-enzymatic glucose sensors. Thus, as presented in Fig. 7(A), we have fabricated a non-enzymatic electrode based on ZnO:Ag5/ITO and studied its glucose-sensing performance in the concentration range of 0–8 mM. The fabricated non-enzymatic electrode exhibited a higher sensitivity of ∼104.7 μA mM−1 cm−2 and good linearity of ∼0.981, with a lower LOD of ∼0.06 mM, in comparison to the enzymatic biosensor (GOx/ZnO:Ag5/ITO, sensitivity ∼98.3 μA mM−1 cm−2, LOD ∼0.098 mM and linearity ∼0.92). Moreover, as listed in Table 1, we have compared our fabricated glucose sensors based on Ag-doped ZnO nanosheets with previously reported glucose sensors based on pristine and doped-ZnO nanostructures.
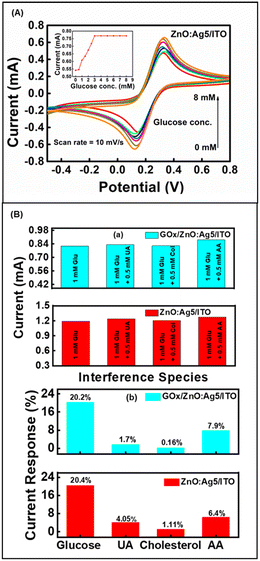 |
| Fig. 7 (A) CVs of non-enzymatic (ZnO:Ag5/ITO) sensing electrode in the presence of 0–8 mM glucose concentration at a scan rate of 10 mV s−1. The inset shows the current variation obtained as a function of glucose concentration. (B) (a) and (b) Effect of interfering species with 0.5 mM UA, cholesterol (Chol.), and AA each added to 1 mM glucose on the enzymatic (GOx/ZnO:Ag5/ITO) and non-enzymatic (ZnO:Ag5/ITO) electrodes. | |
Table 1 Comparison of the current work with previously reported enzymatic and non-enzymatic glucose sensing electrodes based on pristine and doped-ZnO nanostructures
Method used |
Sensing electrode |
Sensitivity (μA mM−1 cm−2) |
Linear range (mM) |
Response time (s) |
K
appm (mM) |
Ref. |
Enzymatic |
Non-enzymatic |
Electrochemical |
GOx/ZnO:Co |
— |
13.3 |
0–4 |
8 |
21 |
79
|
GOx/porous ZnO |
— |
23.4 |
0.1–1 |
7 |
— |
80
|
GOx/ZnO-NWs/graphite |
— |
13–17 |
— |
— |
2.11 |
81
|
GOx/ZnO Nanorod array |
— |
18.7 |
0.5–2.5 |
<5 |
1.3 |
82
|
GOx/ZnO–Al |
— |
5.5 |
0.28–28 |
10 |
66.7 |
83
|
GOx/ZnO–Ag |
— |
3.85 |
1.5 × 10−3–6.5 |
— |
3.87 |
21
|
GOx/ZnO(Co–Fe)/ITO |
— |
32.2 |
0–4 |
6.21 |
0.054 |
84
|
|
ZnO/MWCNT/GCE |
64.29 |
— |
— |
— |
12
|
|
ZnO/Ti |
7.65 |
3.33–11.1 |
— |
— |
85
|
GOx/ZnO:Ag5/ITO |
— |
∼98.3 |
0–4 |
∼5 |
∼0.26 |
This work |
|
ZnO:Ag5/ITO |
∼104.7 |
0–3 |
∼5 |
— |
This work |
Method used |
Sensing electrode |
Sensitivity (% mM−1) |
Linear range (mM) |
Response time (s) |
K
appm (mM) |
Ref. |
Enzymatic |
Non-enzymatic |
Fluorescence spectroscopy |
— |
ZnO nanotubes |
3.5 |
0.1–15 |
— |
— |
86
|
— |
ZnO nanorods |
1.4 |
0.5–30 |
— |
— |
87
|
Gox/ZnO nanoparticles |
— |
0.5 |
10–130 |
— |
— |
88
|
Method used |
Sensing electrode |
Sensitivity |
Linear range |
Response time |
K
appm
|
Ref. |
Enzymatic |
Non-enzymatic |
Surface plasmon resonance |
GOx/ZnO/Au/prism |
— |
— |
0–300 mg dl−1 |
— |
— |
89
|
Surface enhanced Raman spectroscopy |
GOx/CdSe/ ZnS QDs decorated ZnO NRs |
— |
— |
0.03–3 mM |
— |
— |
90
|
Furthermore, anti-interference ability (selectivity), the effect of temperature, repeatability, and reproducibility are important aspects for the practical application of fabricated sensing electrodes (ESI† Fig. S6 and S7). It is well known that a few electro-active species such as dopamine, cholesterol, ascorbic acid, uric acid, cysteine, etc. in serum may affect the performance of a biosensor.91 Thus, the anti-interference capability and selectivity of the prepared biosensor have been studied by introducing electro-active species: uric acid (UA), cholesterol (Chol.), and ascorbic acid (AA). Fig. 7(B) shows the anti-interference ability of the fabricated enzymatic and non-enzymatic sensing electrodes based on ZnO:Ag5 nanosheets. Selectivity measurements were performed with the addition of the interfering species 0.5 mM UA, 0.5 mM Chol., and 0.5 mM AA to a standard solution of 1 mM glucose concentration. As shown in Fig. 7(B)(a), although the interfering species slightly increased the current response, these results can be ruled out, as the highest concentration of these interfering species in the human body is approximately 0.1 mM.68,92 Consequently, the sensing electrodes can be used to determine glucose levels in human serum samples under physiological conditions. As presented in Fig. 7(B)(b), the ZnO:Ag5 nanosheet based enzymatic and non-enzymatic sensing electrodes exhibited a current response of ∼20.2% (1 mM glucose), ∼1.7% (0.5 mM UA), ∼0.16% (0.5 mM Chol.), and ∼7.9% (0.5 mM AA), and ∼20.4% (1 mM glucose), ∼4.05% (for 0.5 mM UA), 1.11% (for 0.5 mM Chol.), and 6.4% (for 0.5 mM AA), respectively. These results show that both the enzymatic and non-enzymatic sensing electrodes based on the ZnO:Ag5 nanosheets are highly selective towards glucose; however, the non-enzymatic sensing electrode has better selectivity towards glucose as compared to the enzymatic electrode.
Fig. 8(a) and (b) show the effect of temperature (5 °C to 60 °C) on both enzymatic and non-enzymatic sensing electrodes. It is found that the non-enzymatic sensing electrode exhibited a small change in current response (∼0.25–13%) with temperature compared to the enzymatic sensing electrode (∼0.12–28%), indicating that the non-enzymatic glucose sensing electrode has better temperature stability in comparison to the enzymatic glucose sensing electrode. As presented in Fig. 8(c) and (d), the repeatability of the both prepared enzymatic and non-enzymatic electrodes was determined by analyzing the current response in 1 mM glucose 10 times in a single day (ESI† Fig. S5(a and b)). The relative standard deviation (RSD) was calculated for the 10 repeated cycles and was found to be 2.4% and 0.3% for the enzymatic and non-enzymatic sensing electrodes, respectively, revealing the excellent repeatability of the fabricated electrodes. Likewise, as shown in Fig. 8(e) and (f), we have also investigated the reproducibility of both enzymatic and non-enzymatic sensing electrodes which was examined by analyzing the current response in 1 mM glucose for five distinct electrodes (ESI† Fig. S5(c and d)). The calculated RSD values for both enzymatic and non-enzymatic sensing electrodes were found to be ∼1.9% and ∼1.8%, respectively, suggesting good reproducibility. Besides, it can be seen from Fig. 8(c–f) that the fabricated non-enzymatic glucose sensing electrode has better repeatability and reproducibility than the enzymatic glucose sensing electrode. Next, the stability of both electrodes was tested by measuring the current response in 1 mM glucose for 16 days. When not in use, the modified enzymatic electrode was kept dry at 4 °C and the non-enzymatic electrode was kept in a desiccator. As shown in Fig. 8(g) and (h), the prepared enzymatic glucose sensing electrode maintained 97% of its original response after 8 days and 90.5% after 16 days, and the non-enzymatic glucose sensing electrode maintained 98% of its initial response after 8 days and 92% after 16 days, suggesting high storage stability.
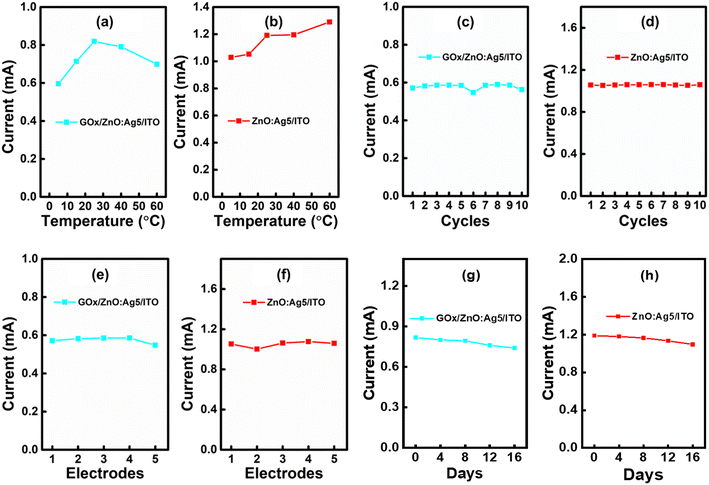 |
| Fig. 8 Effect of temperature on the fabricated (a) enzymatic (GOx/ZnO:Ag5/ITO) and (b) non-enzymatic (ZnO:Ag5/ITO) sensing electrodes at 1 mM glucose concentration. Current response of the (c) enzymatic (GOx/ZnO:Ag5/ITO) and (d) non-enzymatic (ZnO:Ag5/ITO) sensing electrodes for 10 cycles at 1 mM glucose concentration. Current response of the (e) enzymatic (GOx/ZnO:Ag5/ITO) and (f) non-enzymatic (ZnO:Ag5/ITO) sensing electrodes for 5 different electrodes at 1 mM glucose concentration. Current response of the (g) enzymatic (GOx/ZnO:Ag5/ITO) and (h) non-enzymatic (ZnO:Ag5/ITO) sensing electrodes at 1 mM glucose concentration over 16 days. | |
6. Mechanism of glucose sensing
Fig. 9A shows a schematic diagram of CV analysis of the prepared electrodes for glucose monitoring using an electrochemical workstation. Schematic representations of the electrochemical oxidation of glucose on the ZnO:Ag5 nanosheet based ITO electrode surface for enzymatic and non-enzymatic glucose sensing are shown in Fig. 9B(a) and (b), respectively. The electrochemical recognition of glucose was performed using Fe[(CN)6]3−/4− as a redox mediator to avoid electrode contamination and improve redox cycling.90 As presented in Fig. 9B(a), immobilized GOx on the ZnO:Ag5 nanosheet-based electrode oxidizes glucose to gluconolactone while simultaneously converting from GOx(FAD) into GOx(FADH2). Then, the redox mediator Fe[(CN)6]3− reacts with the reduced GOx and accepts electrons to become electro-reduced Fe[(CN)6]4− and regenerates the oxidized GOx. Further, these electrons are transported from Fe[(CN)6]4− to ZnO:Ag5 nanosheets and eventually to the ITO electrode.93–96 An oxidation current is generated when Fe[(CN)6]4− returns to its oxidized state Fe[(CN)6]3−. Next, the probable mechanism of glucose oxidation on the ZnO:Ag5 nanosheet surface for the application of a non-enzymatic glucose sensor is depicted in Fig. 9B(b). At first, glucose is oxidized to gluconolactone by releasing electrons, and then the redox mediator Fe[(CN)6]3− accepts these electrons and becomes Fe[(CN)6]4−. Further, these electrons transfer to the nanosheets, resulting in an oxidation current.95,97 Thus, the current response obtained in the presence of glucose (Fig. 6(B) and 7(A)) is caused by these released electrons for both enzymatic and non-enzymatic glucose sensing.
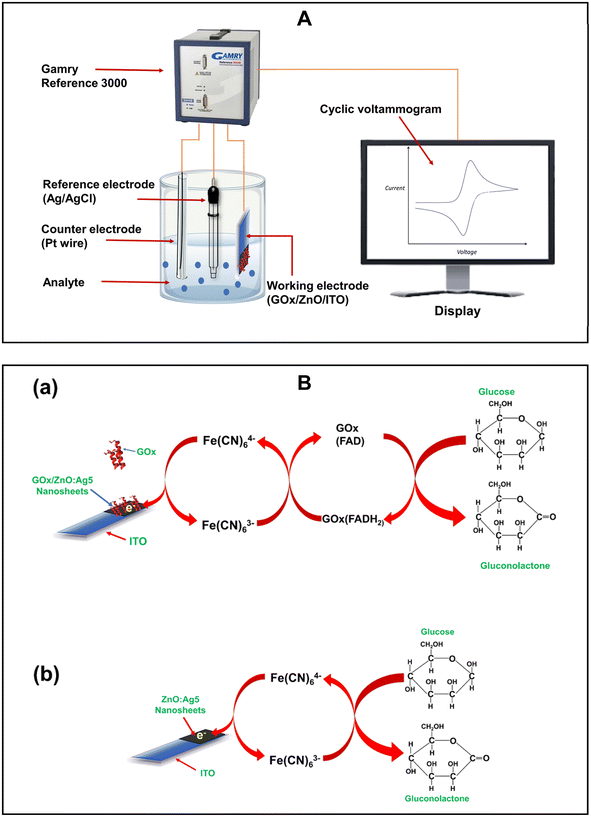 |
| Fig. 9 A. Schematic of the electrochemical (CV) analysis using an electrochemical workstation. B. The schematic representations of the electrochemical oxidation of glucose for the (a) enzymatic (GOx/ZnO:Ag5/ITO) and (b) non-enzymatic (ZnO:Ag5/ITO) sensing electrodes. | |
7. Conclusions
In summary, pristine and Ag-doped ZnO nanosheets were successfully synthesized by a hydrothermal method and it was found that Ag doping improved the electrochemical characteristics of the ZnO matrix, which has a significant impact on the glucose-sensing performance with and without the enzyme GOx. FESEM images revealed the growth of nanosheet-like morphologies in pristine and Ag-doped ZnO samples and it was also observed that the grain size and thickness decrease with increasing Ag-dopant concentration in the ZnO host matrix. The XRD, EDX, XPS, and FTIR results showed the formation of pristine ZnO and successful incorporation of Ag dopants into the ZnO matrix, including the presence of different defect sites in the samples. Further, FTIR spectra revealed the successful immobilization of GOx on the surface of the pristine ZnO and Ag-doped ZnO samples. For application as glucose sensors, the pristine and Ag-doped ZnO-based ITO electrodes are studied electrochemically. Among all prepared electrodes, the 5% Ag-doped ZnO nanosheet electrode showed a higher conductivity than the pristine and other Ag-doped ZnO electrodes, which may be due to the introduction of defect states by Ag doping in the ZnO host matrix as well as the improved surface area. At room temperature, for non-enzymatic glucose sensing, the 5% Ag-doped ZnO nanosheet electrode exhibited higher sensitivity (∼104.7 μA mM−1 cm−2), lower detection limits (∼0.06 mM), good linearity (∼0.981), higher selectivity, reasonable repeatability, and good reproducibility and stability compared to that for enzymatic glucose sensing (sensitivity ∼98.3 μA mM−1 cm−2, LOD ∼0.098 mM and linearity ∼0.92). We demonstrate a high sensing performance without using any enzyme on Ag-doped ZnO nanosheets fabricated via a simple approach which may be a potential candidate for low-cost biosensors and could have a wide range of applications in clinical diagnosis, industry, and food science.
Conflicts of interest
The authors declare that they have no known competing financial interests or personal relationships that could have appeared to influence the work reported in this paper.
Acknowledgements
The authors Sanjeev Kumar and Jyoti Jaiswal would like to acknowledge the Science and Engineering Research Board, Government of India for the financial support with Grant No. CRG/2022/001635 and the Department of Science and Technology, Government of India for the financial support with Grant No. SR/PURSE/2021/58. The author Saptaka Baruah would like to acknowledge Department of Science and Technology, Government of India for the financial assistance (Grant No. SR/PURSE/2021/58). The authors would also like to acknowledge the Department of Physics and Chemistry, Rajiv Gandhi University, Itanagar-791112, India.
References
- E. E. Ventura, J. N. Davis and M. I. Goran, Obesity, 2011, 19(4), 868–874 CrossRef CAS PubMed.
- F. Conzuelo, M. Gamella, S. Campuzano, M. A. Ruiz, A. J. Reviejo and J. M. Pingarron, J. Agric. Food Chem., 2010, 58(12), 7141–7148 CrossRef CAS PubMed.
- V. Scognamiglio, Biosens. Bioelectron., 2013, 47, 12–25 CrossRef CAS PubMed.
- N. S. Ridhuan, K. A. Razak and Z. Lockman, Sci. Rep., 2018, 8(1), 1–12 CAS.
- P. Hu, R. Zhou, D. Wang, H. Luo, X. Xiong, K. Huang, L. Li and P. Chen, Sens. Actuators, B, 2020, 308, 127702 CrossRef CAS.
- B. Liu, Z. Sun, P. J. Huang and J. Liu, J. Am. Chem. Soc., 2015, 137, 1290–1295 CrossRef CAS PubMed.
- H. He, X. Xu, H. Wu and Y. Jin, Adv. Mater., 2012, 24(13), 1736–1740 CrossRef CAS PubMed.
- H. Teymourian, A. Barfidokht and J. Wang, Chem. Soc. Rev., 2020, 49(21), 7671–7709 RSC.
- A. Heller and B. Feldman, Chem. Rev., 2008, 108(7), 2482–2505 CrossRef CAS PubMed.
- A. Wei, X. W. Sun, J. X. Wang, Y. Lei, X. P. Cai, C. M. Li and W. Huang, Appl. Phys. Lett., 2006, 89(12), 123902 CrossRef.
- T. Kong, Y. Chen, Y. Ye, K. Zhang, Z. Wang and X. Wang, Sens. Actuators, B, 2009, 38(1), 344–350 CrossRef.
- S. M. U. Ali, O. Nur, M. Willander and B. Danielsson, Sens. Actuators, B, 2010, 145(2), 869–874 CrossRef.
- C. L. Hsu, J. H. Lin, D. X. Hsu, S. H. Wang, S. Y. Lin and T. J. Hsueh, Sens. Actuators, B, 2017, 238, 150–159 CrossRef CAS.
- Z. Wang, J. Zhang, R. Jian, J. Liao, X. Xiong and K. Huang, Microchem. J., 2020, 159, 105396 CrossRef CAS.
- S. D. Lawaniya, S. Kumar, Y. Yu, H. G. Rubahn, Y. K. Mishra and K. Awasthi, Mater. Today Chem., 2023, 29, 101428 CrossRef CAS.
- N. Tripathy and D. H. Kim, Metal oxide modified ZnO nanomaterials for biosensor applications, Nano Convergence, 2018, 5(1), 1–10 CrossRef PubMed.
- A. Tereshchenko, M. Bechelany, R. Viter, V. Khranovskyy, V. Smyntyna, N. Starodub and R. Yakimova, Sens. Actuators, B, 2016, 229, 664–677 CrossRef CAS.
- L. Cao, J. Kiely, M. Piano and R. Luxton, Sci. Rep., 2018, 8(1), 1–9 Search PubMed.
- N. R. Shanmugam, S. Muthukumar and S. Prasad, Future Sci. OA, 2017, 3(4), FSO196 CrossRef CAS PubMed.
- J. Li, S. Ma, X. Liu, Z. Zhou and C. Q. Sun, Chem. Rev., 2012, 112(5), 2833–2852 CrossRef CAS PubMed.
- B. Ortiz-Casas, A. Galdámez-Martínez, J. Gutiérrez-Flores, A. B. Ibañez, P. K. Panda, G. Santana and A. Dutt, Mater. Today, 2021, 50, 533–569 CrossRef CAS.
- Z. L. Wang, Mater. Today, 2004, 7(6), 26–33 CrossRef CAS.
- N. Izyumskaya, V. Avrutin, Ü. Özgür, Y. I. Alivov and H. Morkoç, Phys. Status Solidi B, 2007, 244(5), 1439–1450 CrossRef CAS.
- A. Mahmoud, M. Echabaane, K. Omri, J. Boudon, L. Saviot, N. Millot and R. B. Chaabane, Molecules, 2021, 26(4), 929 CrossRef CAS PubMed.
- W. Raza and K. Ahmad, Mater. Lett., 2018, 212, 231–234 CrossRef CAS.
- J. Luo, P. Luo, M. Xie, K. Du, B. Zhao, F. Pan and G. Liang, Biosens. Bioelectron., 2013, 49, 512–518 CrossRef CAS PubMed.
- M. Shukla, J. Agrawal, T. Dixit, I. A. Palani and V. Singh, Mater. Res. Express, 2018, 5(5), 055031 CrossRef.
- J. Ghosh, R. Ghosh and P. K. Giri, Sens. Actuators, B, 2018, 254, 681–689 CrossRef CAS.
- G. Vijayaprasath, R. Murugan, J. S. Narayanan, V. Dharuman, G. Ravi and Y. Hayakawa, J. Mater. Sci.: Mater. Electron., 2015, 26(7), 4988–4996 CrossRef CAS.
- A. Mahmoud, M. Echabaane, K. Omri, L. El Mir and R. B. Chaabane, J. Alloys Compd., 2019, 786, 960–968 CrossRef CAS.
-
W. C. Peng, Z. H. Wang, C. C. Yang, C. S. Huang, Y. K. Su and J. L. Ruan, in SPIE Proc. Biomed. Imag. Sens. Conf., 2017, vol. 10251, pp. 165–167 Search PubMed.
- P. Chakraborty, T. Majumder, S. Dhar and S. P. Mondal, AIP Conf. Proc., 2018, 1942, 050074 CrossRef.
- S. S. Patil, M. G. Mali, M. S. Tamboli, D. R. Patil, M. V. Kulkarni, H. Yoon, H. Kim, S. S. Al-Deyab, S. S. Yoon, S. S. Kolekar and B. B. Kale, Catal. Today, 2016, 260, 126–134 CrossRef CAS.
- Y. Zheng, L. Zheng, Y. Zhan, X. Lin, Q. Zheng and K. Wei, Inorg. Chem., 2007, 46, 6980–6986 CrossRef CAS PubMed.
- F. Xian, K. Miao, X. Bai, Y. Ji, F. Chen and X. Li, Optik, 2013, 124, 4876–4879 CrossRef CAS.
- F. Zhou, W. Jing, P. Liu, D. Han, Z. Jiang and Z. Wei, Sensors, 2017, 17(10), 2214 CrossRef PubMed.
- J. Jaiswal, A. Sanger, P. Tiwari and R. Chandra, Sens. Actuators, B, 2020, 305, 127437 CrossRef CAS.
- C. Li, Y. Lin, F. Li, L. Zhu, D. Sun, L. Shen and S. Ruan, RSC Adv., 2015, 5(98), 80561–80567 RSC.
- X. Pan, X. Liu, A. Bermak and Z. Fan, ACS Nano, 2013, 7(10), 9318–9324 CrossRef CAS PubMed.
- A. Fulati, S. M. U. Ali, M. H. Asif, M. Willander, C. Brännmark, P. Strålfors and B. Danielsson, Sens. Actuators, B, 2010, 150(2), 673–680 CrossRef CAS.
- N. Akhtar, S. K. Metkar, A. Girigoswami and K. Girigoswami, Mater. Sci. Eng., C, 2017, 78, 960–968 CrossRef CAS PubMed.
- H. M. Kim, J. H. Park and S. K. Lee, Sci. Rep., 2019, 9(1), 1–9 CrossRef PubMed.
- F. Zhang, X. Wang, S. Ai, Z. Sun, Q. Wan, Z. Zhu and K. Yamamoto, Anal. Chim. Acta, 2004, 519(2), 155–160 CrossRef CAS.
- M. Liu, P. Song, D. Liang, Z. Yang and Q. Wang, Mater. Res. Express, 2019, 6(12), 125910 CrossRef CAS.
- J. Jaiswal, A. Das, V. Chetry, S. Kumar and R. Chandra, Sens. Actuators, B, 2022, 359, 131552 CrossRef CAS.
- S. K. Arya, S. Saha, J. E. Ramirez-Vick, V. Gupta, S. Bhansali and S. P. Singh, Anal. Chim. Acta, 2012, 737, 1–21 CrossRef CAS PubMed.
- N. Baig, I. Kammakakam and W. Falath, Mater. Adv., 2021, 2(6), 1821–1871 RSC.
- E. Andrade and M. Miki-Yoshida, Thin Solid Films, 1999, 350(1–2), 192–202 Search PubMed.
- A. Kołodziejczak-Radzimska and T. Jesionowski, Materials, 2014, 7(4), 2833–2881 CrossRef PubMed.
- K. S. Babu and V. Narayanan, Chem. Sci. Trans., 2013, 2(S1), S33–S36 Search PubMed.
- S. A. Kaur, G. S. Randhawa and R. Singh, IOSR J. Appl. Phys., 2017, 9, 18–24 CrossRef.
- R. S. Zeferino, M. B. Flores and U. Pal, J. Appl. Phys., 2011, 109(1), 014308 CrossRef.
- S. Khosravi-Gandomani, R. Yousefi, F. Jamali-Sheini and N. M. Huang, Ceram. Int., 2014, 40(6), 7957–7963 CrossRef CAS.
- B. D. Ahn, H. S. Kang, J. H. Kim, G. H. Kim, H. W. Chang and S. Y. Lee, J. Appl. Phys., 2006, 100(9), 093701 CrossRef.
- C. Karunakaran, V. Rajeswari and P. Gomathisankar, Superlattices Microstruct., 2011, 50(3), 234–241 CrossRef CAS.
- J. Jaiswal, P. Tiwari, P. Singh and R. Chandra, Sens. Actuators, B, 2020, 325, 128800 CrossRef CAS.
- S. K. Pandey, S. K. Pandey, C. Mukherjee, P. Mishra, M. Gupta, S. R. Barman and S. Mukherjee, J. Mater. Sci.: Mater. Electron., 2013, 24(7), 2541–2547 CrossRef CAS.
- A. Meng, S. Sun, Z. Li and J. Han, Adv. Powder Technol., 2013, 24(1), 224–228 CrossRef CAS.
- S. M. Hosseini, I. A. Sarsari, P. Kameli and H. Salamati, J. Alloys Compd., 2015, 640, 408–415 CrossRef CAS.
- J. Jaiswal, P. Singh and R. Chandra, Sens. Actuators, B, 2021, 327, 128862 CrossRef CAS.
- S. Gandla, S. R. Gollu, R. Sharma, V. Sarangi and D. Gupta, Appl. Phys. Lett., 2015, 107(15), 152102 CrossRef.
- K. M. Wong, Y. Fang, A. Devaux, L. Wen, J. Huang, L. De Cola and Y. Lei, Nanoscale, 2011, 3(11), 4830–4839 RSC.
- L. Jing, Z. Xu, J. Shang, X. Sun, W. Cai and H. Guo, Mater. Sci. Eng., A, 2002, 332, 356–361 CrossRef.
- I. Ahmad, E. Ahmad and M. Ahmad, SN Appl. Sci., 2019, 1(4), 1–12 Search PubMed.
- M. Karyaoui, D. B. Ahmed, M. Jemia, I. B. Gannouni, A. Assaker, M. Amlouk Bardaoui and R. Chtourou, Inorg. Chem. Commun., 2020, 119, 108114 CrossRef CAS.
- K. Jindal, M. Tomar and V. Gupta, Analyst, 2013, 138(15), 4353–4362 RSC.
- E. A. Gomaa, A. Negm and R. M. Abu-Qarn, Measurement, 2018, 125, 645–650 CrossRef.
- N. S. Ridhuan, K. A. Razak and Z. Lockman, Sci. Rep., 2018, 8(1), 1–12 CAS.
- L. Fotouhi, M. Fatollahzadeh and M. M. Heravi, Int. J. Electrochem. Sci., 2012, 7, 3919–3928 CrossRef CAS.
-
A. J. Bard, L. R. Faulkner and H. S. White, Electrochemical methods: Fundamentals and applications, John Wiley & Sons, 2022 Search PubMed.
- A. Sharma, D. Baral, H. B. Bohidar and P. R. Solanki, Chem.-Biol. Interact., 2015, 238, 129–137 CrossRef CAS PubMed.
- S. Hrapovic, Y. Liu, K. B. Male and J. H. T. Luong, Anal. Chem., 2004, 76(4), 1083–1088 CrossRef CAS PubMed.
- A. Sharma, K. Rawat, P. R. Solanki and H. B. Bohidar, Anal. Methods, 2015, 7(14), 5876–5885 RSC.
-
F. R. Simões and M. G. Xavier, Nanosci. and its Appl., 2017, vol. 1, pp. 155–178 Search PubMed.
- R. Ahmad, N. Tripathy, J. H. Kim and Y. B. Hahn, Sens. Actuators, B, 2012, 174, 195–201 CrossRef CAS.
- J. R. Li, Y. K. Du, P. Boullanger and L. Jiang, Thin Solid Films, 1999, 352(1–2), 213–217 CrossRef CAS.
- L. Alvarado-Ramírez, M. Rostro-Alanis, J. Rodríguez-Rodríguez, J. E. Sosa-Hernández, E. M. Melchor-Martínez, H. Iqbal and R. Parra-Saldívar, Biosensors, 2021, 11(11), 410 CrossRef PubMed.
- V. Naresh and N. Lee, Sensors, 2021, 21(4), 1109 CrossRef CAS PubMed.
- Z. W. Zhao, X. J. Chen, B. K. Tay, J. S. Chen, Z. J. Han and K. A. Khor, Biosens. Bioelectron., 2007, 23(1), 135–139 CrossRef CAS PubMed.
- H. Fatemi, A. A. Khodadadi, A. A. Firooz and Y. Mortazavi, Curr. Appl. Phys., 2012, 12(4), 1033–1038 CrossRef.
- P. Gallay, E. Tosi, R. Madrid, M. Tirado and D. Comedi, Nanotechnology, 2016, 27(42), 425501 CrossRef CAS PubMed.
- M. Shukla, T. Dixit, R. Prakash, I. A. Palani and V. Singh, Appl. Surf. Sci., 2017, 422, 798–808 CrossRef CAS.
- V. T. K. P. Fidal, S. Inguva, S. Krishnamurthy, E. Marsili, J. P. Mosnier and T. S. Chandra, Enzyme Microb. Technol., 2017, 96, 67–74 CrossRef PubMed.
- S. Baruah, B. Maibam, C. K. Borah, T. Agarkar, A. Kumar and S. Kumar, IEEE Sens. J., 2021, 21(13), 14601–14608 CAS.
- G. Villasana-Ponce, F. Chalé-Lara, J. Olarte Villamizar, M. Zapata-Torres, E. Valaguez Velázquez, N. Cruz González and A. Márquez Herrera, Superficies Vacio, 2019, 32, 22–26 CrossRef CAS.
- H. H. Mai, D. H. Tran and E. Janssens, Microchim. Acta, 2019, 186, 1–10 CrossRef CAS PubMed.
- S. N. Sarangi, S. Nozaki and S. N. Sahu, J. Biomed. Nanotechnol., 2015, 11(6), 988–996 CrossRef CAS PubMed.
- D. Sodzel, V. Khranovskyy, V. Beni, A. P. Turner, R. Viter, M. O. Eriksson and R. Yakimova, Microchim. Acta, 2015, 182, 1819–1826 CrossRef CAS.
- A. Paliwal, R. Gaur, A. Sharma, M. Tomar and V. Gupta, Optik, 2016, 127(19), 7642–7647 CrossRef CAS.
- L. Chen, W. H. Tse, Y. Chen, M. W. Melling, J. McDonald and J. Zhang, Biosens. Bioelectron., 2017, 91, 393–399 CrossRef CAS PubMed.
- R. Sha, A. Basak, P. C. Maity and S. Badhulika, Sens. Actuators Rep., 2022, 4, 100098 CrossRef.
- G. Aydoğdu, D. K. Zeybek, Ş. Pekyardımcı and E. Kılıç, Artif. Cells, Nanomed., Biotechnol., 2013, 41(5), 332–338 CrossRef PubMed.
- D. Sharma, Y. Lim, Y. Lee and H. Shin, Anal. Chim. Acta, 2015, 88, 194–202 CrossRef PubMed.
- S. H. Lee, H. Y. Fang, W. C. Chen, H. M. Lin and C. A. Chang, Anal. Bioanal. Chem., 2005, 383(3), 532–538 CrossRef CAS PubMed.
- H. Deng, A. K. L. Teo and Z. Gao, Sens. Actuators, B, 2014, 191, 522–528 CrossRef CAS.
- K. Tian, S. Alex, G. Siegel and A. Tiwari, Mater. Sci. Eng., C, 2015, 46, 548–552 CrossRef CAS PubMed.
- A. Senthamizhan, B. Balusamy and T. Uyar, Anal. Bioanal. Chem., 2016, 408(5), 1285–1306 CrossRef CAS PubMed.
|
This journal is © The Royal Society of Chemistry 2023 |
Click here to see how this site uses Cookies. View our privacy policy here.