DOI:
10.1039/D3SC03934J
(Perspective)
Chem. Sci., 2023,
14, 12430-12446
Flexibility in zeolites: origin, limits, and evaluation
Received
29th July 2023
, Accepted 12th October 2023
First published on 23rd October 2023
Abstract
Numerous pieces of evidence in the literature suggest that zeolitic materials exhibit significant intrinsic flexibility as a consequence of the spring-like behavior of Si–O and Al–O bonds and the distortion ability of Si–O–Si and Al–O–Si angles. Understanding the origin of flexibility and how it may be tuned to afford high adsorption selectivity in zeolites is a big challenge. Zeolite flexibility may be triggered by changes in temperature, pressure, or chemical composition of the framework and extra-framework compounds, as well as by the presence of guest molecules. Therefore, zeolite flexibility can be classified into three categories: (i) temperature and pressure-induced flexibility; (ii) guest-induced flexibility; and (iii) compositionally-induced flexibility. An outlook on zeolite flexibility and the challenges met during the precise experimental evaluations of zeolites will be discussed. Overcoming these challenges will provide an important tool for designing novel selective adsorbents.
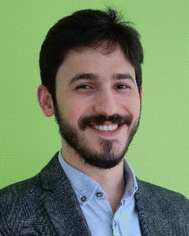 Sajjad Ghojavand | Sajjad Ghojavand received his B.Sc. in Chemical Engineering from Ferdowsi University of Mashhad (Iran) and has been working on different projects related to natural gas processing disciplines. He was awarded an Erasmus Mundus scholarship to pursue triple M.Sc. degrees as part of the European program entitled “Membrane Engineering for a Sustainable World (EM3E-4SW)” at the University of Montpellier (France), University of Chemistry and Technology Prague (Czech Republic), and University of Twente (the Netherlands). He got his PhD in chemistry in LCS under the supervision of Dr Mintova where he works as a post-doc on the synthesis of novel nanozeolites for CO2 capture and separation supported by TotalEnergies. His research involves developing novel methodologies to characterize and synthesize flexible zeolites. |
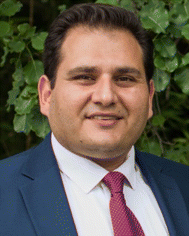 Eddy Dib | Eddy Dib received his PhD degree in physical chemistry from the National Graduate School of Engineering of Montpellier, and his HDR degree from the University of Caen and is now a CNRS researcher in Orléans, France. His research interests cover the understanding of the relationships between local order/disorder and the properties of zeolites combining experimental and theoretical approaches of multinuclear solid-state nuclear magnetic resonance (NMR). |
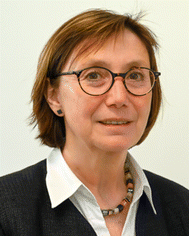 Svetlana Mintova | Svetlana Mintova is the Director of Research (DR1) at CNRS, LCS-ENSICAEN-Normandy University and Head of the Centre for Zeolites and Nanoporous Materials. The focus of her research is in the fields of porous materials and physical chemistry with expertise in the synthesis of zeolites, advanced characterization and their applications in catalysis, separation, chemical sensors, membranes and biomedicine. She is also a Visiting Professor at the China University of Petroleum (UPC), President of the International Zeolite Association (IZA), President of the European Zeolite Associations Federation (FEZA), and Chair of the “Synthesis Commission”. She is the Associate Editor of Inorganic Chemistry Frontiers (RSC) and Editor of Microporous Mesoporous Materials (Elsevier) and receiver of the ERC Advanced Grant 2022. |
1. Introduction
Zeolites are microporous materials that play a key role in industrial applications as catalysts, gas adsorbents and ion exchangers in many processes intimately related to environmental and economic challenges.1–3 Different approaches have been applied to control the selectivity of zeolite adsorbents and simultaneously control the adsorption and release of guest molecules.4–7 One of the key contributors to the selectivity towards adsorbed gas molecules was recently regarded to result from the zeolite framework's flexibility.8Yet, there is not a generally established definition in the literature of the term “flexibility of zeolites”. This flexibility may be defined as a reversible framework deformation (expansion/contraction) or dynamics of the zeolite structure including the movement of extra-framework cations, an intrinsic property of zeolitic frameworks due to the spring-like behavior of Si–O and Al–O bonds and the distortion of Si–O–Al and Si–O–Si angles as a response to an external trigger such as gas adsorption/desorption or a change in temperature or pressure.5,9–11 This spring-like behavior was used in the development of flexible force field parameters for the modeling of porous materials (zeolites) using Monte Carlo calculations or molecular dynamics simulations for many years.12–20 These models allow us to simulate the dynamics-related parameters in zeolites such as diffusion coefficients.21–25 Moreover, theoretical calculations of minimal and maximal possible framework densities showed that zeolitic frameworks exhibit a flexibility window in their all silica forms and almost all of them show some degree of flexibility in their aluminosilicate forms.26–28 As a result of these framework density calculations, 25 zeolitic frameworks were highlighted as potentially flexible structures: ACO, AST, ASV, DFO, EAB, EMT, ERI, FAU, KFI, LEV, LTA, LTL, MER, MOZ, MTN, OFF, PAU, RHO, SAS, SOD, SSF, TSC, UFI, UOS, and UOZ.29,30
The term flexibility window and its related parameters are merely theoretical and they have been used to predict the synthesis of millions of hypothetical zeolitic frameworks.29,31 However, a cohesive experimental factor describing zeolite flexibility is still not established and different aspects of zeolite flexibility are not fully explored yet. In this contribution, the different aspects of zeolite flexibility are summarized into three categories: (i) temperature and pressure-induced flexibility; (ii) guest-induced flexibility; and (iii) compositionally-induced flexibility.
1.1. Temperature and pressure-induced flexibility
Zeolite flexibility can be observed as a response to temperature and pressure changes. Temperature-induced flexibility usually manifests as alteration of the lattice parameters of the zeolite framework (e.g. RHO, SOD, MFI, etc.) or by migration of extra-framework cations within the zeolite structure (e.g. RHO, CHA, etc.).3,10,32 Zeolites undergo alterations in their lattice parameters when subjected to pressure.11,33–35 These alterations are reversible until they reach maximum pressure, commonly referred to as the ‘threshold pressure’ in the literature.11,33–35 These physical triggers can occur in gas adsorption, gas storage, and sensing applications.
1.1.1. Temperature-induced flexibility.
The change in the zeolite's lattice parameters in response to temperature variation was the first to be highlighted in the literature.36–40 The most prominent example is the work reported for RHO zeolite in 1984.36 The structural flexibility of RHO zeolites can be observed due to changes in temperature, however, the effect of temperature can also be linked to guest molecules (dehydration) and extra-framework cation behavior. RHO zeolite has shown a unit cell deformation and, subsequently, a change in its symmetry from acentric (I
3m) to centric (Im
m) when heated from 30 to 800 °C and these results were supported by both theoretical and experimental data (a variation of around 8% in RHO's unit cell volume – see Fig. 1).37,41–43
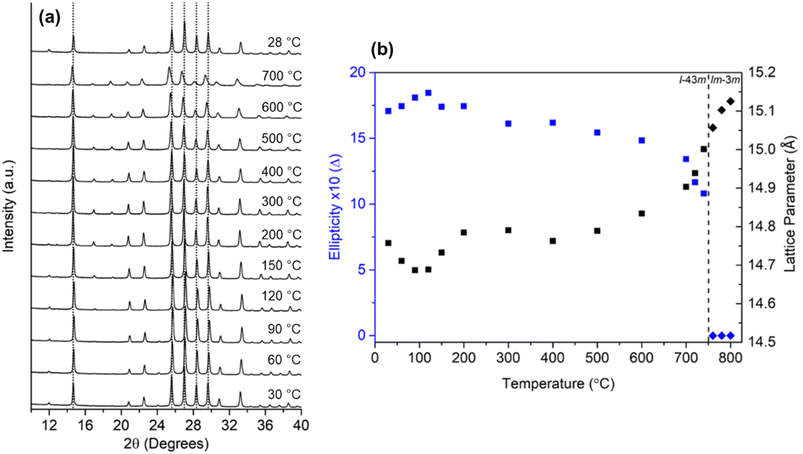 |
| Fig. 1 (a) In situ variable-temperature XRD scans of nanosized RHO zeolite from 30 to 700 °C and back to 28 °C, (b) plot of the ellipticity of eight-membered ring opening (in blue) and the lattice parameter (black). The dashed line delineates the adoption of either the acentric (squares) or centric (diamonds) space groups (reprinted with permission from ref. 43, copyright 2022 American Chemical Society). | |
Recently, our group observed such changes in the zeolite RHO's lattice parameter by in situ variable-temperature XRD measurements and the expansion of the lattice parameter was also visualized by in situ TEM imaging (Fig. 2) which was identified due to the temperature-induced oscillations of the extra-framework Cs+ around their average position.32 Another example is the encapsulation of Ar and Kr atoms (kinetic diameters of 3.3 and 3.6 Å, respectively) inside the sodalite framework with a pore diameter of 2.4 Å.44 Admission of these gases was only possible because of sodalite's framework flexibility at elevated temperatures as it was shown by molecular dynamics simulations.45 Similar behavior about the flexibility of zeolites at higher temperatures was observed for other zeolite topologies, e.g. MFI, MEL, and CHA.38,43,46
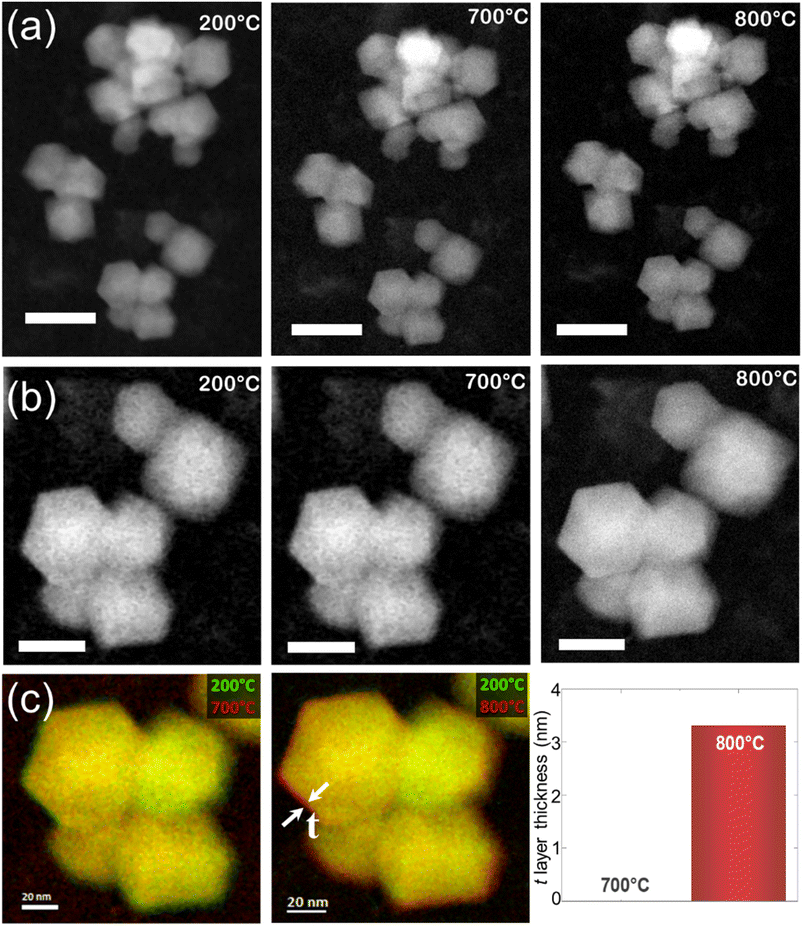 |
| Fig. 2 (a and b) High-angle annular dark-field scanning transmission electron microscopy (HAADF-STEM) analysis of the nanosized RHO submitted to thermal treatment between 200 and 800 °C at different magnifications. Scale bars of 50 and 20 nm for the first and second rows, respectively. (c) Superimposed micrographs acquired at different temperatures show the volume expansion of the region highlighted by red color and denoted by t (adopted with permission from ref. 32, copyright 2023 American Chemical Society). | |
An expansion of the lattice parameter does not always happen when the temperature increases. In some zeolitic frameworks, a contraction of lattice parameters is observed upon the increase of temperature which is called negative thermal expansion.47–51 This phenomenon is only observed in some metal oxides, metal cyanides, polymers, and zeolites. In zeolites which show negative thermal expansion (e.g. CHA, LTA, and FAU) upon increasing the temperature, the whole tetrahedra SiO4 units rotate inside the zeolite structure reducing the unit cell volume.19,47,52–54
The temperature-induced zeolite flexibility is not limited to contractions or expansions of the zeolite lattice; changes within the structure, such as cation movements, are also triggered by temperature variations.39,40,55,56 For example, when cadmium-exchanged zeolite RHO (Cd-RHO) is heated up to 300 °C, the pore-blocking Cd2+ cations relocate from the double eight-ring to the six-ring site, which are 5.7 Å apart.39 This phenomenon was also reported for Ba2+ and Sr2+ cations, which migrate from the single to the double eight-rings of RHO.40 However, for Cd-RHO it was later found that the migration of the cation was associated with the removal of water upon increasing the temperature.57 Another well-known example of cationic movements triggered by temperature is the potassium-exchanged CHA zeolite (K-CHA).10 Depending on the temperature, it is possible for the pores of K-CHA to permit access to certain guest molecules due to their interactions with the K+ cations; the movement of the K+ cations is described as a temporary and reversible displacement. Upon decreasing the temperature the K+ cations go back to their original position in the middle of CHA main pores (eight-membered rings), blocking the access of guest molecules.10 This behavior is very important for gas storage applications and was observed for different zeolitic frameworks.55,56,58Fig. 3 illustrates the effects of temperature and pressure-induced flexibility on zeolites.
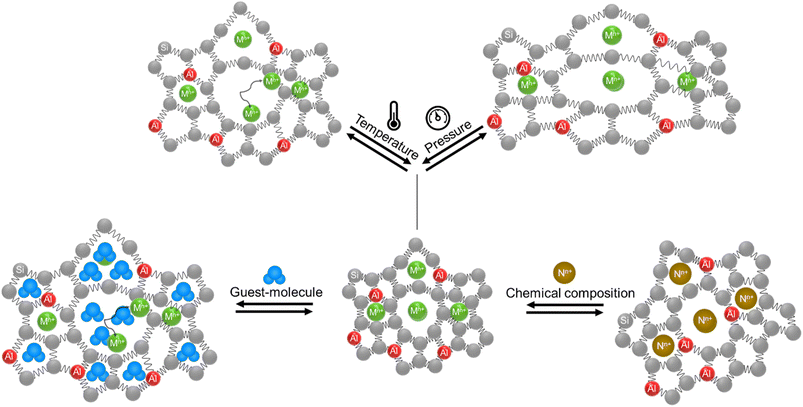 |
| Fig. 3 Schematic illustration of temperature and pressure-induced, guest-induced, and compositionally-induced flexibility in zeolites (Mn+ and Nn+ are two arbitrary cations assuming smaller cationic diameter for Mn+ compared to Nn+). | |
1.1.2. Pressure-induced flexibility.
Zeolites undergo alterations in their lattice parameters when subjected to pressure. These alterations are reversible until they reach a maximum pressure, commonly referred to as the “threshold pressure” in the literature.11,33–35 Beyond the threshold pressure, an irreversible change in the zeolite topology occurs reaching amorphization at high pressures.11,33–35,59 The threshold pressure limit is a distinct quality of zeolite frameworks.11,33,59 The pressure-induced flexibility observed in zeolites is mainly associated with movements of the rigid tetrahedra around the shared O atoms that behave like hinges within the framework.60 The channel content, i.e. adsorbed gasses (H2O, CO2, etc.) or extra-framework cations, govern the compressibility of the cavities, leading to different degrees of unit cell volume changes.60 For instance, sodium-exchanged LTA (Na-LTA) and natural yugawaralite zeolites can exhibit 18.4% and 15% reversible volume changes, respectively, in a non-intrusive medium at a pressure of up to 10 GPa.61 All zeolites related to the ANA framework family with initial space groups of Ia
d, I41/a, Ia
d, and I2/a for analcime,62 leucite,63 pollucite,64 and wairakite,65 respectively change and converge their symmetry to triclinic (P
) under pressures as low as 1.08 GPa followed by a reduction of their average Si–O–T (T = Al or Si) angle from 150 to 123°.33,66–68 Similar behavior was observed for NAT-type zeolite with a reduction of its Si–O–T angle from 133 to 119° under 8.5 GPa.69–71 CHA and MFI zeolites also show 10% and 16% volume variations under pressures of up to 8 GPa, respectively.11,33 KFI (ZK-5 type zeolite), RHO and SOD frameworks change their space group symmetry from Im
m to I4/mmm, I
3m, and I
3m respectively, under pressure (Fig. 3).26,42,59,72–76
Both temperature and pressure variations change the energy level of the zeolite structure thermodynamically (ΔG, Gibbs free energy), hence, there is always an energy barrier to see the aforementioned structural flexibilities. Temperature changes vary the entropic term of Gibbs free energy (ΔG = ΔH − TΔS). However, pressure changes are directly correlated with the Gibbs free energy and unit cell volume as well (ΔG = VΔP). As a result, these structural flexibilities categorized as temperature and pressure-induced flexibility are all of a thermodynamic nature and the necessary energy barriers for these structural changes can be estimated via thermodynamic simulations. More studies are needed to determine these energies.
1.2. Guest-induced flexibility
Zeolite flexibility can also be observed as a response to guest molecule adsorption or desorption. This can manifest as changes in the zeolite lattice parameters (framework dynamics) or by the relocation of extra-framework cations within zeolite pores (extra-framework dynamics).9,77,78 Guest-induced flexibility is highly interesting for gas separation and storage applications.
1.2.1. Framework dynamics.
The first attempts to describe guest-induced flexibility in zeolites were done using molecular dynamics simulations.79–82 Rigid zeolitic force fields were dynamically modified using transition state theory to include lattice flexibility, and the results obtained by these models were in good agreement with experimental data.79–81,83,84 It was shown that framework flexibility in silicalite-1 (MFI-type framework) is responsible for the adsorption of large molecules such as isobutane and heptane with inflection behavior at high loadings,80 while enhanced self-diffusivity at low loadings of short alkanes (i.e. methane and n-butane) was observed.81 Framework flexibility is also crucial for aromatics adsorption on MFI zeolite, especially at high pressures.84,85 While the use of rigid zeolitic models led to the underestimation of the aromatics adsorption in MFI zeolite by a factor of two,84,85 the use of flexible models to simulate diffusion coefficients of various alkanes in silicalite-1 zeolite resulted in a convergence of experimental and theoretical values.86 Recently by using in situ integrated differential phase contrast scanning transmission electron microscopy (iDPC-STEM) while adsorbing benzene molecules on MFI zeolite, Xiong et al. showed that the MFI structure goes through severe deformations giving another proof of guest-induced flexibility in zeolites (see Fig. 4).87
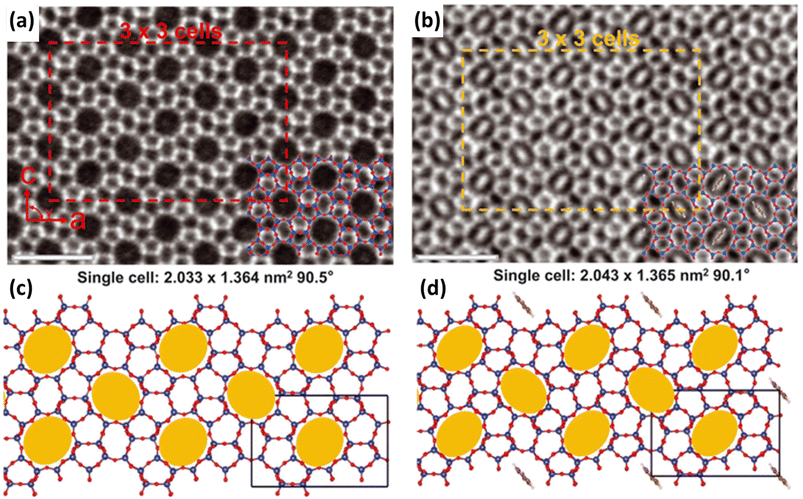 |
| Fig. 4 Integrated differential phase contrast scanning transmission electron microscopy (iDPC-STEM) images of MFI straight channels (a) before and (b) after benzene adsorption. MFI structure flexibility is also schematically highlighted (c) before and (d) after benzene adsorption. Scale bar, 500 pm (reprinted with permission from ref. 87, copyright 2022 The American Association for the Advancement of Science). | |
For the diffusion of small gas molecules such as methane through AFI and LTL pores, the diffusion coefficients were calculated and measured at high loadings.79 Calculations of methane adsorption in LTA zeolites showed that using flexible zeolitic models largely influences the diffusion coefficients and it is dependent on the type of extra-framework cations, the loading of methane in the structure, and the force field parameter used for simulations.88 Small-pore ITQ-55 zeolite with a minimal pore aperture diameter of 2.4 Å showed expansion of this minimal aperture to 3.1 Å, while allowing for ethylene to be adsorbed. This framework flexibility was used for separation of ethylene from ethane with a selectivity enhanced by a factor of ∼100.89Fig. 3 illustrates the guest-induced flexibility in zeolites.
Water adsorption on Na-LTA zeolite showed a phase transition with a small contraction followed by an expansion of the LTA framework, suggesting a hydration-driven flexibility transition, with a two-phase region separating hydrated zeolite A from its dehydrated form.90 Moreover, it was also observed that preferential water adsorption sites are within the beta cages of zeolite A.91,92 Thus, it was suggested that the diffusion of water molecules can only be simulated when flexible models are employed.91 Furthermore, the S-shaped water adsorption isotherms observed in LTA zeolite resemble those found in another significant category of porous materials: metal–organic frameworks (MOFs), known for their remarkable flexibility.93,94 This type of S-shaped adsorption behavior in MOFs is linked to pore expansion induced by adsorbates, which is comparable to the outcomes observed in various zeolites like LTA.90,95 These results suggest that zeolites, to varying degrees, may exhibit similar flexibility behavior to MOFs in the presence of guest molecules.
Na+ form of zeolite PAU, up to 5% unit cell shrinkage was observed upon dehydration.96 Water adsorption in silicalite-1 showed a transition from monoclinic to orthorhombic and this behavior was successfully modeled using flexible framework parameters during molecular dynamics simulations.97 Similar behavior was recorded for both water and CO2 adsorption on Na+ and Rb+ forms of gismondine (Na-GIS and Rb-GIS) where the ellipticity of the empty pores is reduced significantly by CO2 and water adsorption to a circular pore shape with up to 14% expansion of the unit cell volume.98 Ni-exchanged zeolite Y (FAU framework type) undergoes a series of structural rearrangements during dehydration from 20 to 400 °C. The Ni2+ cations start migrating towards tightly confined sites when water molecules desorb, thus a unit cell contraction happens with a strong deformation of the hexagonal prism of the FAU framework.99 Similar behavior was also observed for the Na–Y sample.100 Dehydration of zeolites Na-MER, K-MER, and Cs-MER also results in 10, 8, and 7% shrinkage of their unit cell volumes, respectively.101 All these studies suggested a zeolite framework flexibility in the presence of guest molecules. The most important flexibility observed was the 8% variation of the unit cell volume of RHO zeolite by dehydration, a completely reversible process after adsorption of H2O or CO2 molecules (Fig. 5).9,43,102–106
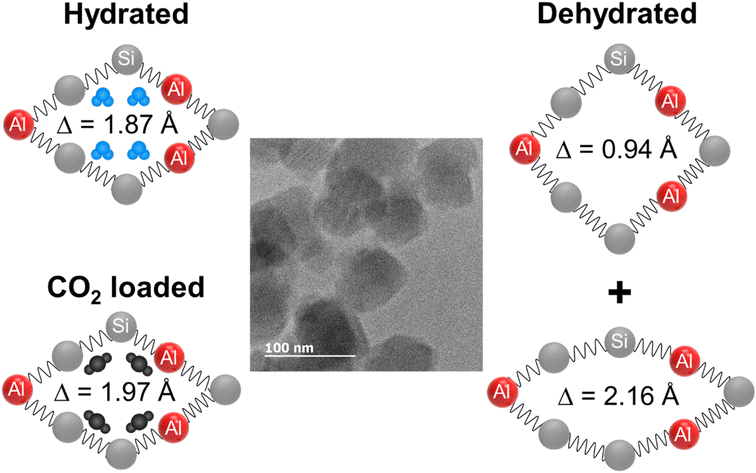 |
| Fig. 5 Schematic illustration of reversible ellipticity of RHO nanosized zeolites upon dehydration and CO2 adsorption (adopted with permission from ref. 9, copyright 2020 American Chemical Society). | |
1.2.2. Extra-framework dynamics.
Similar to the cation relocations within the zeolite framework triggered by temperature (vide supra), it has been shown in the literature that sorption of guest molecules can also trigger such cation movements.9,77,107 The migration of Fe3+ cations inside the FAU cages after dehydration was confirmed by electron spin resonance spectroscopy.108 Similarly, the migration of La3+ cations from the supercages of FAU to small sodalite cages was observed during dehydration.109 In Li+ and Mg2+ forms of ZK-5 zeolite (KFI framework type), the migration of these cations from the center of the hexagonal prisms of their structure to the α-cages was observed after CO2 adsorption.110 These cation relocations are known as “cation gating” (also known as “trapdoor behavior”), and they were proposed simultaneously by P. A. Wright and P. Webley and co-workers in 2012.77,111 It was shown that the gating movement of cations is responsible for opening or closing the pore access depending on the nature of guest molecules.77,111,112 In Na-RHO, migration of the Na+ cations was described and CO2 molecules could pass through the pores between the α-cages while Na+ cations migrate from the single eight-membered rings (see Fig. 6a).111 In K-CHA, however, K+ cations reject or admit the guest molecules based on their attraction and repulsion towards them and the movement of K+ cations is completely reversible from and to the single eight-membered rings (see Fig. 6b).6 Based on DFT calculations, door-keeper cations have to pay an energy penalty to move away from the center of the pore aperture (thus allows gas admission) in typical trapdoor zeolites such as Cs-CHA, Na-RHO, etc.78,104,113 The difficulty of this pore opening process is reflected by the energy barrier associated with the cation movement path from its most stable position (where it blocks the entrance of the pore aperture) to the second most stable position inside the zeolite (where the pore aperture is open).113 The energy difference between these two configurations can qualitatively feature the actual energy barrier. The presence of polarizable gas molecules that possess some sort of dipole or quadrupole interactions with the door-keeper cations such as CO2 and CO can substantially lower the energy difference, whereas those non-polar molecules such as N2, CH4, and H2 can hardly change the energy difference.113 Hence, the polarizable guest molecules (e.g. CO2) induce the door-keeper cations to move out from the center of the pores of the zeolite and selectively admit CO2 to the CHA or RHO structure while rejecting nonpolar molecules such as N2 and CH4.3,10,77,112–115 Similar behavior was observed also for other zeolitic types such as MER and PAU frameworks.96,101 Another interpretation of the “cation gating” phenomenon, namely the “swinging door” mechanism focuses on the thermal motions of door-keeper cations rather than their movement.116 Based on this interpretation, the amplitude of the thermal motion of the door-keeper cations is always large and thus CO2 can squeeze in while the gate is swinging because of the stronger attraction to the framework (quadrupole interactions of CO2 and the cations), while nonpolar guests such as methane cannot.116 The consequence of the “cation gating” phenomenon, regardless of which interpretation (“trapdoor behavior” or “swinging door” mechanism) used, is superior selectivity for separation of CO2 from CH4 or N2 (CO2/CH4 up to 583 and CO2/N2 up to 688 for K-CHA with Si/Al = 1.9).117 In summary, a large opportunity is available to design materials for gas separation applications already suggested by many studies.3,10,77,112–115,118Fig. 6 shows a schematic illustration of the trapdoor behavior in Cs-CHA proposed by Shang et al.77
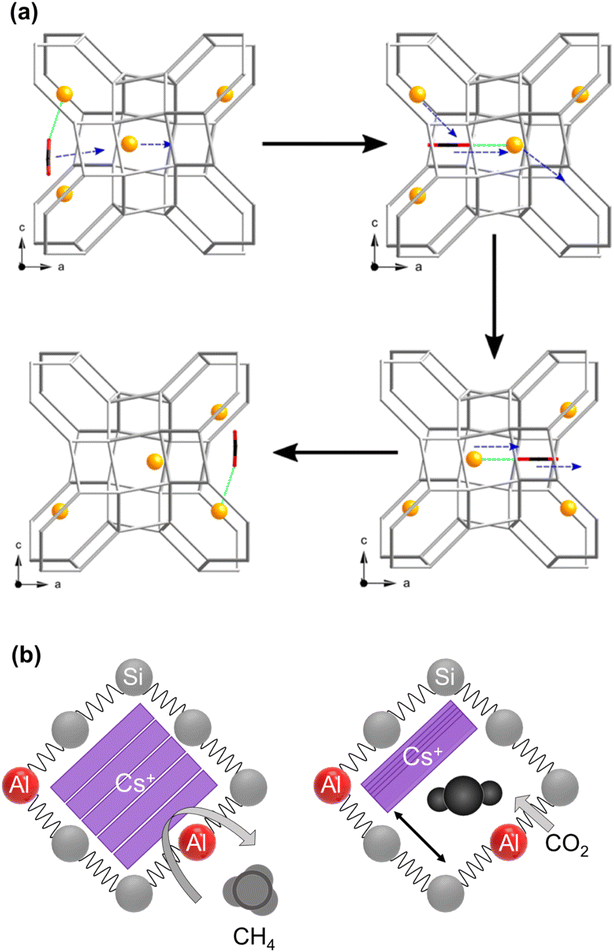 |
| Fig. 6 Schematic illustration of cation gating (a) permanent relocation of Na+ cations, this is a cooperative mechanism by which CO2 molecules could pass through a window site between α-cages in zeolite Na-Rho proposed by Lozinska et al. (reprinted with permission from ref. 111, copyright 2012 American Chemical Society). (b) Reversible Cs+ relocations namely trapdoor behavior in Cs-CHA proposed by Shang et al.77 (adopted with permission from ref. 77, copyright 2012 American Chemical Society). | |
1.3. Compositionally-induced flexibility
Compositional changes in zeolites were also shown to play an important role in framework flexibility. Substitution of Si atoms with Al (framework composition) and the type and content of extra-framework cations inside the zeolite structure (extra-framework composition) can also induce significant changes in the zeolite lattice parameters. The ability to engineer the pore diameter and pore shape of zeolites is another important aspect of zeolite flexibility especially for gas separation applications. A schematic illustration of compositionally-induced flexibility in zeolites is presented in Fig. 3.
1.3.1. Framework composition.
Based on computational studies, it was found that the flexibility of LTA zeolite also depends heavily on the Al content.119 Higher Al amounts result in longer Al–O bond lengths and more flexibility of the Si–O–Al bridging angles which creates a more open and more flexible framework.119 In our group, we recently showed for a series of RHO zeolites with different Si/Al ratios and lattice parameters, that changes to the Al distribution within the nanosized RHO samples, represented by the shift in the 29Si NMR barycenter, could be correlated with the CO2 adsorption capacity.120 Changing the Si/Al ratio of RHO from 1.5 to 1.7 resulted in an increase of the CO2 capacity from 1.37 to 2.01 mmol g−1.120 This is due to changes in the framework charge distribution, which play a fundamental role in controlling the CO2 adsorption capacity and should not be overlooked as the cation distribution will always be guided by the negative charge distribution within the framework.120
1.3.2. Extra-framework composition.
Another important factor affecting zeolite flexibility is the type and content of the extra-framework cations. The calcium-exchanged RHO zeolite is an example: the partial exchange of deuteron cations with calcium cations resulted in a 21% decrease in the zeolite's unit cell volume.121 Depending on the cationic composition, RHO zeolites have shown significantly different unit cell volumes, pore diameters, and pore shapes (see Fig. 7).73,102,122 For example, the proton form of RHO (H-RHO) shows a circular pore with a size of 3.9 Å, while a lithium form shows an elliptical pore with a size of 1.9 Å (Fig. 7a);however Si/Al ratios of these RHO samples are the same.5
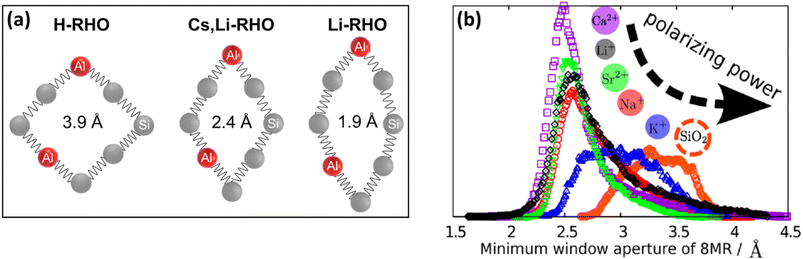 |
| Fig. 7 Ellipticity of zeolite RHO containing different cations (a) based on experiments and (b) based on simulations (adopted with permission from ref. 5 and 123, copyright 2015 and 2016 American Chemical Society). | |
Similarly to RHO, different alkali-metal (Li+, Na+, K+, Rb+, and Cs+)124,125 and alkaline earth metal (Mg2+, Ca2+, Sr2+, and Ba2+)126 forms of zeolites PST-3 and PST-4 (NAT framework) show significantly different pore shapes and sizes with unit cell volumes ranging from ∼2120 Å3 (for Li-form of natural NAT) to ∼2680 Å3 (for Cs-PST-4). Using different alkali-metal cations, it is possible to tune the pore opening of different GIS samples with unit cell volumes of 877, 853, and 851 Å3 for Na-GIS, K-GIS, and Rb-GIS, respectively.98 Similarly, the unit cell volume of different alkali-metal forms of MER zeolite can be tuned to 1809, 1823, and 1881 Å3 for Na-MER, K-MER, and Cs-MER, respectively.101 These changes are due to the tetrahedral tilts and changes in T–O–T bonds and angles (T = Si or Al). The extent of these changes is influenced by the polarizing power of the extra-framework cations and their interaction with the negative charge of the framework due to the presence of Al (Fig. 7b).5
2. Evaluation of the framework flexibility of zeolites
A major effort was made by Sartbaeva and co-workers to identify and explain flexibility in zeolites.127 They have analyzed the geometry of the structural polyhedra in 14 pure silica zeolite structures determining a flexibility window explained by the coulombic inflation: a repulsion between close oxygen atoms that appeared to play a role in stabilizing the open frameworks of zeolites when dehydrated.127,128 The flexibility index is defined as the ratio of the maximum over the minimum feasible framework densities for a particular framework type (ρmax/ρmin).128,129 It has been tabulated for most of the known zeolite framework types but remains a limited descriptor of actual framework flexibility because most framework types can be folded along multiple paths starting from the maximum symmetry point, which occurs very often at the minimum framework density.129 Furthermore, real zeolite materials tend to occupy the low-density end of the flexibility window, so flexibility behavior at higher densities may not play a key role in determining flexibility.129 In addition, to compare the flexibility of different zeolitic frameworks with each other (both pure silica and aluminosilicates), and perhaps to other porous materials like metal organic frameworks (MOFs), a quantitative parameter is missing in the literature. To illustrate that, Fig. 8 shows the maximum changes in the unit cell volume observed for the most flexible structures found in the literature. Based on Fig. 8, the changes in the unit cell volume upon different flexibility triggers can be as significant as 20%, which once more proves the importance of understanding flexibility as a way to design smart materials for selective separation or catalysis in porous materials. However, standard protocols are missing in the literature to be able to quantify flexibility in zeolites and compare them with other porous materials. Perhaps, performing in situ powder X-ray diffraction (XRD) measurements at different temperatures would be sufficient to explore the temperature-induced flexibility of different zeolites. XRD patterns of dehydrated forms of different zeolites with variable compositions (mainly their Si/Al ratios and extra-framework cationic contents) could be used to study compositionally-induced flexibility. However, to study the guest-induced flexibility, the immediate challenge is whether the probe molecule is a non-polarizable molecule such as N2 or Ar or a polarizable one such as CO2. If a non-polarizable probe molecule is selected, some parts of guest-induced flexibility related to the cation gating effect will be hindered. On the other hand, the selection of polarizable probe molecules may interfere with compositionally-induced flexibility due to the dynamics of cations inside the zeolite structure. Additional work is required to establish a protocol for the experimental evaluation of zeolite flexibility. This is of great importance as it will substantially help in designing smart materials for selective gas separation or catalytic applications.
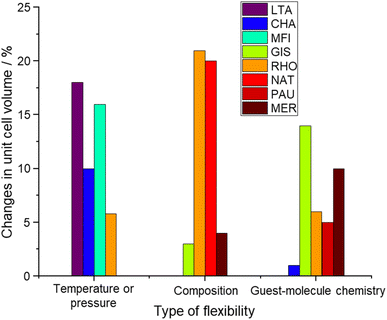 |
| Fig. 8 Maximum changes in the unit cell volume of the most flexible zeolite structures presented in the literature.5,33,37,41–43,46,61,96,98,101,122,125,126 | |
2.1. Challenges in the characterization of flexibility
To establish rigid protocols for the evaluation of zeolite flexibility using different in situ spectroscopic techniques can be considered as a solution to probe zeolites' atomic order. Temperature-induced flexibility can be assessed using in situ XRD measurements at different temperatures (e.g. from 25 to 800 °C) followed by lattice parameter evaluations by Le Bail and Rietveld refinement. Similar procedures have been reported for RHO zeolite.43 Pressure-induced flexibility can be measured by in situ XRD when applying a non-intrusive pressure (e.g. up to 15 GPa); this has also been reported for several zeolites including LTA, ANA, etc.33,61–63,65
The biggest challenge is to quantify guest-induced flexibility. The guest-induced flexibility can manifest both in the form of changes in zeolites' lattice parameters or by a dynamic movement of extra-framework cations within the zeolite structure. Both these phenomena often happen simultaneously and they are intertwined. As a result, their quantification is not a trivial task. To tackle this issue, the use of in situ spectroscopic techniques can be considered. In situ XRD measurements can be considered while different gas molecules are adsorbed on the zeolite samples. Conterosito and co-workers reported CO2 and Xe adsorption in FAU zeolite followed by in situ XRD thus enabling estimation of the lattice parameters and location of extra-framework cations during adsorption and desorption processes.130 The in situ XRD under adsorption of gases can be coupled with DFT calculations and machine learning approaches in order to provide information concerning the necessary energies for extra-framework cation relocations. Additionally, in situ solid-state NMR while adsorbing guest molecules can be of importance to clarify the atomic order that contributes to the guest-induced flexibility.131 Recently, Ilkaeva and co-workers developed a method to follow CO2 adsorption using in situ solid-state NMR which shed light on different CO2 chemisorbed species on SBA-15.132In situ FTIR while adsorbing guest molecules also illustrates the atomic orders especially those of the silanol sites.131,133 As a result, combining all these in situ techniques (i.e. XRD, NMR, and FTIR), while adsorbing guest molecules, can help to solve the intertwined dynamics between the zeolite framework and extra-framework cations.
For the compositionally-induced flexibility due to the framework's T-site composition, we propose to consider lattice parameters of all silica zeolites of any particular framework as the reference in the future. Thus, the flexibility can be compared to all silica zeolites when Al is replacing the Si and the Si/Al ratio varies. The use of NMR spectroscopy may give insights into the distortion of the framework due to the inclusion of Al tetrahedra by monitoring changes in the quadrupolar coupling constants of 27Al and 17O nuclei but also the chemical shifts of 29Si nuclei. For the compositionally-induced flexibility due to the extra-framework cation compositions, the proton form (H-form) of zeolites can be considered as a reference and different cationic forms can be compared to the proton form of zeolites at a constant Si/Al ratio. The framework distortions can be examined by conducting Rietveld refinement of the XRD patterns and by monitoring the framework's vibrational bands through Raman spectroscopy. This terminology was used to estimate the changes in unit cell volume of several zeolites (GIS, RHO, NAT, and MER) presented in Fig. 8. Significantly more detailed studies are needed to explore zeolite flexibility; however, understanding flexibility in zeolites can open a new avenue for zeolite utilizations.
2.2. Emerging applications related to the flexibility of zeolites
The concept of inducing and controlling flexibility in zeolites can be utilized in different applications. The most prominent one is the bulk separation and purification of small gas molecules such as gas drying (H2O removal), CO2 separation from N2 in flue gas, CO2 separation from CH4 in landfill gas separation or bio-methane upgrading, CO2 separation in dilute streams for direct air capture, etc. The main advantage of using flexible zeolites for gas separation is the ability to fine-tune the zeolite pore aperture (size and shape) due to compositionally-induced flexibility by using various extra-framework cations. This has been illustrated for zeolite RHO.5 In addition, thanks to guest-induced flexibility (extra-framework dynamics – cation gating phenomenon), superior selectivity was observed towards any non-neutral gas molecules such as H2O (polar) and CO2 (polarizable molecule – quadrupole moments).3,77,78 These separations are important since enormous efforts have been dedicated to developing materials for carbon capture and storage since the rising concentration of CO2 is contributing to the current anthropogenic global climate change.
In addition, gas storage of CH4, CO2, O2, H2, etc. is of significant importance. Storage of these small gas molecules is extremely hard and can be achieved only at very high-pressures and low-temperatures. Thanks to guest-induced flexibility (extra-framework dynamics), it is possible to selectively admit and store specific gas species at pressures and temperatures near ambient conditions. One example is the storage of CH4 and H2 in CHA zeolite as reported by Li et al.10 The additional advantage of using flexible zeolite for gas storage is the controlled release of the guest molecules under ambient conditions (e.g. CH4) which is much safer compared to the traditional gas storage inside high-pressure vessels (usually between 50 and 200 bars); the encapsulation and release of CH4 gas molecules inside K-CHA zeolite were reported as well by Li et al.10 Another example of controlled guest molecule release is in biological applications where FAU zeolites were used to deliver O2 as well as other necessary drugs to cancer cells.134,135
Finally, flexible zeolites are also suitable candidates for sensing applications as it was reported before.136–138 Wales et al. demonstrated that zeolites hold great promise for various sensing applications, as discussed in their work.139 In this prospective review, we have highlighted zeolites such as FAU, MFI, MOR, and others, showcasing their remarkable flexibility.139 This underscores the idea that understanding various aspects of zeolite flexibility can also contribute to sensing applications. Another important class of porous materials used as gas adsorbents or sensors, which exhibit structural flexibility, is MOFs.140–143 However, zeolites offer advantages in terms of greater chemical and thermal stability (some zeolites can withstand temperatures up to 1200 °C compared to 500 °C for MOF-related zeolitic imidazolates139), environmentally-friendly synthesis methods, and ease of integration into smaller devices such as sensors. This perspective is further supported by the work of Wales et al., who reviewed zeolitic and MOF-based sensors for automotive applications and found that approximately 62% of these sensors were zeolite-based, while 38% were MOF-based.139
Zeolites can no longer be considered exclusively as rigid materials. Based on calculations, all zeolitic frameworks can theoretically show different degrees of flexibility.26–28 Flexibility in zeolites can be defined as a reversible framework deformation (expansion/contraction) or a dynamic of extra-framework cations, an intrinsic property of zeolitic frameworks due to the spring-like behavior of Si–O and Al–O bonds and the distortion of Si–O–Al and Si–O–Si angles as a response to an external trigger such as gas adsorption/desorption or a change in temperature or pressure. Based on these different triggers, we categorized zeolite flexibility into three categories: (1) temperature and pressure-induced flexibility, (2) guest-induced flexibility, and (3) compositionally-induced flexibility. Evaluation of zeolite flexibility is another challenge which can be solved by combining in situ spectroscopic techniques (XRD, FTIR, and NMR), gas adsorption measurements, high resolution microscopy, DFT calculations, and machine learning techniques. Understanding and quantifying zeolite flexibility can open a new avenue for applications such as gas separation, gas storage, drug delivery, sensing applications, etc.
3. Conclusions and outlook
In this perspective article, the origin of zeolite flexibility is revealed based on the understanding of spring-like features of Si–O and Al–O bonds and the distortion of Si–O–Al and Si–O–Si angles as a response to an external trigger. Different aspects of zeolites' flexibility were presented including the main triggers of zeolite flexibility identified as temperature and pressure, guest-molecules, and composition variations. By tuning the temperature and zeolite composition, one can tune the size and shape of zeolite pores. Guest molecule chemistry can also be used to design smart zeolites to capture/separate targeted molecules. The zeolite flexibility can be either at the unit cell level (changes in the lattice parameters) or at the atomic level (relocation of extra-framework cations within the zeolite). Further studies are necessary to develop experimental methods to quantify, normalize and compare the flexibility of different zeolite frameworks and perhaps for other porous materials. By gaining a deep understanding of zeolite flexibility, we can manipulate and control zeolite adsorption and separation properties. Moreover, this newfound ability opens up exciting possibilities for diverse applications, such as drug delivery and sensing. In drug delivery, the flexible nature of zeolites can be exploited to design innovative carriers that respond to specific stimuli, releasing therapeutic agents precisely when and where needed. Similarly, in sensing applications, zeolite flexibility can be leveraged to create advanced sensor materials with improved sensitivity and selectivity. These sensors can detect and quantify various substances, ranging from gases and liquids to biomolecules, enabling their application in environmental monitoring, healthcare, and other industries. Overall, the growing understanding of zeolite flexibility opens up a vast array of emerging applications in diverse fields.
Author contributions
S. G., E. D., and S. M. have conceptualized the paper and the figures. S. G. has written the first draft. Critical inputs were added by E. D and S. M. after revising the manuscript. All authors contributed to the final revisions of the paper.
Conflicts of interest
There are no conflicts to declare.
Acknowledgements
The authors wish to thank Dr Edwin Clatworthy for his helpful discussions. This work was co-funded by the European Union (ERC, ZEOLIghT, 101054004). Views and opinions expressed are however those of the author(s) only and do not necessarily reflect those of the European Union or the European Research Council. Neither the European Union nor the granting authority can be held responsible for them. The support of the Centre for Zeolites and Nanoporous Materials, Label of Excellence, Normandy Region (CLEAR) is acknowledged.
References
- S. Mintova, M. Jaber and V. Valtchev, Nanosized Microporous Crystals: Emerging Applications, Chem. Soc. Rev., 2015, 44(20), 7207–7233, 10.1039/C5CS00210A.
- S. Mintova, J. Grand and V. Valtchev, Nanosized Zeolites: Quo Vadis?, C. R. Chim., 2016, 19(1), 183–191, DOI:10.1016/j.crci.2015.11.005.
- S. Ghojavand, B. Coasne, E. B. Clatworthy, R. Guillet-Nicolas, P. Bazin, M. Desmurs, L. Jacobo Aguilera, V. Ruaux and S. Mintova, Alkali Metal Cations Influence the CO2 Adsorption Capacity of Nanosized Chabazite: Modeling vs. Experiment, ACS Appl. Nano Mater., 2022, 5(4), 5578–5588, DOI:10.1021/acsanm.2c00537.
- K. Chen, S. H. Mousavi, R. Singh, R. Q. Snurr, G. Li and P. A. Webley, Gating Effect for Gas Adsorption in Microporous Materials—Mechanisms and Applications, Chem. Soc. Rev., 2022, 51(3), 1139–1166, 10.1039/D1CS00822F.
- M. M. Lozinska, E. Mangano, A. G. Greenaway, R. Fletcher, S. P. Thompson, C. A. Murray, S. Brandani and P. A. Wright, Cation Control of Molecular Sieving by Flexible Li-Containing Zeolite Rho, J. Phys. Chem. C, 2016, 120(35), 19652–19662, DOI:10.1021/acs.jpcc.6b04837.
- J. Shang, G. Li, R. Singh, P. Xiao, J. Z. Liu and P. A. Webley, Determination of Composition Range for “Molecular Trapdoor” Effect in Chabazite Zeolite, J. Phys. Chem. C, 2013, 117(24), 12841–12847, DOI:10.1021/jp4015146.
-
E. N. Coker, Ion Exchange Equilibria and Kinetics in Zeolites: Influences of Framework Flexibility and Charge Density* *Dedicated to the Memories of Richard M. Barrer (1910 – 1996) and Lovat V.C. Rees (1927 – 2006), in Studies in Surface Science and Catalysis, ed. R. Xu, Z. Gao, J. Chen and W. Yan, From Zeolites to Porous MOF Materials – The 40th Anniversary of International Zeolite Conference, Elsevier, 2007, vol. 170, pp. 110–120, DOI:10.1016/S0167-2991(07)80829-9.
- B. Ilić and S. G. Wettstein, A Review of Adsorbate and Temperature-Induced Zeolite Framework Flexibility, Microporous Mesoporous Mater., 2017, 239, 221–234, DOI:10.1016/j.micromeso.2016.10.005.
- J. Grand, N. Barrier, M. Debost, E. B. Clatworthy, F. Laine, P. Boullay, N. Nesterenko, J.-P. Dath, J.-P. Gilson and S. Mintova, Flexible Template-Free RHO Nanosized Zeolite for Selective CO2 Adsorption, Chem. Mater., 2020, 32(14), 5985–5993, DOI:10.1021/acs.chemmater.0c01016.
- G. Li, J. Shang, Q. Gu, R. V. Awati, N. Jensen, A. Grant, X. Zhang, D. S. Sholl, J. Z. Liu, P. A. Webley and E. F. May, Temperature-Regulated Guest Admission and Release in Microporous Materials, Nat. Commun., 2017, 8(1), 1–9, DOI:10.1038/ncomms15777.
- L. Leardini, S. Quartieri and G. Vezzalini, Compressibility of Microporous Materials with CHA Topology: 1. Natural Chabazite and SAPO-34, Microporous Mesoporous Mater., 2010, 127(3), 219–227, DOI:10.1016/j.micromeso.2009.07.017.
- P. Judeinstein, M. Zeghal, D. Constantin, C. Iojoiu and B. Coasne, Interplay of Structure and Dynamics in Lithium/Ionic Liquid Electrolytes: Experiment and Molecular Simulation, J. Phys. Chem. B, 2021, 125(6), 1618–1631, DOI:10.1021/acs.jpcb.0c09597.
-
D. Frenkel and B. Smit, Understanding Molecular Simulation: From Algorithms to Applications, Elsevier, 2001 Search PubMed.
- B. Liu and B. Smit, Molecular Simulation Studies of Separation of CO2/N2, CO2/CH4, and CH4/N2 by ZIFs, J. Phys. Chem. C, 2010, 114(18), 8515–8522, DOI:10.1021/jp101531m.
- S. Jost, P. Biswas, A. Schüring, J. Kärger, P. A. Bopp, R. Haberlandt and S. Fritzsche, Structure and Self-Diffusion of Water Molecules in Chabazite: A Molecular Dynamics Study, J. Phys. Chem. C, 2007, 111(40), 14707–14712, DOI:10.1021/jp073857s.
- S. E. Jee and D. S. Sholl, Carbon Dioxide and Methane Transport in DDR Zeolite: Insights from Molecular Simulations into Carbon Dioxide Separations in Small Pore Zeolites, J. Am. Chem. Soc., 2009, 131(22), 7896–7904, DOI:10.1021/ja901483e.
- A. Gabrieli, M. Sant, P. Demontis and G. B. Suffritti, Development and Optimization of a New Force Field for Flexible Aluminosilicates, Enabling Fast Molecular Dynamics Simulations on Parallel Architectures, J. Phys. Chem. C, 2013, 117(1), 503–509, DOI:10.1021/jp311411b.
- G. Schrimpf, M. Schlenkrich, J. Brickmann and P. Bopp, Molecular Dynamics Simulation of Zeolite NaY: A Study of Structure, Dynamics, and Thermalization of Sorbates, J. Phys. Chem., 1992, 96(18), 7404–7410, DOI:10.1021/j100197a050.
- K. D. Hammonds, V. Heine and M. T. Dove, Rigid-Unit Modes and the Quantitative Determination of the Flexibility Possessed by Zeolite Frameworks, J. Phys. Chem. B, 1998, 102(10), 1759–1767, DOI:10.1021/jp980006z.
- P. Demontis, J. Gulín-González, M. Masia and G. B. Suffritti, The Behaviour of Water Confined in Zeolites: Molecular Dynamics Simulations versus Experiment, J. Phys.: Condens.Matter, 2010, 22(28), 284106, DOI:10.1088/0953-8984/22/28/284106.
- P. Demontis and G. B. Suffritti, A Comment on the Flexibility of Framework in Molecular Dynamics Simulations of Zeolites, Microporous Mesoporous Mater., 2009, 125(1), 160–168, DOI:10.1016/j.micromeso.2009.03.032.
- R. Krishna and J. M. van Baten, A Molecular Dynamics Investigation of the Diffusion Characteristics of Cavity-Type Zeolites with 8-Ring Windows, Microporous Mesoporous Mater., 2011, 137(1), 83–91, DOI:10.1016/j.micromeso.2010.08.026.
- A. F. Combariza, G. Sastre and A. Corma, Molecular Dynamics Simulations of the Diffusion of Small Chain Hydrocarbons in 8-Ring Zeolites, J. Phys. Chem. C, 2011, 115(4), 875–884, DOI:10.1021/jp102262n.
- M. Jeffroy, C. Nieto-Draghi and A. Boutin, Molecular Simulation of Zeolite Flexibility, Mol. Simul., 2014, 40(1–3), 6–15, DOI:10.1080/08927022.2013.840898.
- S. E. Boulfelfel, P. I. Ravikovitch, L. Koziol and D. S. Sholl, Improved Hill–Sauer Force Field for Accurate Description of Pores in 8-Ring Zeolites, J. Phys. Chem. C, 2016, 120(26), 14140–14148, DOI:10.1021/acs.jpcc.6b03674.
- V. Kapko, C. Dawson, M. M. J. Treacy and M. F. Thorpe, Flexibility of Ideal Zeolite Frameworks, Phys. Chem. Chem. Phys., 2010, 12(30), 8531–8541, 10.1039/C003977B.
- E. Verheyen, L. Joos, C. Martineau, C. J. Dawson, C. Weidenthaler, W. Schmidt, R. Yuan, E. Breynaert, V. V. Speybroeck, M. Waroquier, F. Taulelle, M. M. J. Treacy, J. A. Martens and C. E. A. Kirschhock, Flexibility versus Rigidity: What Determines the Stability of Zeolite Frameworks? A Case Study, Mater. Horiz., 2014, 1(6), 582–587, 10.1039/C4MH00127C.
- S. A. Wells, K. M. Leung, P. P. Edwards, M. G. Tucker and A. Sartbaeva, Defining the Flexibility Window in Ordered Aluminosilicate Zeolites, R. Soc. Open Sci., 2017, 4(9), 170757, DOI:10.1098/rsos.170757.
- V. Kapko, C. Dawson, I. Rivin and M. M. J. Treacy, Density of Mechanisms within the Flexibility Window of Zeolites, Phys. Rev. Lett., 2011, 107(16), 164304, DOI:10.1103/PhysRevLett.107.164304.
- R. E. Fletcher, S. A. Wells, K. M. Leung, P. P. Edwards and A. Sartbaeva, Intrinsic Flexibility of Porous Materials; Theory, Modelling and the Flexibility Window of the EMT Zeolite Framework, Acta Crystallogr., Sect. B: Struct. Sci., Cryst. Eng. Mater., 2015, 71(6), 641–647, DOI:10.1107/S2052520615018739.
- M. M. J. Treacy, C. J. Dawson, V. Kapko and I. Rivin, Flexibility Mechanisms in Ideal Zeolite Frameworks, Philos. Trans. R. Soc., A, 2014, 372(2008), 20120036, DOI:10.1098/rsta.2012.0036.
- E. B. Clatworthy, S. Moldovan, K. Nakouri, S. P. Gramatikov, F. Dalena, M. Daturi, P. St. Petkov, G. N. Vayssilov and S. Mintova, Visualizing the Flexibility of RHO Nanozeolite: Experiment and Modeling, J. Am. Chem. Soc., 2023, 145(28), 15313–15323, DOI:10.1021/jacs.3c02822.
- S. Quartieri, G. Montagna, R. Arletti and G. Vezzalini, Elastic Behavior of MFI-Type Zeolites: Compressibility of H-ZSM-5 in Penetrating and Non-Penetrating Media, J. Solid State Chem., 2011, 184(6), 1505–1516, DOI:10.1016/j.jssc.2011.04.026.
- G. D. Gatta, Extreme Deformation Mechanisms in Open-Framework Silicates at High-Pressure: Evidence of Anomalous Inter-Tetrahedral Angles, Microporous Mesoporous Mater., 2010, 128(1), 78–84, DOI:10.1016/j.micromeso.2009.08.006.
- A. Sartbaeva, J. Haines, O. Cambon, M. Santoro, F. Gorelli, C. Levelut, G. Garbarino and S. A. Wells, Flexibility Windows and Compression of Monoclinic and Orthorhombic Silicalites, Phys. Rev. B: Condens. Matter Mater. Phys., 2012, 85(6), 064109, DOI:10.1103/PhysRevB.85.064109.
- J. B. Parise, T. E. Gier, D. R. Corbin, L. Abrams, J. D. Jorgensen and E. Prince, Flexibility of the Framework of Zeolite Rho. Structural Variation from 11 to 573 K. A Study Using Neutron Powder Diffraction Data, J. Phys. Chem., 1984, 88(11), 2303–2307, DOI:10.1021/j150655a024.
-
W. H. Baur, R. X. Fischer and R. D. Shannon, Relations and Correlations in Zeolite RHO and Computer Simulations of Its Crystal Structure, in Studies in Surface Science and Catalysis, ed. P. J. Grobet, W. J. Mortier, E. F. Vansant and G. Schulz-Ekloff, Innovation in Zeolite Materials Science, Elsevier, 1988, vol. 37, pp. 281–292, DOI:10.1016/S0167-2991(09)60605-4.
- Wm. C. Conner, R. Vincent, P. Man and J. Fraissard, Flexibility in Zeolites:29Si NMR Studies of ZSM-5 Frame Transitions, Catal. Lett., 1990, 4(1), 75–83, DOI:10.1007/BF00764873.
- J. B. Parise, X. Liu and D. R. Corbin, Flexibility of the Zeolite RHO Framework; Relocation of Cadmium Accompanying Transformation of the Unit Cell at High Temperatures, J. Chem. Soc. Chem. Commun., 1991, 3, 162–163, 10.1039/C39910000162.
- J. B. Parise, X. Liu, D. R. Corbin and G. A. Jones, Flexibility Of The Zeolite Rho Framework: The Redistribution Of Extra Framework Cations As A Function Of Temperature, MRS Online Proc. Libr. Arch., 1991, 233, 267, DOI:10.1557/PROC-233-267.
- N. Khosrovani and A. W. Sleight, Flexibility of Network Structures, J. Solid State Chem., 1996, 121(1), 2–11, DOI:10.1006/jssc.1996.0002.
- S. R. G. Balestra, J. J. Gutiérrez-Sevillano, P. J. Merkling, D. Dubbeldam and S. Calero, Simulation Study of Structural Changes in Zeolite RHO, J. Phys. Chem. C, 2013, 117(22), 11592–11599, DOI:10.1021/jp4026283.
- E. B. Clatworthy, A. A. Paecklar, E. Dib, M. Debost, N. Barrier, P. Boullay, J.-P. Gilson, N. Nesterenko and S. Mintova, Engineering RHO Nanozeolite: Controlling the Particle Morphology, Al and Cation Content, Stability, and Flexibility, ACS Appl. Energy Mater., 2022, 5(5), 6032–6042, DOI:10.1021/acsaem.2c00439.
- R. M. Barrer and D. E. W. Vaugiian, Trapping of Inert Gases in Sodalite and Cancrinite Crystals, J. Phys. Chem. Solids, 1971, 32(3), 731–743, DOI:10.1016/S0022-3697(71)80413-4.
- D. I. Kopelevich and H.-C. Chang, Diffusion of Inert Gases in Silica Sodalite: Importance of Lattice Flexibility, J. Chem. Phys., 2001, 115(20), 9519–9527, DOI:10.1063/1.1414373.
- M. Debost, P. B. Klar, N. Barrier, E. B. Clatworthy, J. Grand, F. Laine, P. Brázda, L. Palatinus, N. Nesterenko, P. Boullay and S. Mintova, Synthesis of Discrete CHA Zeolite Nanocrystals without Organic Templates for Selective CO2 Capture, Angew. Chem., Int. Ed., 2020, 59(52), 23491–23495, DOI:10.1002/anie.202009397.
- W. Miller, C. W. Smith, D. S. Mackenzie and K. E. Evans, Negative Thermal Expansion: A Review, J. Mater. Sci., 2009, 44(20), 5441–5451, DOI:10.1007/s10853-009-3692-4.
-
S. H. Park, R.-W. Große Kunstleve, H. Graetsch and H. Gies, The Thermal Expansion of the Zeolites MFI, AFI, DOH, DDR, and MTN in Their Calcined and as Synthesized Forms, in Studies in Surface Science and Catalysis, ed. H. Chon, S.-K. Ihm and Y. S. Uh, Progress in Zeolite and Microporous Materials, Elsevier, 1997, vol. 105, pp. 1989–1994, DOI:10.1016/S0167-2991(97)80664-7.
- M. P. Attfield, Strong Negative Thermal Expansion in Siliceous Faujasite, Chem. Commun., 1998,(5), 601–602, 10.1039/A707141H.
- D. A. Woodcock, P. Lightfoot, P. A. Wright, L. A. Villaescusa and M. A. Camblor, Strong Negative Thermal Expansion in the Siliceous Zeolites ITQ-1, ITQ-3 and SSZ-23, J. Mater. Chem., 1999, 9(2), 349–351, 10.1039/A808059C.
- L. A. Villaescusa, P. Lightfoot, S. J. Teat and R. E. Morris, Variable-Temperature Microcrystal X-Ray Diffraction Studies of Negative Thermal Expansion in the Pure Silica Zeolite IFR, J. Am. Chem. Soc., 2001, 123(23), 5453–5459, DOI:10.1021/ja015797o.
- P. Lightfoot, D. A. Woodcock, M. J. Maple, L. A. Villaescusa and P. A. Wright, The Widespread Occurrence of Negative Thermalexpansion in Zeolites, J. Mater. Chem., 2001, 11(1), 212–216, 10.1039/B002950P.
- I. Bull, P. Lightfoot, L. A. Villaescusa, L. M. Bull, R. K. B. Gover, J. S. O. Evans and R. E. Morris, An X-Ray Diffraction and MAS NMR Study of the Thermal Expansion Properties of Calcined Siliceous Ferrierite, J. Am. Chem. Soc., 2003, 125(14), 4342–4349, DOI:10.1021/ja0292400.
- T. Carey, A. Corma, F. Rey, C. C. Tang, J. A. Hriljac and P. A. Anderson, The Effect of Extra Framework Species on the Intrinsic Negative Thermal Expansion Property of Zeolites with the LTA Topology, Chem. Commun., 2012, 48(47), 5829–5831, 10.1039/C2CC30582H.
- D. R. Corbin, L. Abrams, G. A. Jones, M. L. Smith, C. R. Dybowski, J. A. Hriljac and J. B. Parise, Entrapment and Controlled Release of Xenon in Cd2+-Exchanged Zeolite Rho, J. Chem. Soc. Chem. Commun., 1993,(12), 1027–1029, 10.1039/C39930001027.
- M. L. Smith, D. R. Corbin, L. Abrams and C. Dybowski, Flexibility of Zeolite Rho: Xenon-129 NMR Studies of Entrapped Xenon in Cadmium-Rho, J. Phys. Chem., 1993, 97(30), 7793–7795, DOI:10.1021/j100132a001.
- Y. Lee, B. A. Reisner, J. C. Hanson, G. A. Jones, J. B. Parise, D. R. Corbin, B. H. Toby, A. Freitag and J. Z. Larese, New Insight into Cation Relocations within the Pores of Zeolite Rho: In Situ Synchrotron X-Ray and Neutron Powder Diffraction Studies of Pb- and Cd-Exchanged Rho, J. Phys. Chem. B, 2001, 105(30), 7188–7199, DOI:10.1021/jp0100349.
- N. A. Ramsahye and R. G. Bell, Calculating the Energy Barriers to Sodium Cation Motion through the Six-Rings of Zeolite Y, Microporous Mesoporous Mater., 2008, 109(1), 405–412, DOI:10.1016/j.micromeso.2007.05.065.
- A. Nearchou, M.-L. U. Cornelius, Z. L. Jones, I. E. Collings, S. A. Wells, P. R. Raithby and A. Sartbaeva, Pressure-Induced Symmetry Changes in Body-Centred Cubic Zeolites, R. Soc. Open Sci., 2019, 6(7), 182158, DOI:10.1098/rsos.182158.
- G. D. Gatta and Y. Lee, Zeolites at High Pressure: A Review, Mineral. Mag., 2014, 78(2), 267–291, DOI:10.1180/minmag.2014.078.2.04.
- R. Arletti, O. Ferro, S. Quartieri, A. Sani, G. Tabacchi and G. Vezzalini, Structural Deformation Mechanisms of Zeolites under Pressure, Am. Mineral., 2003, 88(10), 1416–1422, DOI:10.2138/am-2003-1004.
- G. D. Gatta, F. Nestola and T. B. Ballaran, Elastic Behavior, Phase Transition, and Pressure Induced Structural Evolution of Analcime, Am. Mineral., 2006, 91(4), 568–578, DOI:10.2138/am.2006.1994.
- G. D. Gatta, N. Rotiroti, T. B. Ballaran and A. Pavese, Leucite at High Pressure: Elastic Behavior, Phase Stability, and Petrological Implications, Am. Mineral., 2008, 93(10), 1588–1596, DOI:10.2138/am.2008.2932.
- G. D. Gatta, N. Rotiroti, T. B. Ballaran, C. Sanchez-Valle and A. Pavese, Elastic Behavior and Phase Stability of Pollucite, a Potential Host for Nuclear Waste, Am. Mineral., 2009, 94(8–9), 1137–1143, DOI:10.2138/am.2009.3195.
- S. Ori, S. Quartieri, G. Vezzalini and V. Dmitriev, Pressure-Induced Structural Deformation and Elastic Behavior of Wairakite, Am. Mineral., 2008, 93(1), 53–62, DOI:10.2138/am.2008.2554.
- A. Sartbaeva, G. D. Gatta and S. A. Wells, Flexibility Window Controls Pressure-Induced Phase Transition in Analcime, Europhys. Lett., 2008, 83(2), 26002, DOI:10.1209/0295-5075/83/26002.
- S. A. Wells, A. Sartbaeva and G. D. Gatta, Flexibility Windows and Phase Transitions of Ordered and Disordered ANA Framework Zeolites, Europhys. Lett., 2011, 94(5), 56001, DOI:10.1209/0295-5075/94/56001.
- A. Sartbaeva and S. A. Wells, Framework Flexibility and Rational Design of New Zeolites for Catalysis, Appl. Petrochem. Res., 2012, 2(3), 69–72, DOI:10.1007/s13203-012-0017-3.
- G. D. Gatta, A Comparative Study of Fibrous Zeolites under Pressure, Eur. J. Mineral., 2005, 411–422, DOI:10.1127/0935-1221/2005/0017-0411.
- P. Ballone, S. Quartieri, A. Sani and G. Vezzalini, High-Pressure Deformation Mechanism in Scolecite: A Combined Computational experimental Study, Am. Mineral., 2002, 87(8–9), 1194–1206, DOI:10.2138/am-2002-8-919.
- P. Comodi, G. D. Gatta and P. F. Zanazzi, High-Pressure Structural Behaviour of Scolecite, Eur. J. Mineral., 2002, 567–574, DOI:10.1127/0935-1221/2002/0014-0567.
- D. Lee and S. T. Oyama, Gas Permeation Characteristics of a Hydrogen Selective Supported Silica Membrane, J. Membr. Sci., 2002, 210(2), 291–306, DOI:10.1016/S0376-7388(02)00389-7.
- D. R. Corbin, L. Abrams, G. A. Jones, M. M. Eddy, W. T. A. Harrison, G. D. Stucky and D. E. Cox, Flexibility of the Zeolite RHO Framework: In Situ X-Ray and Neutron Powder Structural Characterization of Divalent Cation-Exchanged Zeolite RHO, J. Am. Chem. Soc., 1990, 112(12), 4821–4830, DOI:10.1021/ja00168a029.
- Y. Lee, J. A. Hriljac, T. Vogt, J. B. Parise, M. J. Edmondson, P. A. Anderson, D. R. Corbin and T. Nagai, Phase Transition of Zeolite RHO at High-Pressure, J. Am. Chem. Soc., 2001, 123(34), 8418–8419, DOI:10.1021/ja0161554.
- K. Knorr, B. Winkler and V. Milman, Compression Mechanism of Cubic Silica Sodalite [Si12O24]: A First Principles Study of the Im
m to I
3m Phase Transition, Z. Kristallogr. - Cryst. Mater., 2001, 216(9), 495–500, DOI:10.1524/zkri.216.9.495.20347.
- S. Werner, S. Barrth, R. Jordan and H. Schulz, Single Crystal Study of Sodalite at High Pressure, Z. Kristallogr. - Cryst. Mater., 1996, 211(3), 158–162, DOI:10.1524/zkri.1996.211.3.158.
- J. Shang, G. Li, R. Singh, Q. Gu, K. M. Nairn, T. J. Bastow, N. Medhekar, C. M. Doherty, A. J. Hill, J. Z. Liu and P. A. Webley, Discriminative Separation of Gases by a “Molecular Trapdoor” Mechanism in Chabazite Zeolites, J. Am. Chem. Soc., 2012, 134(46), 19246–19253, DOI:10.1021/ja309274y.
- M. M. Lozinska, J. P. S. Mowat, P. A. Wright, S. P. Thompson, J. L. Jorda, M. Palomino, S. Valencia and F. Rey, Cation Gating and Relocation during the Highly Selective “Trapdoor” Adsorption of CO2 on Univalent Cation Forms of Zeolite Rho, Chem. Mater., 2014, 26(6), 2052–2061, DOI:10.1021/cm404028f.
- N. E. R. Zimmermann, S. Jakobtorweihen, E. Beerdsen, B. Smit and F. J. Keil, In-Depth Study of the Influence of Host−Framework Flexibility on the Diffusion of Small Gas Molecules in One-Dimensional Zeolitic Pore Systems, J. Phys. Chem. C, 2007, 111(46), 17370–17381, DOI:10.1021/jp0746446.
- T. J. H. Vlugt and M. Schenk, Influence of Framework Flexibility on the Adsorption Properties of Hydrocarbons in the Zeolite Silicalite, J. Phys. Chem. B, 2002, 106(49), 12757–12763, DOI:10.1021/jp0263931.
- F. Leroy, B. Rousseau and A. H. Fuchs, Self-Diffusion of n-Alkanes in Silicalite Using Molecular Dynamics Simulation: A Comparison between Rigid and Flexible Frameworks, Phys. Chem. Chem. Phys., 2004, 6(4), 775–783, 10.1039/B310273D.
- V. V. Guliants and A. J. Huth, Force Fields for Classical Atomistic Simulations of Small Gas Molecules in Silicalite-1 for Energy-Related Gas Separations at High Temperatures, J. Porous Mater., 2013, 20(4), 741–751, DOI:10.1007/s10934-012-9649-z.
- R. V. Awati, P. I. Ravikovitch and D. S. Sholl, Efficient and Accurate Methods for Characterizing Effects of Framework Flexibility on Molecular Diffusion in Zeolites: CH4 Diffusion in Eight Member Ring Zeolites, J. Phys. Chem. C, 2013, 117(26), 13462–13473, DOI:10.1021/jp402959t.
- S. Caro-Ortiz, E. Zuidema, M. Rigutto, D. Dubbeldam and T. J. H. Vlugt, Effects of Framework Flexibility on the Adsorption and Diffusion of Aromatics in MFI-Type Zeolites, J. Phys. Chem. C, 2020, 124(44), 24488–24499, DOI:10.1021/acs.jpcc.0c08054.
- S. Caro-Ortiz, E. Zuidema, D. Dekker, M. Rigutto, D. Dubbeldam and T. J. H. Vlugt, Adsorption of Aromatics in MFI-Type Zeolites: Experiments and Framework Flexibility in Monte Carlo Simulations, J. Phys. Chem. C, 2020, 124(39), 21782–21797, DOI:10.1021/acs.jpcc.0c06096.
- A. J. O'Malley and C. R. A. Catlow, Molecular Dynamics Simulations of Longer N-Alkanes in Silicalite: A Comparison of Framework and Hydrocarbon Models, Phys. Chem. Chem. Phys., 2013, 15(43), 19024–19030, 10.1039/C3CP52653D.
- H. Xiong, Z. Liu, X. Chen, H. Wang, W. Qian, C. Zhang, A. Zheng and F. Wei,
In Situ Imaging of the Sorption-Induced Subcell Topological Flexibility of a Rigid Zeolite Framework, Science, 2022, 376(6592), 491–496, DOI:10.1126/science.abn7667.
- A. García-Sánchez, D. Dubbeldam and S. Calero, Modeling Adsorption and Self-Diffusion of Methane in LTA Zeolites: The Influence of Framework Flexibility, J. Phys. Chem. C, 2010, 114(35), 15068–15074, DOI:10.1021/jp1059215.
- P. J. Bereciartua, Á. Cantín, A. Corma, J. L. Jordá, M. Palomino, F. Rey, S. Valencia, E. W. Corcoran, P. Kortunov, P. I. Ravikovitch, A. Burton, C. Yoon, Y. Wang, C. Paur, J. Guzman, A. R. Bishop and G. L. Casty, Control of Zeolite Framework Flexibility and Pore Topology for Separation of Ethane and Ethylene, Science, 2017, 358(6366), 1068–1071, DOI:10.1126/science.aao0092.
- X. Guo and A. Navrotsky, Hydration Dynamics in Zeolite A – An X-Ray Diffraction and Infrared Spectroscopic Study, Microporous Mesoporous Mater., 2018, 268, 197–201, DOI:10.1016/j.micromeso.2018.04.040.
- J. M. Castillo, J. Silvestre-Albero, F. Rodriguez-Reinoso, T. J. H. Vlugt and S. Calero, Water Adsorption in Hydrophilic Zeolites: Experiment and Simulation, Phys. Chem. Chem. Phys., 2013, 15(40), 17374–17382, 10.1039/C3CP52910J.
- X. Guo, L. Wu and A. Navrotsky, Thermodynamic Evidence of Flexibility in H2O and CO2 Absorption of Transition Metal Ion Exchanged Zeolite LTA, Phys. Chem. Chem. Phys., 2018, 20(6), 3970–3978, 10.1039/C7CP08188J.
- S. Hiraide, Y. Sakanaka, Y. Iida, H. Arima, M. T. Miyahara and S. Watanabe, Theoretical Isotherm Equation for Adsorption-Induced Structural Transition on Flexible Metal–Organic Frameworks, Proc. Natl. Acad. Sci. U. S. A., 2023, 120(31), e2305573120, DOI:10.1073/pnas.2305573120.
- J. Canivet, A. Fateeva, Y. Guo, B. Coasne and D. Farrusseng, Water Adsorption in MOFs: Fundamentals and Applications, Chem. Soc. Rev., 2014, 43(16), 5594–5617, 10.1039/C4CS00078A.
- J. Jänchen, D. Ackermann, H. Stach and W. Brösicke, Studies of the Water Adsorption on Zeolites and Modified Mesoporous Materials for Seasonal Storage of Solar Heat, Sol. Energy, 2004, 76(1), 339–344, DOI:10.1016/j.solener.2003.07.036.
- A. G. Greenaway, J. Shin, P. A. Cox, E. Shiko, S. P. Thompson, S. Brandani, S. B. Hong and P. A. Wright, Structural Changes of Synthetic Paulingite (Na,H-ECR-18) upon Dehydration and CO2 Adsorption, Z. Kristallogr. - Cryst. Mater., 2015, 230(4), 223–231, DOI:10.1515/zkri-2014-1824.
- P. Bordat, P.-A. Cazade, I. Baraille and R. Brown, Host and Adsorbate Dynamics in Silicates with Flexible Frameworks: Empirical Force Field Simulation of Water in Silicalite, J. Chem. Phys., 2010, 132(9), 094501, DOI:10.1063/1.3314286.
- H. J. Choi, J. G. Min, S. H. Ahn, J. Shin, S. B. Hong, S. Radhakrishnan, C. V. Chandran, R. G. Bell, E. Breynaert and C. E. A. Kirschhock, Framework Flexibility-Driven CO2 Adsorption on a Zeolite, Mater. Horiz., 2020, 7(6), 1528–1532, 10.1039/D0MH00307G.
- W. Louisfrema, J.-L. Paillaud, F. Porcher, E. Perrin, T. Onfroy, P. Massiani, A. Boutin and B. Rotenberg, Cation Migration and Structural Deformations upon Dehydration of Nickel-Exchanged NaY Zeolite: A Combined Neutron Diffraction and Monte Carlo Study, J. Phys. Chem. C, 2016, 120(32), 18115–18125, DOI:10.1021/acs.jpcc.6b05657.
- X. Guo, P. Zhang and A. Navrotsky, The Thermodynamics of Gas Absorption and Guest-Induced Flexibility in Zeolite Y, Microporous Mesoporous Mater., 2020, 294, 109893, DOI:10.1016/j.micromeso.2019.109893.
- V. M. Georgieva, E. L. Bruce, M. C. Verbraeken, A. R. Scott, W. J. Casteel, S. Brandani and P. A. Wright, Triggered Gate Opening and Breathing Effects during Selective CO2 Adsorption by Merlinoite Zeolite, J. Am. Chem. Soc., 2019, 141(32), 12744–12759, DOI:10.1021/jacs.9b05539.
- D. R. Corbin, L. Abrams, G. A. Jones, R. L. Harlow and P. J. Dunn, Flexibility of the Zeolite RHO Framework: Effect of Dehydration on the Crystal Structure of the Beryllophosphate Mineral, Pahasapaite, Zeolites, 1991, 11(4), 364–367, DOI:10.1016/0144-2449(91)80302-G.
- J. B. Parise and D. E. Cox, Structural Changes Occurring upon Dehydration of Zeolite Rho. A Study Using Neutron Powder Diffraction and Distance-Least-Squares Structural Modeling, J. Phys. Chem., 1984, 88(8), 1635–1640, DOI:10.1021/j150652a039.
- X. Guo, D. R. Corbin and A. Navrotsky, Thermodynamics of H2O and CO2 Absorption and Guest-Induced Phase Transitions in Zeolite RHO, J. Phys. Chem. C, 2018, 122(35), 20366–20376, DOI:10.1021/acs.jpcc.8b06070.
- M. Palomino, A. Corma, J. L. Jordá, F. Rey and S. Valencia, Zeolite Rho: A Highly Selective Adsorbent for CO2/CH4 Separation Induced by a Structural Phase Modification, Chem. Commun., 2011, 48(2), 215–217, 10.1039/C1CC16320E.
- M. Pera-Titus, M. Palomino, S. Valencia and F. Rey, Thermodynamic Analysis of Framework Deformation in Na,Cs-RHO Zeolite upon CO2 Adsorption, Phys. Chem. Chem. Phys., 2014, 16(44), 24391–24400, 10.1039/C4CP03409K.
- G. Y. Gor, P. Huber and N. Bernstein, Adsorption-Induced Deformation of Nanoporous Materials—A Review, Appl. Phys. Rev., 2017, 4(1), 011303, DOI:10.1063/1.4975001.
- E. Włoch, B. Sulikowski, R. Dula and E. M. Serwicka, Cation Environment and Migration in Iron-Exchanged Zeolite Na Y Studied by ESR, Colloids Surf., A, 1996, 115, 257–265, DOI:10.1016/0927-7757(96)89436-2.
- X. Du, H. Zhang, X. Li, Z. Tan, H. Liu and X. Gao, Cation Location and Migration in Lanthanum-Exchanged NaY Zeolite, Chin. J. Catal., 2013, 34(8), 1599–1607, DOI:10.1016/S1872-2067(11)60622-6.
- T. D. Pham, M. R. Hudson, C. M. Brown and R. F. Lobo, On the Structure–Property Relationships of Cation-Exchanged ZK-5 Zeolites for CO2 Adsorption, ChemSusChem, 2017, 10(5), 946–957, DOI:10.1002/cssc.201601648.
- M. M. Lozinska, E. Mangano, J. P. S. Mowat, A. M. Shepherd, R. F. Howe, S. P. Thompson, J. E. Parker, S. Brandani and P. A. Wright, Understanding Carbon Dioxide Adsorption on Univalent Cation Forms of the Flexible Zeolite Rho at Conditions Relevant to Carbon Capture from Flue Gases, J. Am. Chem. Soc., 2012, 134(42), 17628–17642, DOI:10.1021/ja3070864.
- T. De Baerdemaeker and D. De Vos, Trapdoors in Zeolites, Nat. Chem., 2013, 5(2), 89–90, DOI:10.1038/nchem.1560.
- J. Shang, G. Li, P. A. Webley and J. Z. Liu, A Density Functional Theory Study for the Adsorption of Various Gases on a Caesium-Exchanged Trapdoor Chabazite, Comput. Mater. Sci., 2016, 122, 307–313, DOI:10.1016/j.commatsci.2016.05.040.
- J. Shang, G. Li, R. Singh, P. Xiao, J. Z. Liu and P. A. Webley, Potassium Chabazite: A Potential Nanocontainer for Gas Encapsulation, J. Phys. Chem. C, 2010, 114(50), 22025–22031, DOI:10.1021/jp107456w.
- J. Shang, G. Li, R. Singh, P. Xiao, D. Danaci, J. Z. Liu and P. A. Webley, Adsorption of CO2, N2, and CH4 in Cs-Exchanged Chabazite: A Combination of van Der Waals Density Functional Theory Calculations and Experiment Study, J. Chem. Phys., 2014, 140(8), 084705, DOI:10.1063/1.4866455.
- F.-X. Coudert and D. Kohen, Molecular Insight into CO2 “Trapdoor” Adsorption in Zeolite Na-RHO, Chem. Mater., 2017, 29(7), 2724–2730, DOI:10.1021/acs.chemmater.6b03837.
- T. Du, X. Fang, L. Liu, J. Shang, B. Zhang, Y. Wei, H. Gong, S. Rahman, E. F. May, P. A. Webley and G. Li, An Optimal Trapdoor Zeolite for Exclusive Admission of CO2 at Industrial Carbon Capture Operating Temperatures, Chem. Commun., 2018, 54(25), 3134–3137, 10.1039/C8CC00634B.
- M. C. Verbraeken, R. Mennitto, V. M. Georgieva, E. L. Bruce, A. G. Greenaway, P. A. Cox, J. G. Min, S. B. Hong, P. A. Wright and S. Brandani, Understanding CO2 Adsorption in a Flexible Zeolite through a Combination of Structural, Kinetic and Modelling Techniques, Sep. Purif. Technol., 2021, 256, 117846, DOI:10.1016/j.seppur.2020.117846.
- M. L. U. Cornelius, L. Price, S. A. Wells, L. F. Petrik and A. Sartbaeva, The Steric Influence of Extra-Framework Cations on Framework Flexibility: An
LTA Case Study, Z. Kristallogr. - Cryst. Mater., 2019, 234(7–8), 461–468, DOI:10.1515/zkri-2019-0016.
- E. Dib, E. B. Clatworthy, A. A. Paecklar, J. Grand, N. Barrier and S. Mintova, Role of Al Distribution in CO2 Adsorption Capacity in RHO Zeolites, J. Phys. Chem. C, 2023, 127(1), 3–10, DOI:10.1021/acs.jpcc.2c05479.
- D. R. Corbin, L. Abrams, G. A. Jones, M. M. Eddy, G. D. Stucky and D. E. Cox, Flexibility of the Zeolite Rho Framework. Neutron Powder Structural Characterization of Ca-Exchanged Zeolite Rho, J. Chem. Soc. Chem. Commun., 1989, 1, 42–43, 10.1039/C39890000042.
- K. B. Parise, D. R. Corbin, T. E. Gier, R. L. Harlow, L. Abrams and R. B. Von Dreele, Flexibility of the RHO Framework: A Comparison of the Rb-Exchange Zeolite and the Novel Composition Rb24Be24As24O96·3.2 D2O, Zeolites, 1992, 12(4), 360–368, DOI:10.1016/0144-2449(92)90031-J.
- S. R. G. Balestra, S. Hamad, A. R. Ruiz-Salvador, V. Domínguez-García, P. J. Merkling, D. Dubbeldam and S. Calero, Understanding Nanopore Window Distortions in the Reversible Molecular Valve Zeolite RHO, Chem. Mater., 2015, 27(16), 5657–5667, DOI:10.1021/acs.chemmater.5b02103.
- J. Shin, D. S. Bhange, M. B. Park and S. B. Hong, Structural Characterization of Various Alkali Cation Forms of Synthetic Aluminosilicate Natrolites, Microporous Mesoporous Mater., 2015, 210, 20–25, DOI:10.1016/j.micromeso.2015.02.013.
- Y. Lee, D. Seoung and Y. Lee, Natrolite Is Not a “Soda-Stone” Anymore: Structural Study of Alkali (Li+), Alkaline-Earth (Ca2+, Sr2+, Ba2+) and Heavy Metal (Cd2+, Pb2+, Ag+) Cation-Exchanged Natrolites, Am. Mineral., 2011, 96(11–12), 1718–1724, DOI:10.2138/am.2011.3853.
- J. Shin, S. H. Park and S. B. Hong, Abnormal Expansion of a Disordered Zeolite upon Ion Exchange of K+ by Mg2+, J. Phys. Chem. C, 2019, 123(29), 17894–17898, DOI:10.1021/acs.jpcc.9b04486.
- A. Sartbaeva, S. A. Wells, M. M. J. Treacy and M. F. Thorpe, The Flexibility Window in Zeolites, Nat. Mater., 2006, 5(12), 962–965, DOI:10.1038/nmat1784.
- S. A. Wells, K. M. Leung, P. P. Edwards and A. Sartbaeva, Flexibility Windows in Faujasite with Explicit Water and Methanol Extra-Framework Content, Dalton Trans., 2015, 44(13), 5978–5984, 10.1039/C4DT03150D.
- C. J. Dawson, V. Kapko, M. F. Thorpe, M. D. Foster and M. M. J. Treacy, Flexibility As an Indicator of Feasibility of Zeolite Frameworks, J. Phys. Chem. C, 2012, 116(30), 16175–16181, DOI:10.1021/jp2107473.
- E. Conterosito, M. Lopresti and L. Palin, In Situ X-Ray Diffraction Study of Xe and CO2 Adsorption in Y Zeolite: Comparison between Rietveld and PCA-Based Analysis, Crystals, 2020, 10(6), 483, DOI:10.3390/cryst10060483.
- S. Ghojavand, E. Dib, J. Rey, A. Daouli, E. B. Clatworthy, P. Bazin, V. Ruaux, M. Badawi and S. Mintova, Interplay between Alkali-Metal Cations and Silanol Sites in Nanosized CHA Zeolite and Implications for CO2 Adsorption, Commun. Chem., 2023, 6(1), 1–8, DOI:10.1038/s42004-023-00918-1.
- M. Ilkaeva, R. Vieira, J. M. P. Pereira, M. Sardo, I. Marin-Montesinos and L. Mafra, Assessing CO2 Capture in Porous Sorbents via Solid-State NMR-Assisted Adsorption Techniques, J. Am. Chem. Soc., 2023, 145(16), 8764–8769, DOI:10.1021/jacs.3c00281.
- S. Ghojavand, E. Dib, B. Riodent, A. Magisson, V. Ruaux and S. Mintova, Altering the Atomic Order in Nanosized CHA Zeolites by Postsynthetic Silylation Treatment, Adv. Sustainable Syst., 2023, 7(5), 2200480, DOI:10.1002/adsu.202200480.
- C. Helaine, H. Özçelik, S. Komaty, A. Amedlous, S. Ghojavand, D. Goux, R. Retoux, S. Mintova and S. Valable, Internalization Study of Nanosized Zeolite Crystals in Human Glioblastoma Cells, Colloids Surf., B, 2022, 218, 112732, DOI:10.1016/j.colsurfb.2022.112732.
- C. Anfray, S. Komaty, A. Corroyer-Dulmont, M. Zaarour, C. Helaine, H. Ozcelik, C. Allioux, J. Toutain, K. Goldyn, E. Petit, K. Bordji, M. Bernaudin, V. Valtchev, O. Touzani, S. Mintova and S. Valable, Nanosized Zeolites as a Gas Delivery Platform in a Glioblastoma Model, Biomaterials, 2020, 257, 120249, DOI:10.1016/j.biomaterials.2020.120249.
- K. Sahner, G. Hagen, D. Schönauer, S. Reiß and R. Moos, Zeolites — Versatile Materials for Gas Sensors, Solid State Ionics, 2008, 179(40), 2416–2423, DOI:10.1016/j.ssi.2008.08.012.
- Y. Zheng, X. Li and P. K. Dutta, Exploitation of Unique Properties of Zeolites in the Development of Gas Sensors, Sensors, 2012, 12(4), 5170–5194, DOI:10.3390/s120405170.
- X. Xu, J. Wang and Y. Long, Zeolite-Based Materials for Gas Sensors, Sensors, 2006, 6(12), 1751–1764, DOI:10.3390/s6121751.
- D. J. Wales, J. Grand, V. P. Ting, R. D. Burke, K. J. Edler, C. R. Bowen, S. Mintova and A. D. Burrows, Gas Sensing Using Porous Materials for Automotive Applications, Chem. Soc. Rev., 2015, 44(13), 4290–4321, 10.1039/C5CS00040H.
- L. E. Kreno, K. Leong, O. K. Farha, M. Allendorf, R. P. Van Duyne and J. T. Hupp, Metal–Organic Framework Materials as Chemical Sensors, Chem. Rev., 2012, 112(2), 1105–1125, DOI:10.1021/cr200324t.
- O. Shekhah, J. Liu, R. A. Fischer and C. Wöll, MOF Thin Films: Existing and Future Applications, Chem. Soc. Rev., 2011, 40(2), 1081–1106, 10.1039/C0CS00147C.
- M. Fakhraei Ghazvini, M. Vahedi, S. Najafi Nobar and F. Sabouri, Investigation of the MOF Adsorbents and the Gas Adsorptive Separation Mechanisms, J. Environ. Chem. Eng., 2021, 9(1), 104790, DOI:10.1016/j.jece.2020.104790.
- S. Krause, V. Bon, I. Senkovska, U. Stoeck, D. Wallacher, D. M. Többens, S. Zander, R. S. Pillai, G. Maurin, F.-X. Coudert and S. Kaskel, A Pressure-Amplifying Framework Material with Negative Gas Adsorption Transitions, Nature, 2016, 532(7599), 348–352, DOI:10.1038/nature17430.
|
This journal is © The Royal Society of Chemistry 2023 |