DOI:
10.1039/D3SC02868B
(Edge Article)
Chem. Sci., 2023,
14, 8869-8877
Thiol-triggered deconstruction of bifunctional silyl ether terpolymers via an SNAr-triggered cascade†
Received
6th June 2023
, Accepted 12th July 2023
First published on 2nd August 2023
Abstract
While Si-containing polymers can often be deconstructed using chemical triggers such as fluoride, acids, and bases, they are resistant to cleavage by mild reagents such as biological nucleophiles, thus limiting their end-of-life options and potential environmental degradability. Here, using ring-opening metathesis polymerization, we synthesize terpolymers of (1) a “functional” monomer (e.g., a polyethylene glycol macromonomer or dicyclopentadiene); (2) a monomer containing an electrophilic pentafluorophenyl (PFP) substituent; and (3) a cleavable monomer based on a bifunctional silyl ether
. Exposing these polymers to thiols under basic conditions triggers a cascade of nucleophilic aromatic substitution (SNAr) at the PFP groups, which liberates fluoride ions, followed by cleavage of the backbone Si–O bonds, inducing polymer backbone deconstruction. This method is shown to be effective for deconstruction of polyethylene glycol (PEG) based graft terpolymers in organic or aqueous conditions as well as polydicyclopentadiene (pDCPD) thermosets, significantly expanding upon the versatility of bifunctional silyl ether based functional polymers.
Introduction
Polymers that undergo selective bond cleavage1 in response to an external trigger have numerous applications, including in drug delivery,2–4 sensing,5,6 transient electronics,7,8 and recyclable materials.9,10 A wide range of functional groups (e.g., azo,11–13 dihydrofuran,14 disulfide,15,16 diselenium,17 ester,18 ketal,19 poly(benzyl ether),20 and phosphoramidate21) have been employed to enable triggered polymer deconstruction, and various external stimuli such as oxdiation/reduction,22,23 pH,24,25 light,12 heat,26 nucleophiles,27 and mechanical force28 have been extensively studied. Nevertheless, there is often a trade-off between the introduction of cleavable bonds and polymer stability under use conditions, especially when mild cleavage reagents (e.g., biological triggers), and thus easier-to-cleave bonds, are required.
Bifunctional silyl ethers (
; “BSEs”) are versatile functional groups that have found applications as fluoride- and acid/base-cleavable linkages in, for example, biomaterials,29 controlled release systems,30–32 and deconstructable thermosets (Fig. 1A).33–37 BSEs offer a unique combination of synthetic accessibility, compositional diversity,38 and excellent thermal and oxidative stabilities while providing for highly selective cleavage with rates that can be easily controlled through variation of the Si–R substituents.39 Moreover, cyclic olefins containing BSEs are suitable monomers for the synthesis of deconstructable homopolymers,40 copolymers,39 and thermosets via ring-opening metathesis polymerization (ROMP).35–38,41 Nevertheless, while acid and/or fluoride are convenient triggers for BSE cleavage, there are instances where such stimuli are not compatible with a desired application, leading us to consider alternative methods.
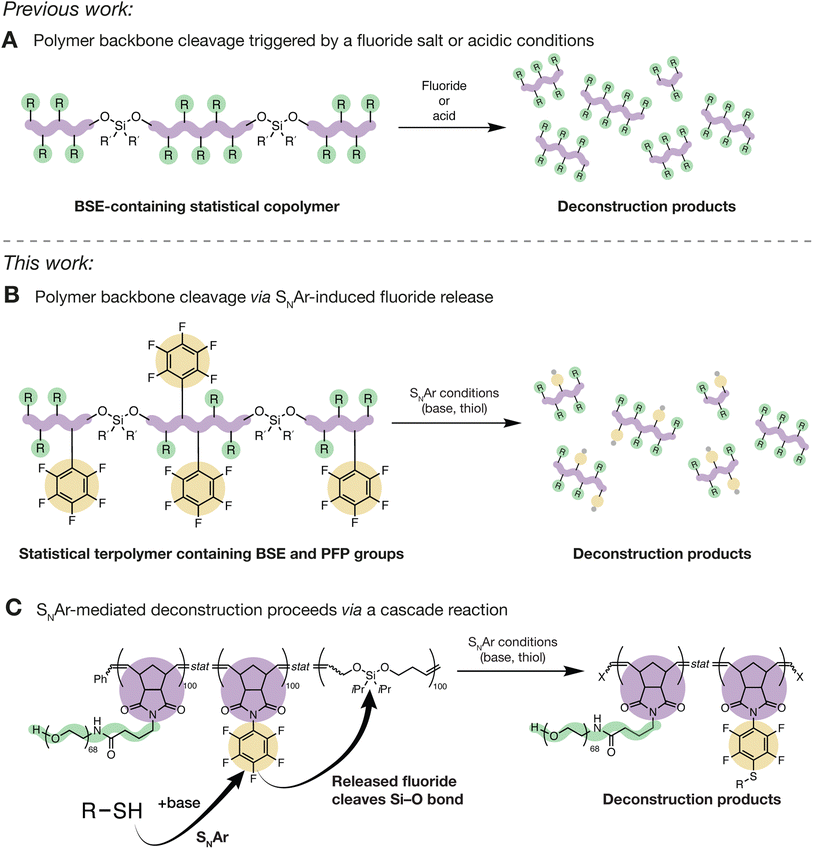 |
| Fig. 1 (A) We have previously reported deconstructable copolymers containing bifunctional silyl ethers (BSEs) where polymer backbone cleavage is initiated by a fluoride source (e.g., tetrabutylammonium fluoride, TBAF) or acidic conditions (e.g., HCl or carboxylic acids), providing oligomeric deconstruction fragments. (B) Here, we describe BSE-containing terpolymers containing pentafluorophenyl (PFP) comonomers, which trigger deconstruction upon exposure to nucleophilic aromatic substitution (SNAr) conditions (a thiol in the presence of base). (C) The SNAr-mediated cleavage proceeds via a cascade reaction, where thiolate attacks at the para-position of the PFP ring via SNAr, releasing a fluoride ion, which in turn cleaves the Si–O bond of the BSE. At full conversion, X = CH2CH2OH, CH2OH, Ph or H. | |
Inspired by advances in radical ring-opening polymerization—where sensitive electrophilic functional groups such as thioesters can be installed within polymer backbones to enable cleavage via nucleophilic attack27,42,43—and with interest in biodegradable polymers, we sought a strategy to facilitate the deconstruction of BSE-based polymers prepared via ROMP through exposure to nucleophiles such as thiols. While BSEs are not sufficiently reactive to undergo cleavage in the presence of thiols under typical conditions, we hypothesized that is would be possible to embed latent, thiol-triggered fluoride sources into BSE-containing polymers to facilitate Si–O bond cleavage following a nucleophilic aromatic substitution (SNAr) event. SNAr reactions of pentafluorophenyl (PFP) derivatives44 using a range of nucleophiles (e.g., alcohols,45 amines,46 phosphites,47 thiols44,48,49) liberate one equivalent of F− per substitution reaction, which we imagined could be utilized as the first step in an SNAr, fluoride release, and BSE cleavage cascade (Fig. 1B). Additionally, SNAr reactions of PFP groups are known to be effective reactions for polymer functionalization.50–54 PFPs are compatible with ROMP, and there are many cheap, benign, and/or biologically-derived thiols that could potentially be used to initiate such a cascade process. Here, we present the realization of this concept, showing that graft and covalently crosslinked thermoset terpolymers with BSE- and PFP-based comonomers undergo selective backbone cleavage via a thiol-triggered SNAr cascade. This work provides a new strategy to enable backbone cleavage of otherwise stable polymeric materials and extends the scope of BSE-based cleavage reactions for responsive materials design.
Results and discussion
Small molecule model studies
First, we sought to determine whether or not BSEs are stable under typical conditions used for SNAr reactions involving PFP groups, which often involve polar organic solvents and bases such as K2CO3, Cs2CO3 or 1,8-diazabicyclo[5,4,0]undec-7-ene (DBU).44 PFP-based monomer Nb-PFP (synthesized following a reported procedure55) and iPrAl, a BSE mimic of a polymer backbone, were exposed to various conditions relevant to SNAr (Fig. 2A); gas chromatography-mass spectrometry (GC-MS) was used to monitor iPrAl cleavage (mesitylene was used as an internal standard; see ESI† section “Small Molecule GC-MS Studies” for further information; see ESI† section “Small molecule syntheses” for details). iPrAl remained intact when treated with either 1-dodecanethiol (nucleophile, 1.5 equiv.), K2CO3 (base, 2.0 equiv.), Nb-PFP (latent fluoride, 1.5 equiv.), a combination of 1-dodecanethiol and K2CO3 (nucleophile and base only), or with a combination of Nb-PFP and K2CO3 (latent fluoride and base) (Fig. 2B). When iPrAl was exposed to 1-dodecanethiol (1.5 equiv.), K2CO3 (2.0 equiv.), and Nb-PFP (1.5 equiv., i.e., all three reagents critical for SNAr), however, >98% cleavage was observed after 1 h and no trace of iPrAl was seen after 2 h (Fig. S1†). 19F NMR spectroscopy of the reaction mixture showed a doublet of doublets of doublets and a multiplet with a downfield shift when compared to the parent Nb-PFP (Fig. S2A†). This spectrum agrees well with the spectrum obtained for an independently synthesized, authentic sample of the expected para-1-dodecanethiol-substituted product Nb-PFP-SC12H25 (see ESI† section “Small molecule syntheses” for details). Moreover, a 19F–19F COSY experiment showed a correlation between the two new 19F resonances generated under the reaction conditions (Fig. S2B†). Altogether, these results confirm that SNAr occurs between 1-dodecanethiol and Nb-PFP in the presence of K2CO3, and that the resulting fluoride can cleave a model BSE.
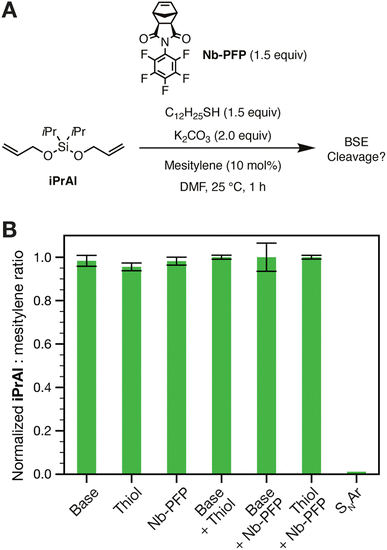 |
| Fig. 2 (A) Scheme showing the methodology for the small molecule GC-MS studies of acyclic BSEiPrAl. (B) Bar chart comparing the ratio of iPrAl to a mesitylene internal standard (10 mol%) from GC-MS runs. iPrAl is treated with either K2CO3 (base), Nb-PFP, 1-dodecanethiol (thiol), or combinations of the above in DMF for 1 h at 25 °C. Ratios were calculated using peak integration and averaged over three runs. Error bars show standard deviation. | |
Nb-PFP homopolymer model studies
To test the viability of SNAr in a ROMP-derived PFP-containing polymer, we subjected Nb-PFP (225 equiv.) to Grubbs' 3rd generation bispyridyl initiator (1 equiv.) in 1,4-dioxane, providing polyNb-PFP (Fig. 3A, see ESI† section “Polymer syntheses” for details). The 1H NMR spectrum of polyNb-PFP agrees well with that reported by Tlenkopatchev and coworkers for similar polymers synthesized using Grubbs 1st and 2nd-generation initiators.56 Complete consumption of Nb-PFP was observed (Fig. S3†). 19F NMR showed the same three general sets of resonances for polyNb-PFP and Nb-PFP (Fig. S4†). PolyNb-PFP had a low dispersity (Đ = 1.05) and a number average molecular weight (Mn) of 78
400, close to its theoretical Mn of 74
100, as determined by size exclusion chromatography (SEC, CHCl3 mobile phase) (Fig. 3B, black trace). Exposure of polyNb-PFP to C12H25SH (1.2 equiv.) and K2CO3 (2.0 equiv.) in DMF at 25 °C (Fig. 3C, see ESI† section “Polymer syntheses” for details) for 1 h gave quantitative conversion to the para-substituted SNAr product as determined by 19F NMR spectroscopy (Fig. 3D). SEC (CHCl3 mobile phase) showed a concomitant increase in molecular weight (Mn of 123
800, close to its theoretical Mn of 115
100) and slight increase in dispersity (Đ = 1.15) (Fig. 3B). A small shoulder is observed, which we attribute to aggregation of the substituted polymer, likely caused by the addition of dodecyl groups to the polymer sidechains.
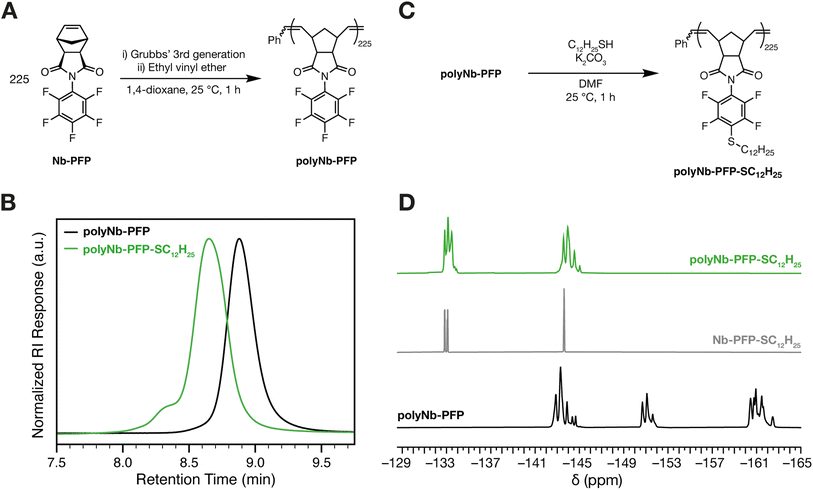 |
| Fig. 3 (A) Synthesis of polyNb-PFP homopolymer. (B) SEC traces (CHCl3 mobile phase) for polyNb-PFP (black) and SNAr product polyNb-PFP–SC12H25. (C) Synthesis of SNAr product polyNb-PFP-SC12H25. (D) 19F NMR spectra (565 MHz, CDCl3, 25 °C) comparing polyNb-PFP (black) to monomer Nb-PFP–SC12H25 (grey) and polyNb-PFP–SC12H25 (green). | |
Terpolymer deconstruction using an SNAr-initiated cascade
After successfully demonstrating SNAr of polyNb-PFP, we set out to test our hypothesis that an SNAr-triggered cascade can enable deconstruction of polymers with backbone BSE groups. Nb-PFP was combined with BSE-containing monomer iPrSi8 (synthesized according to a reported procedure39) and norbornene-terminated 3 kDa polyethylene glycol (PEG) macromonomer57PEG-MM in a 1
:
1
:
1 molar ratio in 1,4-dioxane and exposed to Grubbs' 3rd-generation bis-pyridyl initiator to generate graft terpolymer P1 (total monomer
:
initiator ratio 300
:
1) (Fig. 4A, see ESI† section “Polymer syntheses” for experimental details). High conversions of iPrSi8, Nb-PFP and PEG-MM were confirmed via1H NMR (Fig. S5†) and 19F NMR spectroscopy (Fig. S6†) to yield P1 with Mn of 636
000 (±18.6%) and Đ = 1.57 (See ESI† section “Materials and Methods” for a discussion on Mn calculations). To confirm that BSEs are incorporated into the polymer backbone, P1 was exposed to 1 M aqueous HCl (see ESI† section “Degradation studies” for experimental details), conditions previously shown to cleave BSEs in norbornene-based terpolymers.39 SEC analysis revealed macromolecular deconstruction products at longer retention times, indicative of polymer backbone cleavage resulting in monomers, dimers, trimers, and higher oligomers39 (Fig. 4B). Moreover, 19F NMR spectroscopy shows that the PFP group remains unaffected under these conditions (Fig. 4C).
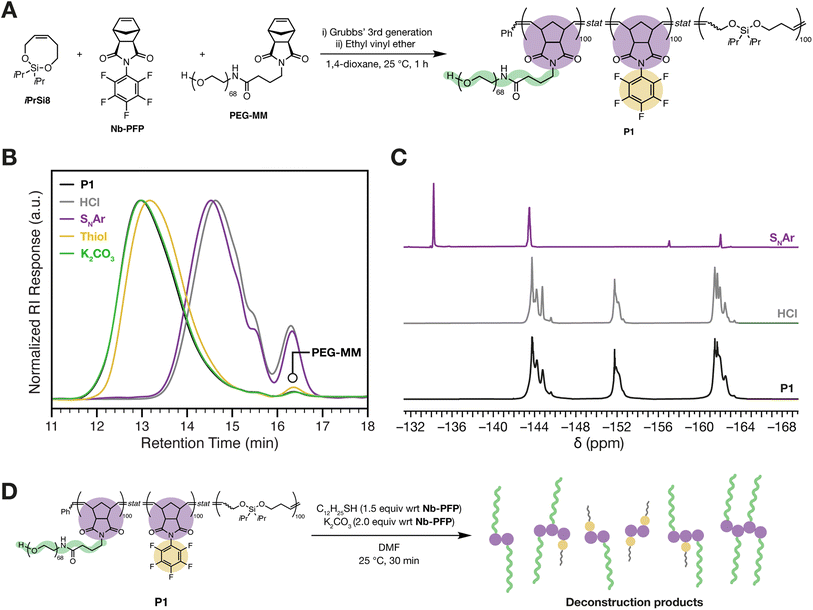 |
| Fig. 4 (A) Synthesis of polynorbornene graft terpolymer P1via ROMP. Incorporation of PEG-MM into the terpolymer enables facile tracking of subsequent deconstruction. (B) SEC traces (DMF mobile phase) showing polymer P1 (black), the control deconstruction of P1 using HCl (grey), the control deconstruction of P1 using K2CO3 (green), the control deconstruction of P1 using 1-dodecanethiol (thiol, yellow) and the deconstruction of P1 using the SNAr conditions of 1-dodecanethiol and K2CO3 (purple). (C) 19F NMR spectra (565 MHz, CDCl3, 25 °C) comparing the parent terpolymer P1 (black), to the deconstruction products using HCl (grey), or SNAr conditions (purple). (D) Schematic showing the deconstruction of P1 using SNAr conditions. | |
Gratifyingly, exposure of P1 to SNAr conditions—K2CO3 (2.0 equiv. w.r.t. Nb-PFP in the terpolymer) and 1-dodecanethiol (1.5 equiv. w.r.t. Nb-PFP in the terpolymer) in DMF—for 30 min at 25 °C led to backbone deconstruction and a nearly identical SEC trace to that for HCl-induced deconstruction (Fig. 4B, purple trace; see ESI† section “Deconstruction studies” for experimental details). 19F NMR spectroscopy confirms formation of the expected SNAr product, suggesting that deconstruction is triggered by SNAr (Fig. 4C). Notably, exposing the polymer to K2CO3 alone (in DMF at room temperature) for the same time period does not lead to any polymer cleavage by SEC (Fig. 4B, green trace, Mn = 536
000 (±17.1%), Đ = 1.53); exposing the polymer to 1-dodacenethiol in the absence of base leads to a small shift in the SEC peak to a longer retention time, suggesting a small amount of decomposition likely due to background SNAr (Fig. 4B, yellow trace, Mn = 268
000 (±14.6%), Đ = 1.57).
PEG-based graft terpolymer deconstruction under aqueous conditions
We initially designed iPrSi8 and related monomers39 for the purpose of enabling the deconstruction of PEG-based graft terpolymers under aqueous conditions that may be translatable to applications in drug delivery and biological imaging.58–62 Delaittre and coworkers reported thiol-mediated SNAr of PFP-containing poly(N,N-dimethylacrylamide) in alkaline water (pH ≥ 11),63 which inspired us to investigate backbone deconstruction of P1 under similar conditions. First, we exposed the polymer to Na2HPO4/NaOH buffer solution (pH = 12; see ESI† section “Degradation studies” for full experimental details) for 30 min at 25 °C; very little change was observed by SEC following this treatment, suggesting that the polymer is stable in aqueous alkaline buffer (Fig. S7†). When 2-mercaptoethanol (1.5 equiv. per PFP group) was added to this solution, which is sufficiently basic to generate the corresponding thiolate (pKa of 2-mercaptoethanol = 9.72),64 complete polymer deconstruction was observed in 30 min at room temperature (Fig. 5). 19F NMR spectroscopy confirms the formation of SNAr products, consistent with SNAr-induced fluoride release and BSE cleavage (Fig. S8†). The efficiency of this reaction is notable; previous work suggested that elevated temperatures (e.g., 40 °C), extend reaction times (e.g., 72 h), and large excesses of thiols (e.g., 10–20 equiv.) are needed to induce SNAr of polymeric PFP groups under aqueous conditions.63 Here, deconstruction may be facilitated by the hydrophobic local environment of P1, where the PFP and BSE groups reside, which may elevate the local concentration of thiol and/or stabilize the SNAr transition state.
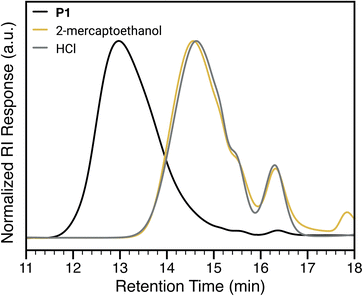 |
| Fig. 5 Deconstruction of P1 in pH 12 buffer. SEC traces (DMF mobile phase) of the parent terpolymer (black), HCl-deconstructed material (grey), and P1 exposed to 2-mercaptoethanol in pH 12 Na2HPO4/NaOH buffer solution (yellow). | |
Thiol-triggered cascade deconstruction of polydicyclopentadiene thermosets
We have shown that BSE-containing monomers such as iPrSi8 can be copolymerized with dicyclopentadiene (DCPD) to generate deconstructable38,41 and remoldable35 polydicyclopentadiene (pDCPD) thermosets and composites, offering a new end-of-life strategy for this high-performance engineering material.65 Nevertheless, in these studies we have used 1 M TBAF, 1 M HCl, or high-temperature treatment with octanoic acid as deconstruction triggers; we hypothesized that the SNAr-triggered cascade process introduced here could serve as an alternative method for pDCPD deconstruction.
To test this hypothesis, we prepared two thermosets: the first—thermoset T1 (Fig. 6A)—was prepared by curing a liquid resin containing DCPD (14.4 equiv.) with 10% v/v of iPrSi8 (1.0 equiv.) and Nb-PFP (2.0 equiv.); the second—thermoset T2 (Fig. 6B)—was prepared by curing a resin containing DCPD with 10% v/v of iPrSi8 only (see ESI† section “Thermoset materials” for full experimental details). Two equivalents of Nb-PFP with respsect to iPrSi8 were chosen to facilitate full material deconstruction; as there are 2 Si–O bonds per BSE, 2 equiv. of fluoride are required to achieve complete Si–O bond cleavage. Dynamic mechanical analysis (DMA, Fig. S9 and Table S1†) showed that the glass transition temperature (Tg) of T1 (Tg = 67 °C) was highly depressed compared to T2 (Tg = 134 °C), which is likely due to dilution of the thermoset crosslink density through incorporation of Nb-PFP. Nevertheless, thermogravimetric analysis (TGA, Fig. S10 and Table S2†) showed that the decomposition temperatures (Td) of T1 (Td = 458 °C (plus a secondary shoulder at 550 °C due to addition of Nb-PFP)) and T2 (Td = 464 °C) were similar, suggesting that the addition of Nb-PFP does not have a major negative impact on the stability of these two thermoset materials. The storage moduli (E′) and loss moduli (E′′) of the thermosets were determined by DMA (Fig. S11 and S12,† respectively). T1 and T2 displayed similar E′ at 30 °C (well below their respective Tg values) when compared to a neat pDCPD sample. Lower rubbery moduli (i.e., moduli collected above Tg) were observed for Nb-PFP-containing T1 when compared to T2 or neat pDCPD, which is also consistent with a reduced crosslink density.35
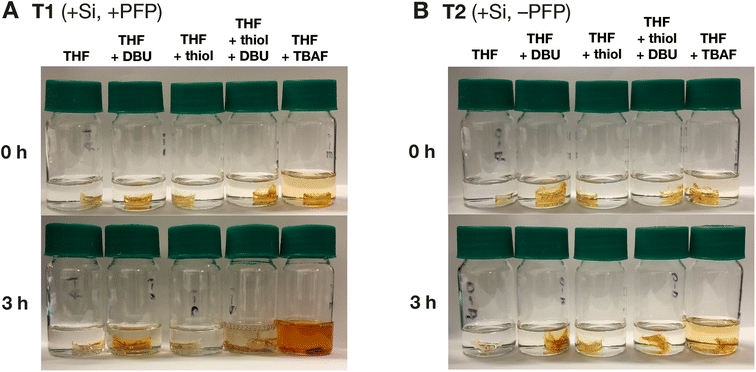 |
| Fig. 6 Deconstruction of BSE-containing pDCPD thermoset materials. (A) Thermoset T1 (+Si, +PFP) prepared using pDCPD, iPrSi8 (10% v/v) and Nb-PFP (2.0 equiv. w.r.t. iPrSi8) in solutions of: THF; THF and DBU; THF and 1-dodecanethiol (thiol); THF, thiol, and DBU; THF and TBAF at 25 °C, after 0 and 3 h. (B) Control thermoset T2 (+Si, −PFP) prepared using pDCPD and iPrSi8 (10% v/v) in solutions of: THF; THF and DBU; THF and 1-dodecanethiol (thiol); THF, thiol, and DBU; THF and TBAF at 25 °C, after 0 and 3 h. | |
Both thermosets were exposed to SNAr conditions,44 in this case using 1-dodecanethiol (2.0 equiv. w.r.t. PFP groups) and the organic base 1,8-diazabicyclo[5,4,0]undec-7-ene (DBU, 2.0 equiv.) in tetrahydrofuran (THF) solvent, which facilitates swelling of the materials. In line with our hypothesis, T1 was almost completely deconstructed (8% residual mass) to form soluble products under these conditions (Fig. 6A) while T2 remained intact (Fig. 6B). Both thermosets were dissolved in 1 M TBAF in THF (4% residual mass), confirming that the stability of T2 under SNAr conditions is due to a lack of latent fluoride groups. Moreover, T1 did not dissolve in the presence of 1-dodecanethiol or DBU alone (Fig. S13–S16†). 19F NMR analysis of the soluble fragments of T1 deconstruction showed that under TBAF conditions (Fig. S17†), the three sets of resonances corresponding to the PFP group are retained and additional 19F resonances consistent with difluorosilane cleavage products are observed,36 in line with direct cleavage of the Si–O bond by TBAF. By contrast, fragments from SNAr conditions show two downfield 19F resonances (Fig. S18†), which is consistent with SNAr of the PFP groups.
Conclusions
In summary, we describe a new method to deconstruct BSE-containing macromolecules using thiol-mediated SNAr of PFP groups, which liberates fluoride and induces Si–O bond cleavage. The method is shown to be applicable to soluble graft terpolymers and insoluble thermosets, and it works in organic (DMF and THF) solvents or water with an appropriate base (K2CO3, DBU, and phosphate buffer) and nucleophile (1-dodecanethiol and 2-mercaptoethanol). This approach expands the scope of BSE chemistry and may provide new ways to couple chemical triggers (e.g., thiols) with polymer deconstruction to achieve novel functions and end-of-life options.
Author contributions
CMB synthesized starting materials and polymers, performed GC-MS studies, and organic and aqueous degradation studies. KELH and YW performed thermoset experiments. YW conducted DMA experiments, LJK synthesized flourinated silyl ether analogues, PS synthesized iPrSi8, HZ performed GC-MS experiments, and DJL synthesized PEG-MM. CMB, PS and JAJ conceived of the project. CMB and JAJ wrote the manuscript.
Conflicts of interest
CMB, PS and JAJ are named inventors on a patent application (US provisional application no. 63/195259) filed by the Massachusetts Institute of Technology on the monomers and copolymers in this work.
Acknowledgements
This work was supported by the NSF Center for the Chemistry of Molecularly Optimized Networks (MONET, CHE-2116298). We thank Dr Mohanraja Kumar for assistance with GC-MS studies. CMB acknowledges the Natural Sciences and Engineering Research Council of Canada (NSERC) for a Postdoctoral Fellowship. PS acknowledges the American Cancer Society for a Postdoctoral Fellowship and DJL acknowledges support from the National Science Foundation (NSF) Graduate Research Fellowship Program.
References
- P. Shieh, M. R. Hill, W. Zhang, S. L. Kristufek and J. A. Johnson, Clip Chemistry: Diverse (Bio)(Macro)Molecular and Material Function through Breaking Covalent Bonds, Chem. Rev., 2021, 121(12), 7059–7121, DOI:10.1021/acs.chemrev.0c01282.
- N. Kamaly, B. Yameen, J. Wu and O. C. Farokhzad, Degradable Controlled-Release Polymers and Polymeric Nanoparticles: Mechanisms of Controlling Drug Release, Chem. Rev., 2016, 116(4), 2602–2663, DOI:10.1021/acs.chemrev.5b00346.
- P. Sharma, P. Negi and N. Mahindroo, Recent Advances in Polymeric Drug Delivery Carrier Systems, Adv. Polym. Biomed. Appl., 2018, 369–388 Search PubMed.
- K. K. Bawa and J. K. Oh, Stimulus-Responsive Degradable Polylactide-Based Block Copolymer Nanoassemblies for Controlled/Enhanced Drug Delivery, Mol. Pharm., 2017, 14(8), 2460–2474, DOI:10.1021/acs.molpharmaceut.7b00284.
- E. S. Hosseini, S. Dervin, P. Ganguly and R. Dahiya, Biodegradable Materials for Sustainable Health Monitoring Devices, ACS Appl. Bio Mater., 2021, 4(1), 163–194, DOI:10.1021/acsabm.0c01139.
- M. E. Roth, O. Green, S. Gnaim and D. Shabat, Dendritic, Oligomeric, and Polymeric Self-Immolative Molecular Amplification, Chem. Rev., 2016, 116(3), 1309–1352, DOI:10.1021/acs.chemrev.5b00372.
- M. J. Tan, C. Owh, P. L. Chee, A. K. K. Kyaw, D. Kai and X. J. Loh, Biodegradable Electronics: Cornerstone for Sustainable Electronics and Transient Applications, J. Mater. Chem. C, 2016, 4(24), 5531–5558, 10.1039/c6tc00678g.
- X. Peng, K. Dong, Z. Wu, J. Wang and Z. L. Wang, A Review on Emerging Biodegradable Polymers for Environmentally Benign Transient Electronic Skins, J. Mater. Sci., 2021, 56(30), 16765–16789, DOI:10.1007/s10853-021-06323-0.
- X. B. Lu, Y. Liu and H. Zhou, Learning Nature: Recyclable Monomers and Polymers, Chem.–A Eur. J., 2018, 24(44), 11255–11266, DOI:10.1002/chem.201704461.
- M. Hong and E. Y. X. Chen, Chemically Recyclable Polymers: A Circular Economy Approach to Sustainability, Green Chem., 2017, 19(16), 3692–3706, 10.1039/c7gc01496a.
- R. A. Kenley and G. E. Manser, Degradable Polymers. Incorporating a Difunctional Azo Compound into a Polymer Network To Produce Thermally Degradable Polyurethanes, Macromolecules, 1985, 18(2), 127–131, DOI:10.1021/ma00144a002.
- M. A. Ayer, S. Schrettl, S. Balog, Y. C. Simon and C. Weder, Light-Responsive Azo-Containing Organogels, Soft Matter, 2017, 13(22), 4017–4023, 10.1039/c7sm00601b.
- H. Mutlu, C. M. Geiselhart and C. Barner-Kowollik, Untapped Potential for Debonding on Demand: The Wonderful World of Azo-Compounds, Mater. Horizons, 2018, 5(2), 162–183, 10.1039/c7mh00920h.
- J. D. Feist, D. C. Lee and Y. Xia, A Versatile Approach for the Synthesis of Degradable Polymers via Controlled Ring-Opening Metathesis Copolymerization, Nat. Chem., 2022, 14(1), 53–58, DOI:10.1038/s41557-021-00810-2.
- J. M. J. Paulusse, R. J. Amir, R. A. Evans and C. J. Hawker, Free Radical Polymers with Tunable and Selective Bio- and Chemical Degradability, J. Am. Chem. Soc., 2009, 131(28), 9805–9812, DOI:10.1021/ja903245p.
- Y. Tachibana, T. Baba and K. Kasuya, Environmental Biodegradation Control of Polymers by Cleavage of Disulfide Bonds, Polym. Degrad. Stab., 2017, 137, 67–74, DOI:10.1016/j.polymdegradstab.2017.01.003.
- J. Xia, T. Li, C. Lu and H. Xu, Selenium-Containing Polymers: Perspectives toward Diverse Applications in Both Adaptive and Biomedical Materials, Macromolecules, 2018, 51(19), 7435–7455, DOI:10.1021/acs.macromol.8b01597.
- H. R. Kricheldorf, Syntheses of Biodegradable and Biocompatible Polymers by Means of Bismuth Catalysts, Chem. Rev., 2009, 109(11), 5579–5594, DOI:10.1021/cr900029e.
- S. E. Paramonov, E. M. Bachelder, T. T. Beaudette, S. M. Standley, C. C. Lee, J. Dashe and J. M. J. Fréchet, Fully Acid-Degradable Biocompatible Polyacetal Microparticles for Drug Delivery, Bioconjug. Chem., 2008, 19(4), 911–919, DOI:10.1021/bc7004472.
- O. Shelef, S. Gnaim and D. Shabat, Self-Immolative Polymers: An Emerging Class of Degradable Materials with Distinct Disassembly Profiles, J. Am. Chem. Soc., 2021, 143(50), 21177–21188, DOI:10.1021/jacs.1c11410.
- Y. Liang, H. Sun, W. Cao, M. P. Thompson and N. C. Gianneschi, Degradable Polyphosphoramidate via Ring-Opening Metathesis Polymerization, ACS Macro Lett., 2020, 9(10), 1417–1422, DOI:10.1021/acsmacrolett.0c00401.
- C. C. Song, F. S. Du and Z. C. Li, Oxidation-Responsive Polymers for Biomedical Applications, J. Mater. Chem. B, 2014, 2(22), 3413–3426, 10.1039/c3tb21725f.
- L. Wang, K. Zhu, W. Cao, C. Sun, C. Lu and H. Xu, ROS-Triggered Degradation of Selenide-Containing Polymers Based on Selenoxide Elimination, Polym. Chem., 2019, 10(16), 2039–2046, 10.1039/c9py00171a.
- K. A. Miller, E. G. Morado, S. R. Samanta, B. A. Walker, A. Z. Nelson, S. Sen, D. T. Tran, D. J. Whitaker, R. H. Ewoldt and P. V. Braun,
et al., Acid-Triggered, Acid-Generating, and Self-Amplifying Degradable Polymers, J. Am. Chem. Soc., 2019, 141(7), 2838–2842, DOI:10.1021/jacs.8b07705.
- Y. Xu, S. Sen, Q. Wu, X. Zhong, R. H. Ewoldt and S. C. Zimmerman, Base-Triggered Self-Amplifying Degradable Polyurethanes with the Ability to Translate Local Stimulation to Continuous Long-Range Degradation, Chem. Sci., 2020, 11(12), 3326–3331, 10.1039/c9sc06582b.
- G. I. Peterson, D. C. Church, N. A. Yakelis and A. J. Boydston, 1,2-Oxazine Linker As a Thermal Trigger for Self-Immolative Polymers, Polymer, 2014, 55(23), 5980–5985, DOI:10.1016/j.polymer.2014.09.048.
- G. R. Kiel, D. J. Lundberg, E. Prince, K. E. L. Husted, A. M. Johnson, V. Lensch, S. Li, P. Shieh and J. A. Johnson, Cleavable Comonomers for Chemically Recyclable Polystyrene: A General Approach to Vinyl Polymer Circularity, J. Am. Chem. Soc., 2022, 144(28), 12979–12988, DOI:10.1021/jacs.2c05374.
- Y. Lin, T. B. Kouznetsova and S. L. Craig, Mechanically Gated Degradable Polymers, J. Am. Chem. Soc., 2020, 142(5), 2105–2109, DOI:10.1021/jacs.9b13359.
- M. C. Parrott, J. C. Luft, J. D. Byrne, J. H. Fain, M. E. Napier and J. M. Desimone, Tunable Bifunctional Silyl Ether Cross-Linkers for the Design of Acid-Sensitive Biomaterials, J. Am. Chem. Soc., 2010, 132(50), 17928–17932, DOI:10.1021/ja108568g.
- J. Szychowski, A. Mahdavi, J. J. L. Hodas, J. D. Bagert, J. T. Ngo, P. Landgraf, D. C. Dieterich, E. M. Schuman and D. A. Tirrell, Cleavable Biotin Probes for Labeling of Biomolecules via Azide-Alkyne Cycloaddition, J. Am. Chem. Soc., 2010, 132(51), 18351–18360, DOI:10.1021/ja1083909.
- C. M. Bunton, Z. M. Bassampour, J. M. Boothby, A. N. Smith, J. V. Rose, D. M. Nguyen, T. H. Ware, K. G. Csaky, A. R. Lippert and N. V. Tsarevsky,
et al., Degradable Silyl Ether-Containing Networks from Trifunctional Thiols and Acrylates, Macromolecules, 2020, 53(22), 9890–9900, DOI:10.1021/acs.macromol.0c01967.
- M. C. Parrott, M. Finniss, J. C. Luft, A. Pandya, A. Gullapalli, M. E. Napier and J. M. Desimone, Incorporation and Controlled Release of Silyl Ether Prodrugs from PRINT Nanoparticles, J. Am. Chem. Soc., 2012, 134(18), 7978–7982, DOI:10.1021/ja301710z.
- T. Ware, A. R. Jennings, Z. S. Bassampour, D. Simon, D. Y. Son and W. Voit, Degradable, Silyl Ether Thiol-Ene Networks, RSC Adv., 2014, 4(75), 39991–40002, 10.1039/c4ra06997h.
- S. Zhang, X. Q. Xu, S. Liao, Q. Pan, X. Ma and Y. Wang, Controllable Degradation of Polyurethane Thermosets with Silaketal Linkages in Response to Weak Acid, ACS Macro Lett., 2022, 11(7), 868–874, DOI:10.1021/acsmacrolett.2c00204.
- K. E. L. Husted, C. M. Brown, P. Shieh, I. Kevlishvili, S. L. Kristufek, H. Zafar, J. V. Accardo, J. C. Cooper, R. S. Klausen and H. J. Kulik,
et al., Remolding and Deconstruction of Industrial Thermosets via Carboxylic Acid-Catalyzed Bifunctional Silyl Ether Exchange, J. Am. Chem. Soc., 2023, 145(3), 1916–1923, DOI:10.1021/jacs.2c11858.
- K. E. L. Husted, P. Shieh, D. J. Lundberg, S. L. Kristufek and J. A. Johnson, Molecularly Designed Additives for Chemically Deconstructable Thermosets without Compromised Thermomechanical Properties, ACS Macro Lett., 2021, 10(7), 805–810, DOI:10.1021/acsmacrolett.1c00255.
- P. Shieh, W. Zhang, K. E. L. Husted, S. L. Kristufek, B. Xiong, D. J. Lundberg, J. Lem, D. Veysset, Y. Sun and K. A. Nelson,
et al., Cleavable Comonomers Enable Degradable, Recyclable Thermoset Plastics, Nature, 2020, 583(7817), 542–547, DOI:10.1038/s41586-020-2495-2.
-
Y. AlFaraj, S. Mohapatra, P. Shieh, K. Husted, D. Ivanoff, E. Lloyd, J. Cooper, Y. Dai, A. Singhal, J. Moore, et al., A Model Ensemble Approach Enables Data-Driven Property Prediction for Chemically Deconstructable Thermosets in the Low Data Regime, ChemRxiv, 2023, 1–19 Search PubMed.
- P. Shieh, H. V. T. Nguyen and J. A. Johnson, Tailored Silyl Ether Monomers Enable Backbone-Degradable Polynorbornene-Based Linear, Bottlebrush and Star Copolymers through ROMP, Nat. Chem., 2019, 11(12), 1124–1132, DOI:10.1038/s41557-019-0352-4.
- A. M. Johnson, K. E. L. Husted, L. J. Kilgallon and J. A. Johnson, Orthogonally Deconstructable and Depolymerizable Polysilylethers via Entropy-Driven Ring-Opening Metathesis Polymerization, Chem. Commun., 2022, 58(61), 8496–8499, 10.1039/d2cc02718f.
- E. M. Lloyd, J. C. Cooper, P. Shieh, D. G. Ivanoff, N. A. Parikh, E. B. Mejia, K. E. L. Husted, L. C. Costa, N. R. Sottos and J. A. Johnson,
et al., Efficient Manufacture, Deconstruction, and Upcycling of High-Performance Thermosets and Composites, ACS Appl. Eng. Mater., 2023, 1(1), 477–485, DOI:10.1021/acsaenm.2c00115.
- R. A. Smith, G. Fu, O. McAteer, M. Xu and W. R. Gutekunst, Radical Approach to Thioester-Containing Polymers, J. Am. Chem. Soc., 2019, 141(4), 1446–1451, DOI:10.1021/jacs.8b12154.
- E. A. Prebihalo, A. M. Luke, Y. Reddi, C. J. LaSalle, V. M. Shah, C. J. Cramer and T. M. Reineke, Radical Ring-Opening Polymerization of Sustainably-Derived Thionoisochromanone, Chem. Sci., 2023, 5689–5698, 10.1039/d2sc06040j.
- G. Delaittre and L. Barner, The Para-Fluoro-Thiol Reaction as an Efficient Tool in Polymer Chemistry, Polym. Chem., 2018, 9(20), 2679–2684, 10.1039/c8py00287h.
- P. Zhu, W. Meng and Y. Huang, Synthesis and Antibiofouling Properties of Crosslinkable Copolymers Grafted with Fluorinated Aromatic Side Chains, RSC Adv., 2017, 7(6), 3179–3189, 10.1039/C6RA26409C.
- C. Ott, R. Hoogenboom and U. S. Schubert, Post-Modification of Poly(Pentafluorostyrene): A Versatile “Click” Method to Create Well-Defined Multifunctional Graft Copolymers, Chem. Commun., 2008, 30, 3516–3518, 10.1039/b807152g.
- V. Atanasov and J. Kerres, Highly Phosphonated Polypentafluorostyrene, Macromolecules, 2011, 44(16), 6416–6423, DOI:10.1021/ma2011574.
- J. M. Noy, A. K. Friedrich, K. Batten, M. N. Bhebhe, N. Busatto, R. R. Batchelor, A. Kristanti, Y. Pei and P. J. Roth, Para-Fluoro Postpolymerization Chemistry of Poly(Pentafluorobenzyl Methacrylate): Modification with Amines, Thiols, and Carbonylthiolates, Macromolecules, 2017, 50(18), 7028–7040, DOI:10.1021/acs.macromol.7b01603.
- P. Boufflet, A. Casey, Y. Xia, P. N. Stavrinou and M. Heeney, Pentafluorobenzene End-Group as a Versatile Handle for Para Fluoro “Click” Functionalization of Polythiophenes, Chem. Sci., 2017, 8(3), 2215–2225, 10.1039/c6sc04427a.
- S. Agar, E. Baysak, G. Hizal, U. Tunca and H. Durmaz, An Emerging Post-Polymerization Modification Technique: The Promise of Thiol-Para-Fluoro Click Reaction, J. Polym. Sci. Part A Polym. Chem., 2018, 56(12), 1181–1198, DOI:10.1002/pola.29004.
- Y. Pei, J. M. Noy, P. J. Roth and A. B. Lowe, Thiol-Reactive Passerini-Methacrylates and Polymorphic Surface Functional Soft Matter Nanoparticles via Ethanolic RAFT Dispersion Polymerization and Post-Synthesis Modification, Polym. Chem., 2015, 6(11), 1928–1931, 10.1039/c4py01558d.
- J. M. Noy, M. Koldevitz and P. J. Roth, Thiol-Reactive Functional Poly(Meth)Acrylates: Multicomponent Monomer Synthesis, RAFT (Co)Polymerization and Highly Efficient Thiol-Para-Fluoro Postpolymerization Modification, Polym. Chem., 2015, 6(3), 436–447, 10.1039/c4py01238k.
- C. S. Gudipati, C. M. Creenlief, J. A. Johnson, P. Prayoncpan and K. L. Wooley, Hyperbranched Fluoropolymer and Linear Poly(Ethylene Glycol) Based Amphiphilic Crosslinked Networks as Efficient Antifouling Coatings: An Insight into the Surface Compositions, Topographies, and Morphologies, J. Polym. Sci. Part A Polym. Chem., 2004, 42(24), 6193–6208, DOI:10.1002/pola.20466.
- D. Gan, A. Mueller and K. L. Wooley, Amphiphilic and Hydrophobic Surface Patterns Generated from Hyperbranched Fluoropolymer/Linear Polymer Networks: Minimally Adhesive Coatings via the Crosslinking of Hyperbranched Fluoropolymers, J. Polym. Sci. Part A Polym. Chem., 2003, 41(22), 3531–3540, DOI:10.1002/pola.10968.
- P. M. Blackmore and W. J. Feast, Stereoregular Fluoropolymers: 6. The Ring-Opening Polymerization of N-Pentafluorophenylbicyclo[2.2.1]Hept-5-Ene-2,3-Dicarboximide, J. Fluorine Chem., 1988, 40(2–3), 331–347, DOI:10.1016/S0022-1139(00)83072-9.
- A. A. Santiago, J. Vargas, S. Fomine, R. Gavino and M. A. Tlenkopatchev, Polynorbornene with Pentafluorophenyl Imide Side Chain Groups: Synthesis and Sulfonation, J. Polym. Sci. Part A Polym. Chem., 2010, 48, 2925–2933 CrossRef CAS.
- Y. Xia, Y. Li, A. O. Burts, M. F. Ottaviani, D. A. Tirrell, J. A. Johnson, N. J. Turro and R. H. Grubbs, EPR Study of Spin Labeled Brush Polymers in Organic Solvents, J. Am. Chem. Soc., 2011, 133(49), 19953–19959, DOI:10.1021/ja2085349.
- H. V. T. Nguyen, A. Detappe, N. M. Gallagher, H. Zhang, P. Harvey, C. Yan, C. Mathieu, M. R. Golder, Y. Jiang and M. F. Ottaviani,
et al., Triply Loaded Nitroxide Brush-Arm Star Polymers Enable Metal-Free Millimetric Tumor Detection by Magnetic Resonance Imaging, ACS Nano, 2018, 12(11), 11343–11354, DOI:10.1021/acsnano.8b06160.
- M. R. Golder, J. Liu, J. N. Andersen, M. V. Shipitsin, F. Vohidov, H. V. T. Nguyen, D. C. Ehrlich, S. J. Huh, B. Vangamudi and K. D. Economides,
et al., Reduction of Liver Fibrosis by Rationally Designed Macromolecular Telmisartan Prodrugs, Nat. Biomed. Eng., 2018, 2(11), 822–830, DOI:10.1038/s41551-018-0279-x.
- F. Vohidov, J. N. Andersen, K. D. Economides, M. V. Shipitsin, O. Burenkova, J. C. Ackley, B. Vangamudi, H. V. T. Nguyen, N. M. Gallagher and P. Shieh,
et al., Design of BET Inhibitor Bottlebrush Prodrugs with Superior Efficacy and Devoid of Systemic Toxicities, J. Am. Chem. Soc., 2021, 143(12), 4714–4724, DOI:10.1021/jacs.1c00312.
- A. Detappe, H. V. T. Nguyen, Y. Jiang, M. P. Agius, W. Wang, C. Mathieu, N. K. Su, S. L. Kristufek, D. J. Lundberg and S. Bhagchandani,
et al., Molecular Bottlebrush Prodrugs as Mono- and Triplex Combination Therapies for Multiple Myeloma, Nat. Nanotechnol., 2023, 18(2), 184–192, DOI:10.1038/s41565-022-01310-1.
- S. H. Bhagchandani, F. Vohidov, L. E. Milling, E. Y. Tong, C. M. Brown, M. L. Ramseier, B. Liu, T. B. Fessenden, H. V. T. Nguyen and G. R. Kiel,
et al., Engineering Kinetics of TLR7/8 Agonist Release from Bottlebrush Prodrugs Enables Tumor-Focused Immune Stimulation, Sci. Adv., 2023, 9(16), eadg2239, DOI:10.1126/sciadv.adg2239.
- H. Turgut, A. C. Schmidt, P. Wadhwani, A. Welle, R. Müller and G. Delaittre, The Para-Fluoro-Thiol Ligation in Water, Polym. Chem., 2017, 8(8), 1288–1293, 10.1039/c6py02108e.
-
E. P. Serjeant, B. Dempsey, Ionisation constants of organic acids in aqueous solutionPergamon Press, Oxford, 1979 Search PubMed.
- S. Kovačič and C. Slugovc, Ring-Opening Metathesis Polymerisation Derived Poly(Dicyclopentadiene) Based Materials, Mater. Chem. Front., 2020, 4(8), 2235–2255, 10.1039/d0qm00296h.
|
This journal is © The Royal Society of Chemistry 2023 |