DOI:
10.1039/D3SC02709K
(Edge Article)
Chem. Sci., 2023,
14, 12477-12483
Fast and scalable solvent-free access to Lappert's heavier tetrylenes E{N(SiMe3)2}2 (E = Ge, Sn, Pb) and ECl{N(SiMe3)2} (E = Ge, Sn)†
Received
29th May 2023
, Accepted 24th September 2023
First published on 28th September 2023
Abstract
Iconic Lappert's heavier tetrylenes E{N(SiMe3)2}2 (E = Ge (1), Sn (2), Pb (3)) have been efficiently prepared from GeCl2·(1,4-dioxane), SnCl2 or PbCl2 and Li{N(SiMe3)2} via a completely solvent-free one-pot mechanochemical route followed by sublimation. This fast, high-yielding and scalable approach (2 has been prepared in a 100 mmol scale), which involves a small environmental footprint, represents a remarkable improvement over any synthetic route reported over the last five decades, being a so far rare example of the use of mechanochemistry in the realm of main group chemistry. This solventless route has been successfully extended to the preparation of other heavier tetrylenes, such as ECl{N(SiMe3)2} (E = Ge (4), Sn (5)).
Introduction
Over the last two decades the main group arena has experienced a renaissance, from fundamental structural studies, through bonding and reactivity, to applications in catalysis and materials,1,2 and the development of new synthetic approaches has proven fundamental to the advancement of chemistry and its neighbouring fields.3
In this context, mechanochemistry represents an effective alternative to classical solution-based methods since it allows for chemical transformations to proceed with minimal (or no) solvent involvement, which has a profound impact, not only reducing solvent waste, but also eliminating time- and energy-consuming solvent purification and removal steps. Hence, mechanosynthesis has been growing as powerful alternative to solution-based thermochemistry for the last two decades4 in a wide range of fields.5–12 However, it remains underrepresented across the main group element arena.8,13
On the other hand, heavier carbene analogues, also known as heavier tetrylenes (HTs), are currently receiving increasing attention, not only for their rich and unique fundamental chemistry (small molecule activation, insertion into σ-bonds, catalysis, etc.)14,15 but also for their rising use as ligands in coordination chemistry and homogeneous catalysis.16,17 In fact, HT species can be very strong electron-donating ligands,18 stronger than NHCs, being also able to cooperate with the metal in substrate activation processes.19
With these two precedents in mind, we decided to revisit the currently used synthetic routes to E{N(SiMe3)2}2 (E = Ge (1), Sn (2), Pb (3)), reported by both Lappert and Zuckerman (only 2) in 1974.20 These are some of the first HTs ever reported different from the long-known heavier group 14 (Ge, Sn and Pb) dihalides. Notably, compounds 1–3 have an extensive chemistry (e.g., materials chemistry,21a–c metal-free catalysis,21d,e CH activation,21f,g coordination chemistry16n–p,22) as well as being popular precursors to other HTs.15b,m,n,16p,23 As proof of their synthetical value, compounds 1–3 are commercially available from various chemical suppliers (CAS numbers 55290-25-0, 59863-13-7 and 55147-59-6).
In addition to the original reports (in the 70's), several routes have been described for the synthesis of 1–3 (see Table S1 in the ESI†).20,24 In all cases, the solution-based transmetalation process comprises the reaction of Li{N(SiMe3)2} (prepared in situ from nBuLi and HN(SiMe3)2 or commercially obtained) with the corresponding tetrel dichloride (i.e., ECl2, E = Ge, Sn and Pb), followed by one or more purification steps (i.e., solvent evaporation, addition of a different solvent, filtration, solvent evaporation20b,24d and distillation/sublimation;20b filtration, solvent evaporation and distillation/sublimation;24a,c,e or solvent evaporation and distillation/sublimation24b,f) to remove the LiCl produced and other impurities. In addition, these syntheses require environmentally hazardous solvents (e.g., diethyl ether, tetrahydrofuran, hexane, toluene or benzene) that need to be previously dried and deoxygenated (see Fig. 1).
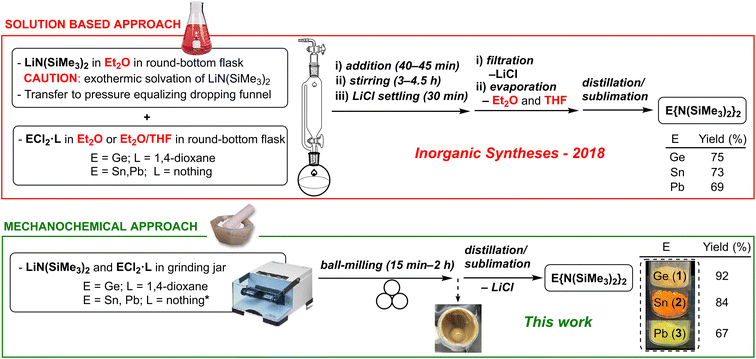 |
| Fig. 1 Conventional solution-based (ref. 24c) vs. mechanochemical syntheses (this work) for compounds 1–3. | |
Herein, five decades after their first report, we demonstrate that Lappert's homoleptic heavier tetrylenes E{N(SiMe3)2}2 (E = Ge (1), Sn (2), Pb (3)) as well as the heteroleptic derivatives ECl{N(SiMe3)2} (E = Ge (4), Sn (5)) can be efficiently prepared in the absence of any solvent using a novel mechanochemical approach (see Fig. 1).
Results and discussion
Synthesis of E{N(SiMe3)2}2 (E = Ge (1), Sn (2) and Pb (3))
Firstly, a 2
:
1 mixture of solid Li{N(SiMe3)2} and GeCl2·(1,4-dioxane) was ball-milled at 30 Hz at room temperature. After 15 min, an aliquot of the crude reaction mixture was analysed by ex situ1H NMR in C6D6, showing almost quantitative formation of compound 1 (Fig. S1;† sharp singlet at 0.33 ppm (ref. 25)). In contrast, under analogous conditions, the reaction of Li{N(SiMe3)2} with SnCl2 afforded a 22
:
78 mixture of 2 and Li{N(SiMe3)2} (determined by the integral ratio of the singlets at 0.30 (ref. 25) and 0.13 (ref. 26) ppm in the 1H NMR spectrum of the crude mixture, Fig. S4†) after 15 min. Given the incomplete conversion, longer reaction times were attempted. However, only a slight increase in the amount of 2 was observed after 16 h (reaching a 24
:
76 ratio between 2 and Li{N(SiMe3)2}; Fig. S6†).
The absence of a 1,4-dioxane solvate molecule in SnCl2 was claimed as responsible for the slow progress observed for the formation of stannylene 2 compared to that of germylene 1, in which one equivalent of 1,4-dioxane is present in the reaction medium. In order to assess if the presence of 1,4-dioxane improved the reaction outcome, the synthesis of 2 was attempted using a minute amount of 1,4-dioxane (η = 4 × 10−4).27 Still, this approach did not produce any improvement after 15 min of ball-milling (Fig. S7†). However, it significantly accelerated the reaction conversion after 5 h, leading to an 86
:
14 mole ratio between 2 and Li{N(SiMe3)2} (Fig. S8†), cf. 24
:
76 mole ratio for neat grinding after 16 h.
Considering the importance of solvent molecules and solvated species in the main-group arena,28,29 together with the concept of “solvate-assisted grinding”, recently proposed by Hanusa et al.,30 we mimicked the solvation conditions existing throughout the formation of 1 by using η-solvate (ηsolv), which we outlined as the formal number of solvate molecules per metal centre. Hence, the synthesis of 2 was carried out by adding one equivalent of 1,4-dioxane to the mixture of reagents (ηsolv = 1).
To our delight, this approach allowed the formation of 2 almost quantitatively after 15 min of ball milling at 30 Hz (an aliquot of the crude was analysed by 1H NMR, showing a sharp singlet at 0.30 ppm; Fig. S9†). The external addition of an amount of liquid additive for 2 equals the formal intrinsic solvation value, determined by using the GeCl2·(1,4-dioxane) starting material, which allowed to mimic the faster reaction kinetics observed for the synthesis of 1. Notably, while ηsolv value remains constant on descending the group, η decreases due to the increased atomic weight of the tetrel element (0.21 and 019, for 1 and 2, respectively). In this manner, ηsolv allows to readily quantify the amount of solvent additives involved when solvated and unsolvated species are directly compared. However, whether 1,4-dioxane increases reaction kinetics via changes in the rheology of the reaction mixture and/or the formation of more reactive solvate species requires further investigation.
To complete the triad, plumbylene 3 was obtained under the same experimental conditions used for 2. Thus, a 2
:
1
:
1 mixture of solid Li{N(SiMe3)2}, PbCl2 and 1,4-dioxane (i.e., 500 µL, ηsolv = 1, which corresponds to η = 0.16) was ball-milled at 30 Hz at room temperature. After 15 min, an aliquot of the crude reaction outcome was analysed by 1H NMR in C6D6, showing the formation of 3 (singlet at 0.25 ppm (ref. 25)) and an unknown species (singlet at 0.28 ppm), exhibiting signals of similar intensity (Fig. S13†). Longer reaction times (45 min) greatly increased the amount of 3 (Fig. S14†), with no further evolution after 2 h of ball-milling (Fig. S15†).
Having developed a new optimised mechanochemical method for the formation of 1, 2 and 3 as major products, these species were isolated by distillation/sublimation of the crude reaction outcomes in yields of 92%, 84% and 67%, respectively, and characterised employing multinuclear NMR (Fig. 2, S2, S3, S10–S12, S16–S18†).25
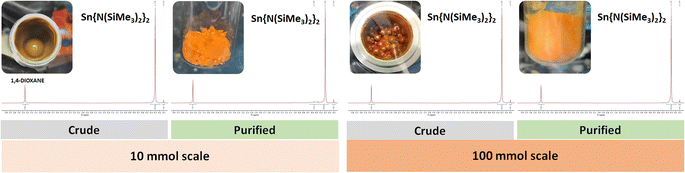 |
| Fig. 2 Side-by-side comparison of the 10 and 100 mmol scale reactions (left and right, respectively) for the synthesis of Sn{N(SiMe3)2}2 (2). The inserts display the crude product obtained in 10 mL and the 250 mL stainless steel jars, and the resulting isolated product stored in a 20 mL vial and a 100 mL Schlenk tube for the small and large scales, respectively. See ESI† for expanded NMRs. | |
Comparative assessment of the environmental footprint
Sustainability and environmental footprint has become increasingly important in synthetic chemistry.31–33 However, detailed comparisons between mechanochemical and conventional solution-based methodologies remain rare, especially for the chemistry of main group elements.34,35 Therefore, we compared our approach to compounds 1–3 with a contrasted solution-based synthetic route published recently (2018) in Inorganic Syntheses.24c Qualitatively, a step-by-step comparison of the different steps required to prepare 1–3 indicates the advantages of the mechanochemical solventless route (fewer steps, less complexity, fewer hazards, shorter times, less waste management and less energy consumption to achieve comparable or better product yields).
In a quantitative manner, a green chemistry metrics (GCM)36 approach was used for comparison. The environmental factor (E-factor),37 atom economy (AE), process mass intensity (PMI), and reaction mass efficiency (RME) for both synthetic routes were calculated (see Table 1; and ESI†). Under mechanochemical conditions, lower E-factors were observed (0.57, 0.70 and 1.06 vs. 2.53, 1.48 and 1.56) even though the energy consumption of the solvent purification and deoxygenation steps of the solution route was not taken into account. In the case of 2 and 3, if the liquid additive used is considered as a solvent, the E-factor is further reduced (from 0.70 and 1.06 to 0.45 and 0.79, respectively). The PMI for the mechanochemical synthesis showed much lower values (1.57, 1.70 and 2.06) than their solution-based counterparts (18.07, 12.42 and 12.62). However, since both approaches use the same reagents, RME and AE values are comparable (depending on the yield of isolated products) and equal, respectively, for both processes.
Table 1 Comparison of green metrics between mechanochemical and a model conventional solution-based route for 1–3
Compound |
E-factor |
PMI |
RME (%) |
AE (%) |
Yield (%) |
Treating 1,4-dioxane as a “solvent”.
|
Mechanochemical route (this work)
|
1
|
0.57 |
1.57 |
63.9 |
69.4 |
92 |
2
|
0.70/0.45a |
1.70 |
70.4 |
83.8 |
84 |
3
|
1.06/0.79a |
2.06 |
57.7 |
86.2 |
67 |
![[thin space (1/6-em)]](https://www.rsc.org/images/entities/char_2009.gif) |
Solution-based route24c
|
1
|
2.53 |
18.07 |
52.1 |
69.4 |
75 |
2
|
1.48 |
12.42 |
61.2 |
83.8 |
73 |
3
|
1.56 |
12.62 |
59.4 |
86.2 |
69 |
Upscaled synthesis of Sn{N(SiMe3)2}2 (2)
Currently, one of the major challenges for the broader adoption of mechanochemical methodologies is its ability to be implemented at large preparative scales.38 This is especially needed in the main group field, where examples of compounds, complexes and materials at large scales are scarce.35
Based on this, we set off to upscale the route to compound 2, which is the most used precursor of the series to prepare other HTs (stannylenes in this case),15b,m,n,16p,23 using a scale-up batch approach employing a planetary ball mill. A 250 mL jar was used to produce 2 in a 100 mmol scale by milling SnCl2 (18.96 g, 100 mmol) with Li{N(SiMe3)2} (33.46 g, 200 mmol) in the presence of 1,4-dioxane (10 mL, η = 0.19 and ηsolv = 1, respectively) for 30 min. To our delight, the reaction was successfully scaled 10-fold, maintaining an almost quantitative conversion as illustrated by the 1H NMR of the crude products (see Fig. 2). The larger scale preparation allowed the isolation of 2 by distillation (see Fig. S20–S22†) in a remarkable 88% yield (38.6 g), cf. 73% yield for the 100 mmol scale solution-based synthetic route published in Inorganic Syntheses,24c demonstrating the suitability of the solventless designed methodology for multigram preparations.
Mechanochemical derivatisation of Lappert's heavier tetrylenes: synthesis of ECl{N(SiMe3)2} (E = Ge (4) and Sn (5))
The successful solventless synthesis of 1–3 prompted us to extent our mechanochemical approach to the preparation of other heavier tetrylenes, in particular, the heteroleptic derivatives ECl{N(SiMe3)2} (E = Ge (4), Sn (5) and Pb (6)).
Chloroamido-stannylene 5 was first isolated by both Lappert and Zuckerman in 1974 (ref. 20a and c) as a white solid in ca. 50% yield (latter improved to 87% in 1991).39 Compound 5 is conventionally obtained following a solution-based40 redistribution reaction between SnCl2 and 2 (ref. 20a and 39) or a 1
:
1 transmetalation reaction of SnCl2 and Li{N(SiMe3)2}.20a,c
Chlorogermylene 4 has never been isolated, although it has been proposed as a reaction intermediate during the thermal decomposition of germacyclopentenes as a route to germaisonitriles.41
Lastly, in contrast to the lighter Ge and Sn counterparts, which identities have been fully confirmed39 and proposed using photoelectron spectroscopy,41 respectively, chloroplumbylene 6 is unknown, to the best of our knowledge.
We chose the synthesis of 4–6 (Scheme 1) by the redistribution approach because no reaction subproducts were expected as well as it will demonstrate the viability of the mechano-derivatisation of compounds 1–3, which will prove the broad applicability of mechanochemistry towards the synthesis and derivatisation of tetrylene species.
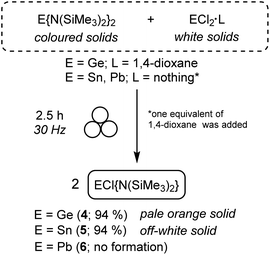 |
| Scheme 1 Mechanochemical reactions of homoleptic E{N(SiMe3)2}2 (E = Ge (1), Sn (2) and Pb (3)) with the corresponding tetrel dihalide to produce heteroleptic ECl{N(SiMe3)2}2 (E = Ge (4) and Sn (5)). | |
Therefore, a 1
:
1 mixture of solid 1, 2 or 3 and the corresponding tetrel dihalide ECl2·L (E = Ge, Sn, Pb; L = 1,4-dioxane for E = Ge; nothing for E = Sn, Pb), in the presence of one equivalent of 1,4-dioxane for E = Sn, Pb (ηsolv = 1), was ball-milled at 30 Hz at room temperature. After 2.5 h, aliquots of the crude reaction mixtures were analysed by ex situ1H NMR in C6D6. For E = Ge and Sn, these spectra displayed sharp singlets at different chemical shifts from those of 1 and 2, which had entirely disappeared, indicating the formation of 4 (Fig. S23†) and 5 (Fig. S26†), respectively, as the major reaction products. Both compounds were isolated in 94% yield after evacuating the small amount of 1,4-dioxane present in the reaction medium (see Fig. S24 and S25 for 4 and S27–S29† for 5). The NMR data of 5 is consistent with that reported in the literature,39,42 being the 119Sn chemical shift at 124 ppm (Fig. S29†) particularly informative (note that the 119Sn NMR spectrum of 2 shows a singlet at 769 ppm; Fig. S12†).
In contrast, for the heavier tetrel counterpart (E = Pb), no reaction took place after 2.5 h, with homoleptic 3 being the only species observed by NMR analysis (Fig. S30-top†). An alternative direct mechanochemical synthesis of heteroleptic 6 was attempted by the 1
:
1 reaction of PbCl2 and Li{N(SiMe3)2}. Unfortunately, the 1
:
1 reaction only led to the formation of 3 (Fig. S30-bottom†) and unreacted PbCl2. The presence of unreacted PbCl2 was evidenced by the existence of a large amount of unsoluble white solid in C6D6.
The efficient transformation of the homoleptic diamido-HTs 1 and 2 to the heteroleptic chloroamido-HTs 4 and 5, respectively, encouraged us to seek for further mechano-derivatisations that could show the full potential of mechanochemical approaches in main group chemistry. In this context, since chloroamidostannylene 5 has been utilised in several reports as a precursor to prepare other HTs42,43 employing conventional solution-based protocols; as a proof of concept, we set out to mechanochemically synthesise chloroguanidinatostannylene SnCl[iPrNC{N(SiMe3)2}NiPr] (7), previously carried out in diethylether by Růžička et al.,43j by reacting 5 with the carbodiimide iPrNCNiPr. To our delight, 20 min of ball-milling at 30 Hz of a 1
:
1
:
1 mixture of 5, iPrNCNiPr and 1,4-dioxane led to the nearly quantitative formation of 7 (Fig. S31†), which was isolated in 72% yield (see Fig. S32–S34†).44
Conclusions
In summary, a novel mechanochemical approach has been used to prepare heavier tetrylenes, allowing the efficient isolation of Lappert's diamidogermylene 1, -stannylene 2 and -plumbylene 3 and their subsequent transformation to the chloroamidogermylene (4) and -stannylene (5) derivatives. Additionally, further mechano-derivatisations are possible (synthesis of 7). These reactions can be readily upscaled, as demonstrated by the preparation of a 100 mmol batch of Sn{N(SiMe3)2}2 (2) in 88% yield.
The solvent-free routes reported in this study surpass all of the solution-based methods reported for their synthesis in the past five decades, as they deliver a rapid, high-yielding, and environmentally benign processes that represent a milestone in the development of mechanochemical methods in the main group arena. The efficient syntheses of 1–5 and 7 anticipate that mechanochemistry holds great potential for a more sustainable synthesis of other homoleptic (i.e., amino-/alkyl-/aryl-/phophino-) and heteroleptic HTs, a field that currently remains unexplored.
We hope our manuscript encourages more main group and synthetic chemists to incorporate mechanochemical methodologies in their synthetic toolbox throughout their studies.
Data availability
The associated ESI file† contains the data supporting this article (synthetic procedures, characterization data, NMR spectra, green metrics calculations).
Author contributions
R. G.-S. and J. F. R. performed the experimental and characterization activities and wrote the draft of the ESI.† The work was conceived by F. G. and P. G.-Á. and supervised by F. G., P. G.-Á. and J. A. C. The manuscript text and the final version of the ESI file† were written and revised by F. G., P. G.-Á. and J. A. C.
Conflicts of interest
There are no conflicts to declare.
Acknowledgements
This work has been supported by research grants obtained from Agencia Estatal de Investigación (PID2019-104652GB-I00, PID2021-127407NB-I00, and RED2022-134074-T) and Fundación para el Fomento en Asturias de la Investigación Científica Aplicada y la Tecnología (FICYT) through the Margarita Salas Senior Program (AYUD/2021/59709). F. G. would like to thank Monash University for an affiliate position. The authors also acknowledge the technical support provided by Servicios Científico-Técnicos de la Universidad de Oviedo.
Notes and references
- S. Aldrich and C. Jones, Chem. Soc. Rev., 2016, 45, 763–764 RSC and articles cited therein..
- P. P. Power, Nature, 2010, 463, 171–177 CrossRef CAS.
- T. Chivers and J. Konu, Comments Inorg. Chem., 2009, 30, 131–176 CrossRef CAS.
-
(a) J.-L. Do and T. Friščić, ACS Cent. Sci., 2017, 3, 13–19 CrossRef CAS;
(b) S. L. James, C. J. Adams, C. Bolm, D. Braga, P. Collier, T. Friščić, F. Grepioni, K. D. Harris, G. Hyett and W. Jones, Chem. Soc. Rev., 2012, 41, 413–447 RSC;
(c) T. Friščić, C. Mottillo and H. M. Titi, Angew. Chem., Int. Ed., 2020, 59, 1030–1041 CrossRef.
-
(a) F. Cuccu, L. De Luca, F. Delogu, E. Colacino, N. Solin, R. Mocci and A. Porcheddu, ChemSusChem, 2022, 15, e202200362 CrossRef CAS PubMed;
(b) D. Virieux, F. Delogu, A. Porcheddu, F. García and E. Colacino, J. Org. Chem., 2021, 86, 13885–13894 CrossRef CAS PubMed;
(c) J. G. Hernández and C. Bolm, J. Org. Chem., 2017, 82, 4007–4019 CrossRef;
(d) V. Declerck, P. Nun, J. Martinez and F. Lamaty, Angew. Chem., Int. Ed., 2009, 48, 9318–9321 CrossRef CAS PubMed.
-
(a) D. Tan, L. Loots and T. Friščić, Chem. Commun., 2016, 52, 7760–7781 RSC;
(b) P. Ying, J. Yu and W. Su, Adv. Synth. Catal., 2021, 363, 1246–1271 CrossRef CAS;
(c) L. Konnert, B. Reneaud, R. Marcia de Figueiredo, J.-M. Campagne, F. Lamaty, J. Martinez and E. Colacino, J. Org. Chem., 2014, 79, 10132–10142 CrossRef CAS PubMed;
(d) O. Galant, G. Cerfeda, A. S. McCalmont, S. L. James, A. Porcheddu, F. Delogu, D. E. Crawford, E. Colacino and S. Spatari, ACS Sustainable Chem. Eng., 2022, 10, 1430–1439 CrossRef CAS.
-
(a) N. R. Rightmire and T. P. Hanusa, Dalton Trans., 2016, 45, 2352–2362 RSC;
(b)
F. Leon and F. Garcia, Comprehensive Coordination Chemistry III, 2021, vol. 9, pp. 620–679 Search PubMed.
-
(a) A. A. Gečiauskaitė and F. García, Beilstein J. Org. Chem., 2017, 13, 2068–2077 CrossRef;
(b) D. Tan and F. García, Chem. Soc. Rev., 2019, 48, 2274–2292 RSC.
-
(a) D. Chen, J. Zhao, P. Zhang and S. Dai, Polyhedron, 2019, 162, 59–64 CrossRef CAS;
(b) T. Stolar and K. Užarević, CrystEngComm, 2020, 22, 4511–4525 RSC;
(c) T. Friščić, J. Mater. Chem., 2010, 20, 7599–7605 RSC;
(d) P. A. Julien, C. Mottillo and T. Friščić, Green Chem., 2017, 19, 2729–2747 RSC.
- V. K. Portnoi, V. A. Leonov, S. E. Filippova, A. I. Logacheva, A. G. Beresnev and I. M. Razumovskii, Inorg. Mater., 2016, 52, 895–901 CrossRef CAS.
-
(a) B. Szczesniak, S. Borysiuk, J. Choma and M. Jaroniec, Mater. Horiz., 2020, 7, 1457–1473 RSC;
(b) T. F. Grigorieva, A. P. Barinova and N. Z. Lyakhov, J. Nanopart. Res., 2003, 5, 439–453 CrossRef CAS.
-
(a) D. Braga, L. Maini and F. Grepioni, Chem. Soc. Rev., 2013, 42, 7638–7648 RSC;
(b) Z. X. Ng, D. Tan, T. Wei Liang, F. Leon, X.-Y. Shi, Y. Sim, Y. Li, R. Ganguly, Z. Yanli, M. Sharmarke and F. García, Angew. Chem., Int. Ed., 2021, 60, 17481–17490 CrossRef CAS PubMed;
(c) M. M. Haskins and M. J. Zaworotko, Cryst. Growth Des., 2021, 21, 4141–4150 CrossRef CAS PubMed.
-
(a) D. Jędrzkiewicz, J. Mai, J. Langer, Z. Mathe, N. Patel, S. DeBeer and S. Harder, Angew. Chem., Int. Ed., 2022, 61, e202200511 CrossRef PubMed;
(b) J. Wang, R. Ganguly, L. Yongxin, J. Díaz, H. S. Soo and F. García, Dalton Trans., 2016, 45, 7941–7946 RSC;
(c) N. R. Rightmire, T. P. Hanusa and A. L. Rheingold, Organometallics, 2014, 33, 5952–5955 CrossRef CAS;
(d) R. F. Koby, T. P. Hanusa and N. D. Schley, J. Am. Chem. Soc., 2018, 140, 15934–15942 CrossRef CAS PubMed;
(e) R. F. Koby, A. M. Doerr, N. R. Rightmire, N. D. Schley, B. K. Long and T. P. Hanusa, Angew. Chem., Int. Ed., 2020, 59, 9542–9548 CrossRef CAS PubMed;
(f) I. R. Speight, S. C. Chmely, T. P. Hanusa and A. L. Rheingold, Chem. Commun., 2019, 55, 2202–2205 RSC;
(g) I. R. Speight and T. P. Hanusa, Molecules, 2020, 25, 570 CrossRef CAS PubMed;
(h) Y. Sim, D. Tan, R. Ganguly, Y. Li and F. García, Chem. Commun., 2018, 54, 6800–6803 RSC;
(i) Y. Sim, Y.-X. Shi, R. Ganguly, Y. Li and F. García, Chem.–Eur. J., 2017, 47, 11279–11285 CrossRef;
(j) P. Gao, J. Jiang, S. Maeda, K. Kubota and H. Ito, Angew. Chem., Int. Ed., 2022, 61, e202207118 CrossRef CAS PubMed;
(k) R. Takahashi, A. Hu, P. Gao, Y. Gao, Y. Pang, T. Seo, J. Jiang, S. Maeda, H. Takaya, K. Kubota and H. Ito, Nat. Commun., 2021, 12, 6691 CrossRef CAS PubMed;
(l) Y.-X. Shi, K. Xu, J. Clegg, R. Ganguly, H. Hirao, T. Friscic and F. García, Angew. Chem., Int. Ed., 2016, 55, 12736–12740 CrossRef CAS PubMed;
(m) R. F. Koby, A. M. Doerr, N. R. Rightmire, N. D. Schley, W. W. Brennessel, B. K. Long and T. P. Hanusa, Chem.–Eur. J., 2021, 27, 8195–8202 CrossRef CAS PubMed;
(n) R. F. Koby, N. D. Schley and T. P. Hanusa, Angew. Chem., Int. Ed., 2021, 60, 21174–21178 CrossRef CAS PubMed.
- Selected recent reviews on general chemistry of HTs (they might include coordination chemistry):
(a) S. Yao, A. Saddington, Y. Xiong and M. Driess, Acc. Chem. Res., 2023, 56, 475–488 CrossRef CAS PubMed;
(b) Y. Zhang, L. Wu and H. Wang, Coord. Chem. Rev., 2023, 477, 214942–214961 CrossRef CAS;
(c) L. Wang, Y. Li, Z. Li and M. Kira, Coord. Chem. Rev., 2022, 457, 214413–214431 CrossRef CAS;
(d) S. Yao, Y. Xiong, A. Saddington and M. Driess, Chem. Commun., 2021, 57, 10139–10153 RSC;
(e) S. Fujimori and S. Inoue, Eur. J. Inorg. Chem., 2020, 33, 3131–3142 CrossRef PubMed;
(f) S. Khan and H. W. Roesky, Chem.–Eur. J., 2019, 25, 1636–1648 CrossRef CAS PubMed;
(g) S. S. Sen and H. W. Roesky, Chem. Commun., 2018, 54, 5046–5057 RSC;
(h) N. Muthukumaran, K. Velappan, K. Gour and G. Prabusankar, Coord. Chem. Rev., 2018, 377, 1–43 CrossRef CAS;
(i) T. J. Hadlington, M. Driess and C. Jones, Chem. Soc. Rev., 2018, 47, 4176–4197 RSC;
(j) R. Tacke and T. Ribbeck, Dalton Trans., 2017, 46, 13628–13659 RSC.
- Selected reviews on general chemistry of HTs (they might include coordination chemistry):
(a) E. Rivard, Chem. Soc. Rev., 2016, 45, 989–1003 RSC;
(b) C. Marschner, Eur. J. Inorg. Chem., 2015, 23, 3805–3820 CrossRef;
(c) G. Prabusankar, A. Sathyanarayana, P. Suresh, C. N. Babu, K. Srinivas and B. P. R. Metla, Coord. Chem. Rev., 2014, 269, 96–133 CrossRef CAS;
(d) K. Izod, Coord. Chem. Rev., 2013, 257, 924–945 CrossRef CAS;
(e) Y. Xiong, S. Yao and M. Driess, Angew. Chem., Int. Ed., 2013, 52, 4302–4311 CrossRef CAS PubMed;
(f) R. S. Ghadwal, R. Azhakar and H. W. Roesky, Acc. Chem. Res., 2013, 46, 444–456 CrossRef CAS PubMed;
(g) S. K. Mandal and H. W. Roesky, Acc. Chem. Res., 2012, 45, 298–307 CrossRef CAS PubMed;
(h) M. Asay, C. Jones and M. Driess, Chem. Rev., 2011, 111, 354–396 CrossRef CAS PubMed;
(i) S. Yao, Y. Xiong and M. Driess, Organometallics, 2011, 30, 1748–1767 CrossRef CAS;
(j) S. K. Mandal and H. W. Roesky, Chem. Commun., 2010, 46, 6016–6041 RSC;
(k) M. Kira, Chem. Commun., 2010, 46, 2893–2903 RSC;
(l)
V. Y. Lee and A. Sekiguchi, in Organometallic Compounds of Low Coordinate Si, Ge, Sn and Pb: From Phantom Species to Stable Compounds, Wiley-VCH, Chichester, UK, 2010 CrossRef;
(m) Y. Mizuhata, T. Sasamori and N. Tokitoh, Chem. Rev., 2009, 109, 3479–3511 CrossRef CAS PubMed;
(n) A. V. Zabula and F. E. Hahn, Eur. J. Inorg. Chem., 2008, 33, 5165–5179 CrossRef.
- For reviews more focused on the coordination chemistry of HTs, see:
(a) J. A. Cabeza and P. García-Álvarez, Chem.–Eur. J., 2023, 29, e202203096 CrossRef CAS PubMed;
(b) V. L. Lee, Eur. J. Inorg. Chem., 2022, e202200175 CrossRef CAS;
(c) R. J. Somerville and J. Campos, Eur. J. Inorg. Chem., 2021, 3488–3498 CrossRef CAS PubMed;
(d) M. Gosh and S. Khan, Dalton Trans., 2021, 50, 10674–10688 RSC;
(e) J. A. Cabeza, P. García-Álvarez and C. J. Laglera-Gándara, Eur. J. Inorg. Chem., 2020, 784–795 CrossRef CAS;
(f) M. Saito, Acc. Chem. Res., 2018, 51, 160–169 CrossRef CAS PubMed;
(g) M. C. Lipke, A. L. Liberman-Martin and T. D. Tilley, Angew. Chem., Int. Ed., 2017, 56, 2260–2294 CrossRef CAS PubMed;
(h) J. Y. Corey, Chem. Rev., 2016, 116, 11291–11435 CrossRef CAS PubMed;
(i) L. Alvarez-Rodriguez, J. A. Cabeza, P. Garcia-Alvarez and D. Polo, Coord. Chem. Rev., 2015, 300, 1–28 CrossRef CAS;
(j) J. Baumgartner and C. Marschner, Rev. Inorg. Chem., 2014, 34, 119–152 CAS;
(k) B. Blom, M. Stoelzel and M. Driess, Chem.–Eur. J., 2013, 19, 40–62 CrossRef CAS PubMed;
(l) R. Waterman, P. G. Hayes and T. D. Tilley, Acc. Chem. Res., 2007, 40, 712–719 CrossRef CAS PubMed;
(m) M. Okazaki, H. Tobita and H. Ogino, Dalton Trans., 2003, 493–506 RSC;
(n) M. F. Lappert and R. S. Rowe, Coord. Chem. Rev., 1990, 100, 267–292 CrossRef CAS;
(o) W. Petz, Chem. Rev., 1986, 86, 1019–1047 CrossRef CAS;
(p) M. F. Lappert and P. P. Power, J. Chem. Soc., Dalton Trans., 1985, 51–57 RSC.
- For a review more focused on catalysis with HT-metal complexes, see: Y.-P. Zhou and M. Driess, Angew. Chem., Int. Ed., 2019, 58, 3715–3728 CrossRef CAS PubMed.
- See, for example:
(a) Z. Benedek and T. Szilvási, Organometallics, 2017, 36, 1591–1600 CrossRef CAS;
(b) Z. Benedek and T. Szilvási, RSC Adv., 2015, 5, 5077–5086 RSC.
- See, for example:
(a) Ref. 16c;
(b) Y.-P. Zhou, S. Raoufmoghaddam, T. Szilvási and M. Driess, Angew. Chem., Int. Ed., 2016, 55, 12868–12872 CrossRef CAS PubMed;
(c) D. Gallego, A. Bruck, E. Irran, F. Meier, M. Kaupp, M. Driess and J. F. Hartwig, J. Am. Chem. Soc., 2013, 135, 1561–15626 CrossRef PubMed;
(d) B. Blom, S. Enthaler, S. Inoue, E. Irran and M. Driess, J. Am. Chem. Soc., 2013, 135, 6703–6713 CrossRef CAS PubMed;
(e) M. E. Fasulo, M. C. Lipke and T. D. Tilley, Chem. Sci., 2013, 4, 3882–3887 RSC;
(f) E. Calimano and T. D. Tilley, Organometallics, 2010, 29, 1680–1692 CrossRef CAS;
(g) E. Calimano and T. D. Tilley, J. Am. Chem. Soc., 2009, 131, 11161–11173 CrossRef CAS PubMed;
(h) K. E. Litz, J. E. Bender IV, J. W. Kampf and M. M. B. Holl, Angew. Chem., Int. Ed. Engl., 1997, 36, 496–498 CrossRef CAS.
-
(a) D. H. Harris and M. F. Lappert, J. Chem. Soc., Chem. Commun., 1974, 895–896 RSC;
(b) M. J. S. Gynane, D. H. Harris, M. F. Lappert, P. P. Power, P. Rivière and M. Rivière-Baudet, J. Chem. Soc., Dalton Trans., 1977, 2004–2009 RSC;
(c) C. D. Schaeffer and J. J. Zuckerman, J. Am. Chem. Soc., 1974, 96, 7160–7162 CrossRef CAS.
- See, for example:
(a) M. S. Seifner, F. Biegger, A. Lugstein, J. Bernardi and S. Barth, Chem. Mater., 2015, 27, 6125–6130 CrossRef CAS;
(b) M. R. Buck, A. J. Biacchi, E. J. Popczun and R. E. Schaak, Chem. Mater., 2013, 25, 2163–2171 CrossRef CAS;
(c) B. Hernandez-Sanchez, T. J. Boyle, H. D. Pratt, M. A. Rodriguez, L. N. Brewer and D. R. Dunphy, Chem. Mater., 2008, 20, 6643–6656 CrossRef CAS PubMed;
(d) S. Kobayashi, S. Iwate and S. Shoda, Acta Polym., 1995, 46, 471–475 CrossRef CAS;
(e) T. Xing, T. J. Prior and C. Redshaw, Dalton Trans., 2021, 50, 15140–15152 RSC;
(f) K. A. Miller, J. M. Bartolin, R. M. O'Neill, R. D. Sweeder, T. M. Owens, J. W. Kampf, M. M. Banaszak Holl and N. J. Wells, J. Am. Chem. Soc., 2003, 125, 8986–8987 CrossRef CAS PubMed;
(g) J. M. Bartolin, A. Kavara, J. W. Kampf and M. M. Banaszak Holl, Organometallics, 2006, 25, 4738–4740 CrossRef CAS.
- See, for example:
(a) N. Hidalgo, S. Bajo, J. J. Moreno, C. Navarro-Gilabert, B. Q. Mercado and J. Campos, Dalton Trans., 2019, 48, 9127–9138 RSC;
(b) J. A. Cabeza, J. M. Fernández-Colinas, P. García-Álvarez and D. Polo, Inorg. Chem., 2012, 51, 3896–3903 CrossRef CAS;
(c) J. A. Cabeza, P. García-Álvarez and D. Polo, Inorg. Chem., 2012, 51, 2569–2576 CrossRef CAS PubMed;
(d) Z. T. Cygan, J. W. Kampf and M. M. Banaszak Holl, Inorg. Chem., 2003, 42, 7219–7226 CrossRef CAS PubMed;
(e) K. E. Litz, J. W. Kampf and M. M. Banaszak Holl, J. Am. Chem. Soc., 1998, 120, 7484–7492 CrossRef CAS;
(f) K. E. Litz, J. E. Bender IV, J. W. Kampf and M. M. Banaszak Holl, Angew. Chem., Int. Ed., 1997, 36, 496–498 CrossRef CAS;
(g) S. M. Hawkins, P. B. Hitchcock, M. F. Lappert and A. K. Rai, J. Chem. Soc. Chem. Commun., 1986, 1689–1690 RSC.
- See, for example:
(a) P. Sikora, R. Naumann, C. Förster and K. Heinze, Chem. Sci., 2023, 14, 2489–2500 RSC;
(b) Y. K. Loh, L. Ying, M. Á. Fuentes, D. C. H. Do and S. Aldridge, Angew. Chem., Int. Ed., 2019, 58, 4847–4851 CrossRef CAS PubMed;
(c) J. M. Stauber, P. Müller, Y. Dai, G. Wu, D. G. Nocera and C. C. Cummins, Chem. Sci., 2016, 7, 6928–6933 RSC;
(d) I. Orbjartel, N. A. Pott, M. John and D. Stalke, Organometallics, 2010, 29, 5670–5675 CrossRef;
(e) A. Murso, M. Straka, M. Kaupp, R. Bertermann and D. Stalke, Organometallics, 2005, 24, 3576–3578 CrossRef CAS;
(f) A. V. Zabula, T. Pape, A. Hepp, F. M. Schappacher, U. C. Rodewald, R. Pöttgen and F. E. Hahn, J. Am. Chem. Soc., 2008, 130, 5648–5649 CrossRef CAS PubMed;
(g) T. Gans-Eichler, D. Gudat and M. Nieger, Angew. Chem., Int. Ed., 2002, 41, 1888–1891 CrossRef CAS.
- See, for example:
(a) C. D. Schaeffer Jr, L. K. Myers, S. M. Coley, J. C. Otter and C. H. Yoder, J. Chem. Educ., 1990, 67, 347–349 CrossRef;
(b) T. Heidemann and S. Mathur, Eur. J. Inorg. Chem., 2014, 506–510 CrossRef CAS;
(c) A. J. Veinot, D. L. Stack, J. A. C. Clyburne and J. D. Masuda, Inorg. Synth., 2018, 37, 26–31 Search PubMed;
(d) M. Fischer, M. M. D. Roy, L. L. Wales, M. A. Ellwanger, A. Heilmann and S. Aldridge, J. Am. Chem. Soc., 2022, 144, 8908–8913 CrossRef CAS;
(e) G. Bačić, D. Zanders, B. Mallick, A. Devi and S. T. Barry, Inorg. Chem., 2018, 57, 8218–8226 CrossRef PubMed;
(f) A. Herve, A. L. Rodriguez and E. Fouqet, J. Org. Chem., 2005, 70, 1953–1956 CrossRef CAS PubMed.
- The NMR data reported for compounds 1–3 in the Inorganic Syntheses method (ref. 24c) has been used as reference.
- A 1H-NMR spectrum of a pure sample of Li{N(SiMe3)} in C6D6 is shown in Fig. S5,† showing a singlet at 0.13 ppm.
- It is well known that the use of liquid additives accelerates the solid-state grinding process, mechanochemical technique known as liquid-assisted grinding (LAG). The LAG parameter (η), defined as the ratio of liquid (in µL) to the combined weights of solid reactants (in mg), permits a systematic comparison of the various grinding techniques (e.g., η = 0, neat grinding; <1, LAG; ∼2–12, paste/slurry; >12, solution)
(a) G. A. Bowmaker, Chem. Commun., 2013, 49, 334–348 RSC;
(b) T. Friščić and W. Jones, Cryst. Growth Des., 2009, 9, 1621–1637 CrossRef;
(c) T. Friščić, S. L. Childs, S. A. A. Rizvi and W. Jones, CrystEngComm, 2009, 11, 418–426 RSC.
- For reviews on main group chemistry see:
(a) R. Melen, Science, 2019, 363, 479–484 CrossRef CAS PubMed;
(b) D. Bourissou, Chem. Rev., 2019, 119, 8229–8230 CrossRef CAS PubMed;
(c) L. Zhao, S. Pan, N. Holzmann, P. Schwerdtfeger and G. Frenking, Chem. Rev., 2019, 119, 8781–8845 CrossRef CAS PubMed.
-
A. G. Massey, Main Group Chemistry, Wiley, 2nd edn, 2000 Search PubMed.
- H. P. DeGroot and T. P. Hanusa, Organometallics, 2021, 40, 3516–3525 CrossRef CAS.
-
P. T. Anastas and J. C. Warner, Green Chemistry: Theory and Practice, Oxford University Press, New York, 1998, p. 30 Search PubMed.
- “United Nations Sustainable Development Goals,” can be found under https://sdgs.un.org/goals.
- “Chemistry & Sustainable Development Goals,” can be found under https://www.acs.org/content/acs/en/sustainability/chemistry-sustainable-development-goals.html.
- N. Fantozzi, J.-N. Volle, A. Porcheddu, D. Virieux, F. García and E. Colacino, Chem. Soc. Rev., 2023 10.1039/D2CS00997H , advance article..
-
(a) V. K. Singh, A. Chamberlain-Clay, H. C. Ong, F. León, G. Hum, M. Y. Par, P. Daley-Dee and F. García, ACS Sustainable Chem. Eng., 2021, 9, 1152–1160 CrossRef CAS;
(b) F. Leon, C. Li, J. F. Reynes, V. K Singh, X. Lian, H. C. Ong, G. Hum, H. Sun and F. García, Faraday Discuss., 2023, 241, 63–78 RSC.
-
A. Lapkin and D. J. C. Constable, Green Chemistry Metrics: Measuring and Monitoring Sustainable Processes, 2008, Print ISBN: 9781405159685, Online ISBN: 9781444305432 Search PubMed.
- E. Sheldon, Green Chem., 2007, 9, 1273–1283 RSC.
-
(a) E. Colacino, V. Isoni, D. Crawford and F. García, Trends Chem., 2021, 3, 335–339 CrossRef CAS;
(b) J. F. Reynes, V. Isoni and F. García, Angew. Chem., Int. Ed., 2023, e202300819, DOI:10.1002/anie.202300819.
- R. W. Chorley, P. B. Hitchock, B. S. Jolly, M. F. Lappert and G. A. Lawless, J. Chem. Soc., Chem. Commun., 1991, 1302–1303 RSC.
- No detailed experimental procedure is given for the synthesis of SnCl{N(SiMe3)2} (5) in ref. 20a and c. A solution-based approach is assumed considering further information provided shortly after (see: P. J. Davidson, D. H. Harris and M. F. Lappert, J. Chem. Soc., Dalton Trans., 1976, 2268–2274 RSC ) regarding reactivity studies of 5 prepared in situ.
- S. Foucat, T. Pigot, G. Pfister-Guillouzo, H. Lavayssière and S. Mazières, Organometallics, 1999, 18, 5322–5329 CrossRef CAS.
- See, for example:
(a) H. Braunschweig, R. W. Chorley, P. B. Hitchock, M. F. Lappert and L. J.-M. Pierssens, Angew. Chem., Int. Ed., 1994, 33, 1156–1158 CrossRef;
(b) P. B. Hitchock, M. F. Lappert, G. A. Lawless, G. M. de Lima and L. J.-M. Pierssens, J. Organomet. Chem., 2000, 601, 142–146 CrossRef.
- See, for example:
(a) H. Braunschweig, R. W. Chorley, P. B. Hitchock and M. F. Lappert, J. Chem. Soc., Chem. Commun., 1992, 1311–1312 RSC;
(b) H. Braunschweig, B. Gehrhus, P. B. Hitchock and M. F. Lappert, Z. Anorg. Allg. Chem., 1995, 621, 1922–1928 CrossRef CAS;
(c) H. Braunschweig, C. Drost, P. B. Hitchock, M. F. Lappert and L. J.-M. Pierssens, Angew. Chem., Int. Ed., 1997, 36, 261–263 CrossRef CAS;
(d) C. Drost, P. B. Hitchock, M. F. Lappert and L. J.-M. Pierssens, Chem. Commun., 1997, 1141–1142 RSC;
(e) Z.-X. Wang and Y.-X. Li, Organometallics, 2002, 21, 4641–4647 CrossRef CAS;
(f) Z.-X. Wang, D.-Q. Wang and J.-M. Dou, J. Organomet. Chem., 2003, 665, 205–213 CrossRef CAS;
(g) H. Cox, P. B. Hitchock, M. F. Lappert and L. J.-M. Pierssens, Angew. Chem., Int. Ed., 2004, 43, 4500–4504 CrossRef CAS PubMed;
(h) C. F. Caro, M. P. Coles, P. B. Hitchock, M. F. Lappert and L. J.-M. Pierssens, Dalton Trans., 2011, 40, 9821–9983 RSC;
(i) H. Vaňkátová, L. Broeckaert, F. De Proft, R. Olejník, J. Turek, Z. Padělková and A. Růžička, Inorg. Chem., 2011, 50, 9454–9464 CrossRef PubMed;
(j) T. Chlupatý, Z. Padělková, F. De Proft, R. Willem and A. Růžička, Organometallics, 2012, 31, 2203–2211 CrossRef;
(k) W.-C. Chang, A. Raj, H. Hiramatsu, H.-J. Li, M. S. Ziegler, Y. Lin, S. Huanga and H.-J. Liu, Chem. Commun., 2020, 56, 6786–6789 RSC.
- The NMR data reported for compound 7 in ref. 43j has been used as reference.
Footnote |
† Electronic supplementary information (ESI) available: Materials and methods, detailed synthetic procedures, full characterization including 1H and 13C NMR spectra of all compounds. See DOI: https://doi.org/10.1039/d3sc02709k |
|
This journal is © The Royal Society of Chemistry 2023 |