DOI:
10.1039/D3SC01132A
(Edge Article)
Chem. Sci., 2023,
14, 6341-6347
Selective multifunctionalization of N-heterocyclic carbene boranes via the intermediacy of boron-centered radicals†
Received
2nd March 2023
, Accepted 17th May 2023
First published on 18th May 2023
Abstract
The selective difunctionalization of N-heterocyclic carbene (NHC) boranes with alkenes has been achieved via decatungstate and thiol synergistic catalysis. The catalytic system also allows stepwise trifunctionalization, leading to complex NHC boranes with three different functional groups which are challenging to prepare by other methods. The strong hydrogen-abstracting ability of the excited decatungstate enables the generation of boryl radicals from mono- and di-substituted boranes for realizing borane multifunctionalization. This proof-of-principle research provides a new chance for fabricating unsymmetrical boranes and developing boron-atom-economic synthesis.
Introduction
Organoboron chemistry has recently attracted considerable research interest due to the increasing applications of organoboron derivatives in organic synthesis, reticular materials, and pharmaceutical discovery, among others.1 The development of powerful and selective methodologies for the preparation of new organoboron compounds is thus in urgent need.2 A Lewis base borane adduct, L-BH3, has been proved as a readily available and moisture-stable synthon.3 Its three modifiable B–H moieties provide the possibility to be equipped with different functional groups for the design and synthesis of complex organoboron compounds. Vedejs, Curran, and Lacôte pioneered the difunctionalization of N-heterocyclic carbene (NHC) boranes with electron-rich alkenes via the intermediacy of a borenium ion (Scheme 1a).4 The rearrangement of the product was observed to result in a mixture of isomers. Later, Taniguchi and Curran reported stepwise B–H dialkylation and diarylation by the insertion of in situ generated carbenes or benzynes, however with limited examples (Scheme 1b).5 A boryl-radical mediated dicyanation and oxylation of NHC–borane was later developed (Scheme 1c).6 Recently, Pintacuda, Lacôte, and Lalevée discovered a borane–methacrylate photo-click reaction to fabricate polymers containing multifunctional NHC–boranes.7 Despite these seminal advances, to the best of our knowledge, a versatile methodology enabling mono-, di-, and tri-functionalization of NHC–borane remains elusive.
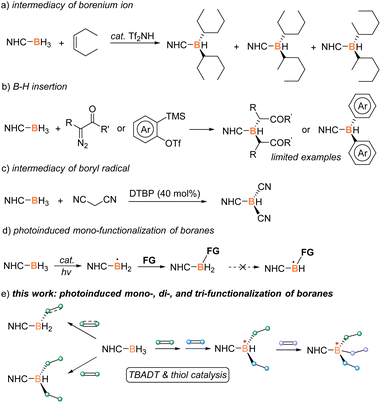 |
| Scheme 1 Representative B–H functionalization of NHC Boranes. | |
Boryl-radical mediated borylation has emerged as transition-metal-free access to various organoboron compounds.8 The active boron-centered radical is reported to attack electron-deficient alkenes,9 alkynes,10 imines,11 (hetero)arenes,12 and isocyanides13 for the construction of new boron–carbon (B–C) bonds. However, the developed strategy has shown limited success in B–H multi-functionalization, probably because the original catalytic system was not competent for the generation of boron-centered radicals from mono-functionalized boranes, the product of the first B–H activation (Scheme 1d). Therefore, a robust and versatile catalytic system is required to efficiently yield boryl radicals from mono- and di-substituted boranes for realizing their multi-functionalization. In view of the strong hydrogen-abstracting ability of excited tetrabutylammonium decatungstate (TBADT),14 we initiated a study of exploring TBADT-based catalysis for borane multi-functionalization via the intermediacy of boryl radicals. After many attempts, controlled mono- and di-functionalization of NHC boranes have been attained (Scheme 1e). The developed strategy also allows tri-functionalization via a stepwise process. The resultant multi-functionalization provides new chances for the construction of chiral boron centers and the potential development of boron atom-economic synthesis.
Results and discussion
Azaarene is arguably one of the most prevalent building blocks in bioactive and drug molecules.15 The traditional transition metal catalysis is typically inefficient for the derivatization of azaarenes due to their strong coordination to metal centers. The active open-shell radicals reportedly react with azaarenes,16 thus providing an alternative route to modifying azaarene derivatives. However, relevant investigation on boryl radicals remains relatively underdeveloped.12 In this connection, we chose 4-vinylpyridine 2 as the model substrate, in view of the unsuccessful application of previous methods on this type of coupling partner (see Table S1† in the ESI for details).8 In the presence of TBADT (1 mol%) and triphenylmethanethiol (50 mol%), the treatment of NHC–borane 1 with 2 in DMA (0.1 M) under light irradiation (390 nm) at room temperature for 48 h afforded the desired disubstituted product 4 in an isolated yield of 61% (Table 1, entry 1). Using DMF or MeCN in place of DMA resulted in a mixture of mono- and di-substituted products (entries 2 and 3, Table 1). Decreasing the thiol loading to 20 mol% led to a similar inferior efficiency (entry 4, Table 1). No reaction occurred in the absence of thiol (entry 5, Table 1) and only a trace amount of 4 was detected in the absence of TBADT (entry 6, Table 1). Control experiments demonstrated that N2 protection and light were also indispensable for this efficient disubstitution reaction (entries 7 and 8, Table 1). The complementary details for the optimization of the reaction conditions are presented in the ESI (Table S1†). Our catalyst system shows superior performance compared with previously reported ones.
Table 1 Optimization of the reaction conditionsa
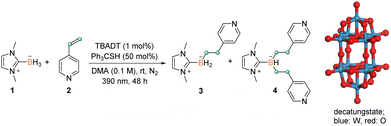
|
Entry |
Variation from standard conditions |
Yield [%] (3/4)b |
Standard conditions A: 1 (0.1 mmol), 2 (0.3 mmol), TBADT (1 mol%), Ph3CSH (50 mmol%), DMA (1 mL), N2, rt, 390 nm, and 48 h; TBADT = tetrabutylammonium decatungstate.
The resultant mixture was first subjected to 11B NMR analysis, and then flash column chromatography was carried out to give the isolated yields of the corresponding products.
|
1 |
None |
0/61 |
2 |
DMF instead of DMA |
27/34 |
3 |
MeCN instead of DMA |
20/28 |
4 |
20 mol% of Ph3CSH |
18/27 |
5 |
Without Ph3CSH |
NR |
6 |
Without TBADT |
0/trace |
7 |
Without N2 |
ND |
8 |
No light irradiation |
NR |
With the optimized di-substitution reaction conditions (standard conditions A) in hand, we explored the substrate generality of this photoinduced di-functionalization with a series of pyridine-bearing alkenes. As shown in Scheme 2, a variety of vinylpyridines containing different substituents reacted with 1 smoothly to generate the corresponding disubstituted products 5–10 in isolated yields ranging from 50% to 62%. The use with 2-vinylpyridine gave a 34% yield of desired product 11, along with the mono-substituted product 41 (8%). Arylvinylpyridines were also compatible with the di-substitution reaction to deliver separable diastereomers of 12 and 13 in 58% and 53% combined yields, respectively. For these substrates, the hydroboration reaction constructed two chiral carbon centers (highlighted in red). When their stereo-configurations were different, a chiral boron center was formed. In principle, three pairs of diastereomers were produced with a ratio of 2
:
1
:
1 (see Scheme S1† in the ESI). 1,2-Di(4-pyridyl)ethylene served as a good coupling partner, giving product 14 in 45% yield. The scope of NHC–boranes was subsequently evaluated. NHC–boranes, more sterically hindered than 1, reacted with 2 well, affording the corresponding products 15–18 with moderate isolated yields.
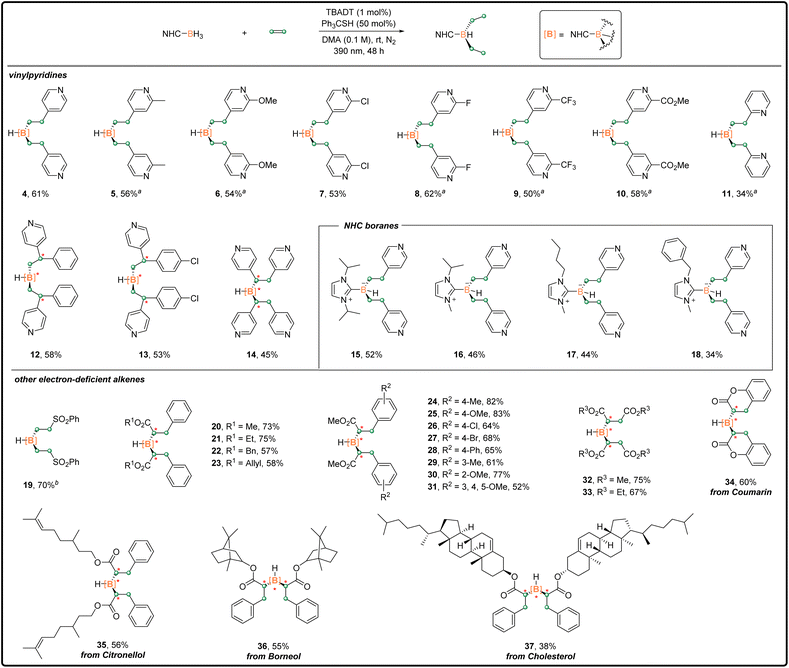 |
| Scheme 2 Disubstitution reaction scope; general procedure A in the ESI.†a4 equiv. of vinylpyridine was used. b2.6 equiv. of phenyl vinyl sulfone was used. | |
Other electron-deficient alkenes were also examined. The utilization of phenyl vinyl sulfone provided 19 in 70% isolated yield. A wide range of cinnamate derivatives decorated with various functional groups on the ester or aryl moiety underwent the target reaction efficiently and consistently. The corresponding products were obtained in good to excellent yields (20–31, up to 83%). No obvious steric or electronic effects of the substituents were observed. It is noteworthy that the tolerated halide, ester, and olefin groups could be readily used for further functionalization. In addition, dimaleates reacted with 1 to give 32 and 33 with yields of 75% and 67%, respectively. Importantly, this methodology could be applied in the synthesis of complex organoboron compounds containing bioactive molecules or natural products, such as coumarin, citronellol, borneol and cholesterol. Moderate to good isolated yields were achieved for 34–37.
We also optimized the reaction conditions for the mono-functionalization of NHC–borane with 4-vinylpyridine (entries 13–15, Table S1† in the ESI). As shown in Scheme 3, the mono-substituted borane 3 was isolated in 52% yield under standard conditions B. Other vinylpyridines worked well to afford the corresponding 38–42 in moderate isolated yields. It is noted that the isolated yields for pyridine-bearing products were obviously lower than their 1H NMR yields (∼10–20% yield drop), due probably to the decomposition of products during purification. A similar yield-drop phenomenon was also observed for the purification of di-substituted boranes with pyridine substituents (Scheme 2). The substrate 2-vinylpyrazine was compatible despite the low isolated yield. Other electron-poor alkenes, including phenyl vinyl sulfone, methyl 4-coumarate, and benzylidenemalonate derivatives, worked as good coupling partners. The corresponding 44–47 were obtained in 53–70% yields. Electron-deficient alkynes were also tested. Dipyridylacetylene reacted with 1 to yield 48 with good E selectivity. In addition, the use of methyl phenylpropiolate resulted in the generation of α-borylated products 49 and 50 in 47% and 26% isolated yields, respectively. The yield is higher than that obtained by a previous method,10e however with relatively moderate E/Z selectivity.
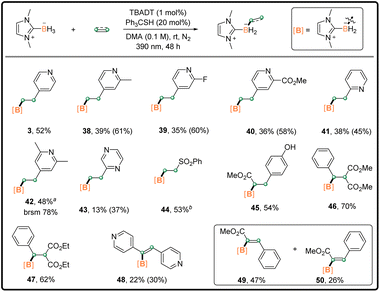 |
| Scheme 3 Monosubstitution reaction scope; general procedure B in the ESI;†1H NMR yield in brackets; general procedure A for 42 and 44–50. a4 equiv. of vinylpyridine was used. b1.2 equiv. of phenyl vinyl sulfone was used. | |
After the investigation on one-pot di-functionalization and mono-substitution, we expanded our exploration by applying the developed strategy in the synthesis of complicated organoboron compounds in a stepwise manner (Scheme 4). The installation of two different alkyl groups succeeded. The unsymmetrical products 51–55 with the stereocenter at the boron atom were attained.17 Notably, a similar stepwise method allowed the synthesis of trisubstituted boranes 56 and 57 although with relatively low isolated yields. Finally, the preparation of unsymmetrical boranes 58 and 59 bearing “quaternary boron” was realized stepwise, which can be viewed as an analogue to quaternary carbon.18 This proof-of-principle research provides new possibility for the construction of chiral boron centers.
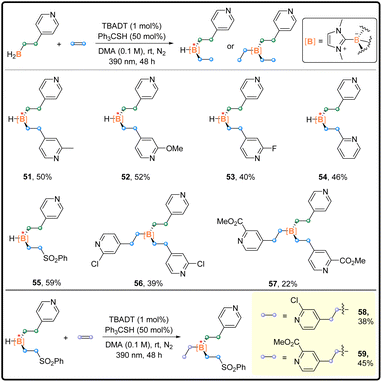 |
| Scheme 4 Diverse substitution reaction scope; general procedure C in the ESI.† | |
The reactivity of representative disubstituted boranes was investigated, and the results are compiled in Scheme 5. Complexes 4 and 6 underwent C–B bond oxidation with hydrogen peroxide to give the corresponding alcohols 60 and 61 in high yields (based on the boron moiety). The treatment of 4 and 19 with Selectfluor led to the fluorination products 62 and 63 in 80% and 74% yields, respectively.19 Furthermore, the Suzuki–Miyaura coupling of 4 with bromobenzene afforded the target product 64 in 136% isolated yield (based on the boron moiety). These results indicated the potential of developing boron-atom economic synthesis, that is the use of one boron atom for the functionalization of multiple carbon centers.
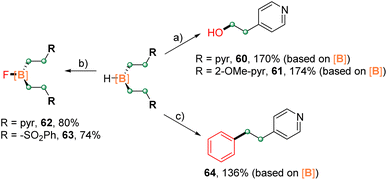 |
| Scheme 5 Transformations. (a) H2O2 (8 equiv.), MeOH/MeCN, rt, and 12 h. (b) Selectfluor (1.1 equiv.), MeCN, rt, and 5 h. (c) PhBr (4 equiv.), PdCl2(dppf) (10 mol%), K3PO4 (6 equiv.), H2O (20 equiv.), THF, 70 °C, N2, and 36 h. | |
To gain more insights into the reaction mechanism, several control experiments were performed (Scheme 6). A radical capture experiment was carried out by adding 2,2,6,6-tetramethyl-1-piperidinyloxy (TEMPO) to the reaction mixture. The disubstitution reaction was completely inhibited, and a boryl radical adduct 65 was detected by HRMS (Scheme 6a). A radical clock experiment was also conducted by using (1-cyclopropylvinyl)benzene as the substrate. Under the standard reaction conditions A, the reaction afforded the ring-opening product 66 in 39% yield (Scheme 6b). These results suggested the involvement of a boryl radical in both B–H functionalization steps. Furthermore, the kinetic isotope effect value kH/kD of borane BH/BD was measured to be 1.0, indicating that the cleavage of B–H bond might not be involved in the rate-determining step (Scheme 6c, Fig. S7†). The replacement of 1 by NHC-BD3 yielded the complex 4-d1 without the deuteration of β-H of the boron atom (Scheme 6c, Fig. S4†). However, the addition of 10 or 50 equiv. of D2O into the reaction mixture afforded the β-H deuterated product 3-d1 with 50% and 80% deuterium incorporation, respectively (Scheme 6c, Fig. S8 and S9†). These results indicated that the β-H might originate from the hydrogen atom transfer (HAT) between the in situ generated β-C radical and thiol. The latter reportedly went through H/D transfer with D2O.20 Furthermore, the same catalytic system enabled the deuteration of methyl groups bonded with nitrogen in DMA (Scheme 6c), indicating the H/D exchange between DMA and thiol, which is believed to account for the little deuteration of β-H when NHC-BD3 was used.
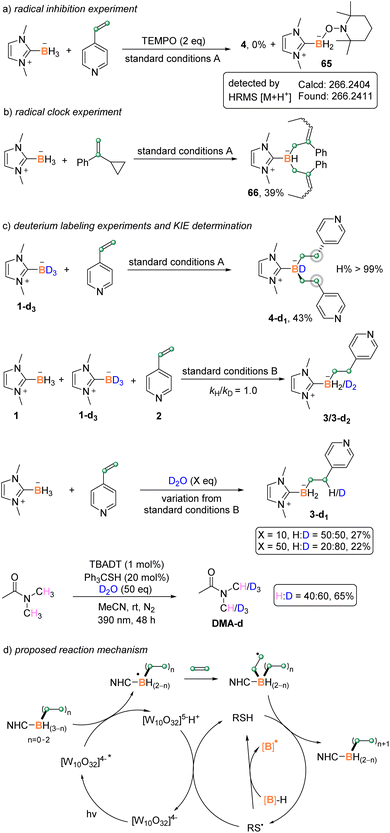 |
| Scheme 6 Mechanistic studies. | |
Based on the above experimental results and literature precedents,9g–i,21 a plausible reaction mechanism is proposed as outlined in Scheme 5d. Upon light irradiation, the excited TBADT abstracts a hydrogen of NHC–borane to give [W10O32]5−H+ and the NHC-boryl radical. The active boryl radical attacks the electron-deficient alkene to generate the carbon radical intermediate, which undergoes HAT with the thiol catalyst to deliver the alkylated product and a thiyl radical. Single electron transfer from [W10O32]5−H+ to the thiyl radical and the subsequent proton transfer regenerate the TBADT and thiol catalysts. Alternatively, the thiyl radical would react with borane directly to give a boron-centered radical to start another cycle. Our light on/off experiments suggested the continuation of the coupling reaction in the dark (Scheme S2† in the ESI), supporting the above HAT reaction between the thiyl radical and borane as well as the involvement of a radical chain pathway. A similar catalytic cycle repeats to give multi-functionalized boranes. The strong hydrogen abstraction ability of excited TBADT is believed to be important for the efficient generation of boryl radicals from substituted boranes.
Conclusions
In summary, a robust and versatile catalytic system featuring the TBADT photosensitizer has been developed for the controlled mono-, di-, and tri-functionalization of NHC–boranes. A wide series of new organoboron compounds are obtained, which are difficult to synthesize by previous methods. The developed stepwise multi-functionalization enables the precise synthesis of unsymmetrical boranes with different substituents, providing new access to constructing chiral boron centers. The transformation of multi-substituted boranes outlines the potential of developing boron-atom economic synthesis. Pursuing the asymmetric catalytic version of multi-functionalization of boranes is underway in our lab.
Data availability
The data underlying this study are available in the ESI.†
Author contributions
Y. Q. conceived and designed the experiments. F. L., X. W., J. L., X. L., J. O., and G. H. performed experiments. F. L. and Y. Q. wrote the manuscript. Y. Q. directed the research.
Conflicts of interest
There are no conflicts to declare.
Acknowledgements
This work was supported by start-up funds from HKUST (Project No. R9804).
Notes and references
- For selected reviews:
(a) H. C. Brown, Tetrahedron, 1961, 12, 117 CrossRef CAS;
(b) N. Miyaura and A. Suzuki, Chem. Rev., 1995, 95, 2457 CrossRef CAS;
(c)
M. G. Davidson, K. Wade, T. B. Marder and A. K. Hughes, Contemporary Boron Chemistry, Royal Society of Chemistry, 2007 Search PubMed;
(d) C. M. Crudden, B. W. Glasspoole and C. J. Lata, Chem. Commun., 2009, 45, 6704 RSC;
(e)
D. G. Hall, Boronic Acids: Preparation and Applications in Organic Synthesis, Medicine and Materials, Wiley-VCH, Weinheim, 2011 CrossRef;
(f) T. S. De Vries, A. Prokofjevs and E. Vedejs, Chem. Rev., 2012, 112, 4246 CrossRef CAS PubMed;
(g) E. C. Neeve, S. J. Geier, I. A. I. Mkhalid, S. A. Westcott and T. B. Marder, Chem. Rev., 2016, 116, 9091 CrossRef CAS PubMed;
(h) J. W. B. Fyfe and A. J. B. Watson, Chem, 2017, 3, 31 CrossRef CAS;
(i) A. García-Domínguez, A. G. Leach and G. C. Lloyd-Jones, Acc. Chem. Res., 2022, 55, 1324 CrossRef PubMed.
- For selected reviews and examples:
(a) L. Monnier, J.-G. Delcros and B. Carboni, Tetrahedron, 2000, 56, 6039 CrossRef CAS;
(b) T. Ishiyama and N. Miyaura, J. Organomet. Chem., 2003, 680, 3 CrossRef CAS;
(c) I. A. I. Mkhalid, J. H. Barnard, T. B. Marder, J. M. Murphy and J. F. Hartwig, Chem. Rev., 2010, 110, 890 CrossRef CAS PubMed;
(d) A. Solovyev, Q. Chu, S. J. Geib, L. Fensterbank, M. Malacria, E. Lacôte and D. P. Curran, J. Am. Chem. Soc., 2010, 132, 15072 CrossRef CAS PubMed;
(e) Q.-Q. Cheng, S.-F. Zhu, Y.-Z. Zhang, X.-L. Xie and Q.-L. Zhou, J. Am. Chem. Soc., 2013, 135, 14094 CrossRef CAS PubMed;
(f) A. Ros, R. Fernández and J. M. Lassaletta, Chem. Soc. Rev., 2014, 43, 3229 RSC;
(g) A. B. Cuenca, R. Shishido, H. Ito and E. Fernández, Chem. Soc. Rev., 2017, 46, 415 RSC;
(h) K. Kubota, H. Iwamoto and H. Ito, Org. Biomol. Chem., 2017, 15, 285 RSC;
(i) L. Xu, G. Wang, S. Zhang, H. Wang, L. Wang, L. Liu, J. Jiao and P. Li, Tetrahedron, 2017, 73, 7123 CrossRef CAS;
(j) A. Issaian, K. N. Tu and S. A. Blum, Acc. Chem. Res., 2017, 50, 2598 CrossRef CAS PubMed;
(k) B. S. L. Collins, C. M. Wilson, E. L. Myers and V. K. Aggarwal, Angew. Chem., Int. Ed., 2017, 56, 11700 CrossRef CAS PubMed;
(l) D. Hemming, R. Fritzemeier, S. A. Westcott, W. L. Santos and P. G. Steel, Chem. Soc. Rev., 2018, 47, 7477 RSC;
(m) M. Kischkewitz, K. Okamoto, C. Mück-Lichtenfeld and A. Studer, Science, 2017, 355, 936 CrossRef CAS PubMed;
(n) S. Namirembe and J. P. Morken, Chem. Soc. Rev., 2019, 48, 3464 RSC;
(o) V. D. Nguyen, V. T. Nguyen, S. Jin, H. T. Dang and O. V. Larionov, Tetrahedron, 2019, 75, 584 CrossRef CAS PubMed;
(p) M. Wang and Z. Shi, Chem. Rev., 2020, 120, 7348 CrossRef CAS PubMed;
(q) S. A. Iqbal, J. Pahl, K. Yuan and M. J. Ingleson, Chem. Soc. Rev., 2020, 49, 4564 RSC;
(r) K. Yang and Q. Song, Acc. Chem. Res., 2021, 54, 2298 CrossRef CAS PubMed;
(s) S. K. Bose, L. Mao, L. Kuehn, U. Radius, J. Nekvinda, W. L. Santos, S. A. Westcott, P. G. Steel and T. B. Marder, Chem. Rev., 2021, 121, 13238 CrossRef CAS PubMed;
(t) W. Ming, H. S. Soor, X. Liu, A. Trofimova, A. K. Yudin and T. B. Marder, Chem. Soc. Rev., 2021, 50, 12151 RSC;
(u) H. Lyu, I. Kevlishvili, X. Yu, P. Liu and G. Dong, Science, 2021, 372, 175 CrossRef CAS PubMed;
(v) A. Lipp, S. O. Badir and G. A. Molander, Angew. Chem., Int. Ed., 2021, 60, 1714 CrossRef CAS PubMed;
(w) S. J. Geier, C. M. Vogels, J. A. Melanson and S. A. Westcott, Chem. Soc. Rev., 2022, 51, 8877 RSC;
(x) S. Rej and N. Chatani, Angew. Chem., Int. Ed., 2022, e202209539 (
Angew. Chem.
, 2022
, 134
, e202209539
) CAS;
(y) T. Taniguchi, Chem.–Eur. J., 2022, 28, e202104333 CAS;
(z) Y. Shoji, N. Tanaka, S. Muranaka, N. Shigeno, H. Sugiyama, K. Takenouchi, F. Hajjaj and T. Fukushima, Nat. Commun., 2016, 7, 12704 CrossRef PubMed.
- For selected reviews:
(a) A. Staubitz, A. P. M. Robertson, M. E. Sloan and I. Manners, Chem. Rev., 2010, 110, 4023 CrossRef CAS PubMed;
(b) D. P. Curran, A. Solovyev, M. M. Brahmi, L. Fensterbank, M. Malacria and E. Lacôte, Angew. Chem., Int. Ed., 2011, 50, 10294 (
Angew. Chem.
, 2011
, 123
, 10476
) CrossRef CAS PubMed;
(c) J.-M. Yang, Z.-Q. Li and S.-F. Zhu, Chin. J. Org. Chem., 2017, 37, 2481 CAS;
(d) D. Lai, S. Ghosh and A. Hajra, Org. Biomol. Chem., 2021, 19, 4397 RSC.
-
(a) A. Prokofjevs, A. Boussonnière, L. Li, H. Bonin, E. Lacôte, D. P. Curran and E. Vedejs, J. Am. Chem. Soc., 2012, 134, 12281 CrossRef CAS PubMed;
(b) A. Boussonnière, X. Pan, S. J. Geib and D. P. Curran, Organomet, 2013, 32, 7445 CrossRef;
(c) Q. Wang, W. Meng, X. Feng and H. Du, Chin. J. Chem., 2021, 39, 918 CrossRef CAS.
-
(a) X. Li and D. P. Curran, J. Am. Chem. Soc., 2013, 135, 12076 CrossRef CAS PubMed;
(b) T. Taniguchi and D. P. Curran, Angew. Chem., Int. Ed., 2014, 53, 13150 CrossRef CAS PubMed;
(c) X. Huang, M. Garcia-Borràs, K. Miao, S. B. J. Kan, A. Zutshi, K. N. Houk and F. H. Arnold, ACS Cent. Sci., 2019, 5(2), 270 CrossRef CAS PubMed.
-
(a) T. Kawamoto, S. J. Geib and D. P. Curran, J. Am. Chem. Soc., 2015, 137, 861 CrossRef PubMed;
(b) D. A. Bolt and D. P. Curran, Adv. Synth. Catal., 2020, 362, 2238 CrossRef.
- B. Aubry, R. Canterel, M. Lansalot, E. Bourgeat-Lami, A. Airoudj, B. Graff, C. Dietlin, F. Morlet-Savary, J. Blahut, L. Benda, G. Pintacuda, E. Lacôte and J. Lalevée, Angew. Chem., Int. Ed., 2021, 60, 17037 CrossRef CAS PubMed.
-
(a) S.-H. Ueng, A. Solovyev, X. Yuan, S. J. Geib, L. Fensterbank, E. Lacôte, M. Malacria, M. Newcomb, J. C. Walton and D. P. Curran, J. Am. Chem. Soc., 2009, 131, 11256 CrossRef CAS PubMed;
(b) T. Taniguchi, Chem. Soc. Rev., 2021, 50, 8995 RSC;
(c) Y.-M. Tian, X.-N. Guo, H. Braunschweig, U. Radius and T. B. Marder, Chem. Rev., 2021, 121, 3561 CrossRef CAS PubMed;
(d) L. Capaldo, T. Noël and D. Ravelli, Chem Catal., 2022, 2, 957 CrossRef CAS;
(e) T.-Y. Peng, F.-L. Zhang and Y.-F. Wang, Acc. Chem. Res., 2023, 56, 169 CrossRef CAS PubMed.
-
(a) S.-C. Ren, F.-L. Zhang, A.-Q. Xu, Y. Yang, M. Zheng, X. Zhou, Y. Fu and Y.-F. Wang, Nat. Commun., 2019, 10, 1934 CrossRef PubMed;
(b) X. Liu, E. E. Lin, G. Chen, J.-L. Li, P. Liu and H. Wang, Org. Lett., 2019, 21, 8454 CrossRef CAS PubMed;
(c) W. Xu, H. Jiang, J. Leng, H.-W. Ong and J. Wu, Angew. Chem., Int. Ed., 2020, 59, 4009 CrossRef CAS PubMed;
(d) J. Qi, F.-L. Zhang, J.-K. Jin, Q. Zhao, B. Li, L.-X. Liu and Y.-F. Wang, Angew. Chem., Int. Ed., 2020, 59, 12876 CrossRef CAS PubMed;
(e) P.-J. Xia, D. Song, Z.-P. Ye, Y.-Z. Hu, J.-A. Xiao, H.-Y. Xiang, X.-Q. Chen and H. Yang, Angew. Chem., Int. Ed., 2020, 59, 6706 CrossRef CAS PubMed;
(f) C. Zhu, J. Dong, X. Liu, L. Gao, Y. Zhao, J. Xie, S. Li and C. Zhu, Angew. Chem., Int. Ed., 2020, 59, 12817 CrossRef CAS PubMed;
(g) G. Li, G. Huang, R. Sun, D. P. Curran and W. Dai, Org. Lett., 2021, 23, 4353 CrossRef CAS PubMed;
(h) X. Liu, Y. Shen, C. Lu, Y. Jian, S. Xia, Z. Gao, Y. Zheng, Y. An and Y. Wang, Chem. Commun., 2022, 58, 8380 RSC;
(i) Y.-Q. Miao, X.-Y. Li, Q.-J. Pan, Y. Ma, J.-X. Kang, Y.-N. Ma, Z. Liu and X. Chen, Green Chem., 2022, 24, 7113 RSC;
(j) Y. Zhang, Y. Liao, P. Liu, Y. Ran and X. Liu, Org. Biomol. Chem., 2022, 20, 3550 RSC;
(k) Q. Shi, M. Xu, R. Chang, D. Ramanathan, B. Peñin, I. Funes-Ardoiz and J. Ye, Nat. Commun., 2022, 13, 4453 CrossRef CAS PubMed.
-
(a) T. Watanabe, D. Hirose, D. P. Curran and T. Taniguchi, Chem.–Eur. J., 2017, 23, 5404 CrossRef CAS PubMed;
(b) T. R. McFadden, C. Fang, S. J. Geib, E. Merling, P. Liu and D. P. Curran, J. Am. Chem. Soc., 2017, 139, 1726 CrossRef CAS PubMed;
(c) S.-C. Ren, F.-L. Zhang, J. Qi, Y.-S. Huang, A.-Q. Xu, H.-Y. Yan and Y.-F. Wang, J. Am. Chem. Soc., 2017, 139, 6050 CrossRef CAS PubMed;
(d) W. Dai, T. R. McFadden, D. P. Curran, H. A. Früchtl and J. C. Walton, J. Am. Chem. Soc., 2018, 140, 15868 CrossRef CAS PubMed;
(e) M. Shimoi, T. Watanabe, K. Maeda, D. P. Curran and T. Taniguchi, Angew. Chem., Int. Ed., 2018, 57, 9485 CrossRef CAS PubMed;
(f) M. Shimoi, K. Maeda, S. J. Geib, D. P. Curran and T. Taniguchi, Angew. Chem., Int. Ed., 2019, 131, 6423 CrossRef;
(g) W. Dai, D. P. Curran and J. C. Walton, J. Org. Chem., 2019, 84, 2102 CrossRef CAS PubMed;
(h) K. Wang, Z. Zhuang, H. Tia, P. Wu, X. Zhao and H. Wang, Chin. Chem. Lett., 2020, 31, 1564 CrossRef CAS;
(i) K. Wang, Q. Yu, W. Mao, Y. Zheng, J. Xu and Y. Wang, iScience, 2022, 25, 104977 CrossRef CAS PubMed;
(j) T. Ye, F.-L. Zhang, H.-M. Xia, X. Zhou, Z.-X. Yu and Y.-F. Wang, Nat. Commun., 2022, 13, 426 CrossRef CAS PubMed.
-
(a) N. Zhou, X.-A. Yuan, Y. Zhao, J. Xie and C. Zhu, Angew. Chem., Int. Ed., 2018, 57, 3990 CrossRef CAS PubMed;
(b) T. Kawamoto, T. Morioka, K. Noguchi, D. P. Curran and A. Kamimura, Org. Lett., 2021, 23, 1825 CrossRef CAS PubMed.
-
(a) W. Dai, S. J. Geib and D. P. Curran, J. Am. Chem. Soc., 2020, 142, 6261 CrossRef PubMed;
(b) P.-J. Xia, Z.-P. Ye, Y.-Z. Hu, J.-A. Xiao, K. Chen, H.-Y. Xiang, X.-Q. Chen and H. Yang, Org. Lett., 2020, 22, 1742 CrossRef CAS PubMed;
(c) J. H. Kim, T. Constantin, M. Simonetti, J. Llaveria, N. S. Sheikh and D. Leonori, Nature, 2021, 595, 677 CrossRef CAS PubMed;
(d) H. Zheng, H. Xiong, C. Su, H. Cao, H. Yao and X. Liu, RSC Adv., 2022, 12, 470 RSC;
(e) H. Sheng, B.-B. Zhang, Q. Liu, Z.-S. Yang, Z.-X. Wang and X.-Y. Chen, Sci. China: Chem., 2022, 65, 2494 CrossRef CAS;
(f) Z. Wang, J. Chen, Z. Lin and Y. Quan, Chem.–Eur. J., 2022, 29, e202203053 Search PubMed;
(g) W. Choi, M. Kim, K. Lee, S. Park and S. Hong, Org. Lett., 2022, 24, 9452 CrossRef CAS PubMed.
- Y. Liu, J.-L. Li, X.-G. Liu, J.-Q. Wu, Z.-S. Huang, Q. Li and H. Wang, Org. Lett., 2021, 23, 1891 CrossRef CAS PubMed.
-
(a) J. G. West, D. Huang and E. J. Sorensen, Nat. Commun., 2015, 6, 10093 CrossRef PubMed;
(b) D. Ravelli, S. Protti and M. Fagnoni, Acc. Chem. Res., 2016, 49, 2232 CrossRef CAS PubMed;
(c) I. B. Perry, T. F. Brewer, P. J. Sarver, D. M. Schultz, D. A. DiRocco and D. W. C. MacMillan, Nature, 2018, 560, 70 CrossRef CAS PubMed;
(d) G. Laudadio, Y. Deng, K. van der Wal, D. Ravelli, M. Nuño, M. Fagnoni, D. Guthrie, Y. Sun and T. Noël, Science, 2020, 369, 92 CrossRef CAS PubMed;
(e) L. Capaldo, M. Fagnoni and D. Ravelli, Chem.–Eur. J., 2017, 23, 6527 CrossRef CAS PubMed.
-
(a)
P. Kiuru and J. Yli-Kauhaluoma, Heterocycles in Natural Product Synthesis, 2011, p. 267 Search PubMed;
(b) O. Thiel, Heterocyclic Chemistry in Drug Discovery (
Angew. Chem., Int. Ed.
, 2013
, 52
, 13515
) Search PubMed;
(c) E. Vitaku, D. T. Smith and J. T. Njardarson, J. Med. Chem., 2014, 57, 10257 CrossRef CAS PubMed.
-
(a) C. K. Prier, D. A. Rankic and D. W. C. MacMillan, Chem. Rev., 2013, 113, 5322 CrossRef CAS PubMed;
(b) K. L. Skubi, T. R. Blum and T. P. Yoon, Chem. Rev., 2016, 116, 10035 CrossRef CAS PubMed.
-
(a) B. Zu, Y. Guo and C. He, J. Am. Chem. Soc., 2021, 143, 16302 CrossRef CAS PubMed;
(b) K. Yang, Y. Mao, J. Xu, H. Wang, Y. He, W. Li and Q. Song, J. Am. Chem. Soc., 2021, 143, 10048 CrossRef CAS PubMed.
-
(a) E. J. Corey and A. Guzman-Perez, Angew. Chem., Int. Ed., 1998, 37, 388 CrossRef;
(b) K. W. Quasdorf and L. E. Overman, Nature, 2014, 516, 181 CrossRef CAS PubMed.
- S. Nerkar and D. P. Curran, Org. Lett., 2015, 17, 3394 CrossRef CAS PubMed.
-
(a) M. Zhang, X.-A. Yuan, C. Zhu and J. Xie, Angew. Chem., Int. Ed., 2019, 58, 312 CrossRef CAS PubMed;
(b) J. Dong, X. Wang, Z. Wang, H. Song, Y. Liu and Q. Wang, Chem. Sci., 2020, 11, 1026 RSC.
- T. Wan, L. Capaldo, D. Ravelli, W. Vitullo, F. J. de Zwart, B. de Bruin and T. Noël, J. Am. Chem. Soc., 2022, 145, 991–999 CrossRef PubMed.
|
This journal is © The Royal Society of Chemistry 2023 |
Click here to see how this site uses Cookies. View our privacy policy here.