DOI:
10.1039/D3SC00911D
(Edge Article)
Chem. Sci., 2023,
14, 5369-5378
Unconventional gas-phase preparation of the prototype polycyclic aromatic hydrocarbon naphthalene (C10H8) via the reaction of benzyl (C7H7) and propargyl (C3H3) radicals coupled with hydrogen-atom assisted isomerization†
Received
17th February 2023
, Accepted 19th April 2023
First published on 19th April 2023
Introduction
Since the pioneering observation of the simplest organic radical methylidyne (CH) toward ζ-Oph by Swings and Rosenfeld in 1973,1 resonantly stabilized free radicals–organic radicals in which the unpaired electron is delocalized over multiple carbon atoms – such as propargyl
have been suggested as fundamental building blocks in molecular mass growth processes to polycyclic aromatic hydrocarbons (PAHs).2–12 Polycyclic aromatic hydrocarbons (PAHs) are organic molecules consisting of fused benzene rings with naphthalene (C10H8) being the simplest representative.13 Along with their protonated, ionized, (de)hydrogenated, alkylated, and nitrogen-substituted counterparts like (iso)quinoline (C9H7N), aromatics in deep space have been projected to account for up to 30% of the galactic carbon budget.14–20 PAHs identified in carbonaceous chondrites like Allende, Murchison, and Orgueil as well as in cold molecular clouds such as the Taurus Molecular Cloud (TMC-1) through their cyano derivatives such as 1- and 2-cyanonaphthalene (C10H7CN)21 may signify the missing link between resonantly stabilized free radicals and carbonaceous nanoparticles, commonly referred to as interstellar grains.16–20,22
Whereas on present-day Earth, PAHs along with carbonaceous nanoparticles (soot) as their descendants exemplify unwanted, often carcinogenic by-products of incomplete combustion processes,23–25 carbon-rich circumstellar environments of Asymptotic Giant Branch (AGB) stars and of planetary nebulae have been inferred as natural ‘breeding grounds’ of PAHs on the macroscopic scale.26–28 However, as of now, there is still a critical lack of a fundamental understanding of the reaction pathways of polycyclic aromatics in carbon-rich interstellar envelopes. This deficiency is evident considering the predicted lifetimes of interstellar PAHs of a few 108 years limited through their destruction by shock waves and galactic cosmic rays,16,29–32 while the time scale for the injection of new PAHs from circumstellar envelopes to the interstellar medium exceeds some 109 years.32 This controversy suggests PAHs should not exist in extraterrestrial environments and in meteorites, thus implying that our knowledge on the underlying formation mechanisms to even the simplest representatives of PAHs – the 10π Hückel aromatic naphthalene (C10H8) molecule – in carbon rich circumstellar envelopes is still in its infancy.
Hansen and coworkers investigated molecular-growth pathways in propyne-doped low-pressure premixed flames of benzene and toluene and suggested that benzyl
radicals contribute to naphthalene formation through reactions with propargyl
radical along with the involvement of phenyl-substituted butadienyl and vinylacetylene isomers.33 The kinetics and mechanisms of the recombination reaction between benzyl
and propargyl
radicals have been theoretically studied by utilizing the B3LYP, CBS-QB3, and CASPT2 quantum chemical methods, as well as the steady-state unimolecular master equation analysis based on the Rice–Ramsperger–Kassel–Marcus theory.34 Here, we report on the results of molecular beams experiments combined with electronic structure calculations along with computational fluid dynamics (CFD) and kinetic modeling of the reaction between the resonantly stabilized benzyl
and the propargyl
radicals. The experiments exploit a chemical microreactor coupled with isomer-specific detection of the naphthalene molecule (C10H8) as the prototype PAH carrying two fused benzene rings through tunable vacuum ultraviolet (VUV) light. The barrierless additions of the aromatic and resonantly stabilized benzyl radical
to the methylenic (CH2) or acetylenic (CH) moieties of the resonantly stabilized propargyl
radical access the 3-butynylbenzene or 2,3-butadienylbenzene (C10H10) collision complexes, which undergo facile isomerization (hydrogen migration, ring closure) followed by atomic hydrogen loss to distinct methylene-indanyl radicals
At elevated temperatures, these radicals undergo yet another hydrogen atom loss accessing benzofulvene (C10H8) and naphthalene (C10H8). This facile Propargyl Addition–BenzAnnulation (PABA) mechanism involving the reaction of astronomically abundant propargyl radicals35 with aromatic radicals carrying the radical center at the off-ring methylene moiety
with benzyl
to naphthalene and perhaps higher order PAHs like anthracene and phenanthrene signifies a fundamental shift in the perception that PAHs are predominantly formed via the Hydrogen-Abstraction–Acetylene Addition (HACA) pathway in carbon rich circumstellar envelopes.13
Results
Mass spectra
The reaction between the resonantly stabilized benzyl
and propargyl
radicals was explored by exploiting a chemical micro reactor36 with radicals prepared in situ through flash pyrolysis of the benzylbromide (C6H5CH2Br)37 and propargyl bromide (C3H3Br)38 precursors, respectively, within a heated silicon carbide tube at 1473 K. The products were entrained in a molecular beam, ionized via fragment-free, soft photoionization39 using tunable synchrotron vacuum ultraviolet (VUV) light, and detected isomer-specifically with a reflectron time-of-flight mass spectrometer (Re-TOF-MS) by scanning the photon energy from 7.90 to 10.05 eV (ESI†). A representative mass spectrum recorded at a photon energy of 9.50 eV for the reaction of the benzyl with propargyl radicals at a reactor temperature of 1473 K is shown in Fig. 1. Control experiments of helium-seeded benzylbromide and propargyl bromide precursors within the identical experimental setup were also studied by keeping the silicon carbide tube at 298 K (Fig. 1a). We would like to highlight that the self-recombination of propargyl38 or of benzyl radicals37 does not lead to signal from m/z = 128 to 130. Hence, a comparison of the mass spectra provides compelling evidence that signal at m/z = 128 (C10H8+), 129 (13CC9H8+ and C10H9+), and 130 (C10H10+) is linked to the reaction of benzyl with propargyl radicals (Fig. 1b); signal at these m/z ratios is absent in the control experiment (Fig. 1a). Note that the intensity of the ion counts at m/z = 128 (C10H8+) increases, whereas those at m/z = 129 and 130 diminish as the temperature of the reactor increases from 1173 K to 1473 K (Fig. 2). Due to the natural abundance of 13C, the 13CC9H8+ should only contribute up to 11% to the total intensity of m/z = 129, which suggests that there should be a significant amount of C10H9+. Accounting for the molecular weight of the reactants (
91 amu;
39 amu) and products (C10H8, 128 amu; C10H10, 130 amu), species with the molecular formula C10H10 can be linked to reaction products of the benzyl–propargyl radical–radical recombination. The temperature-dependance study (Fig. 2b) reveals that the higher temperature favors hydrogen loss and thus gradually consumes the signal of C10H10+ to C10H9+ and C10H8+. At our highest experimental temperature of 1473 K, the ion counts of m/z = 129 stabilize at a level of (13 ± 4)% to m/z = 128, whereas m/z = 130 is almost depleted, indicating that there remains mostly 13CC9H8+ in m/z = 129. To summarize, the analysis of the mass spectra alone reveals that the reaction of the benzyl
with the propargyl
radical synthesizes hydrocarbon molecules with the molecular formulae C10H10 and C10H8 in the gas-phase.
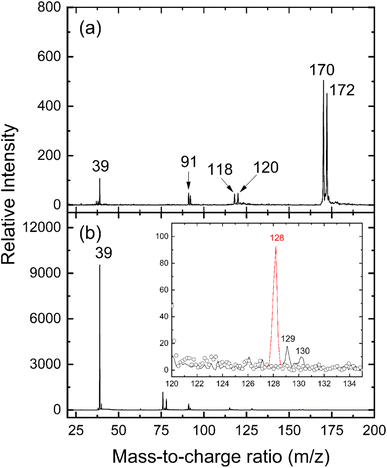 |
| Fig. 1 Photoionization mass spectra recorded at a photon energy of 9.50 eV for the benzyl ( m/z = 91) plus propargyl ( m/z = 39) reaction at a temperature of 298 K (a) and 1473 ± 10 K (b). At 298 K, m/z = 118 and 120 are C3H379Br and C3H381Br, and m/z = 170 and 172 are C6H5CH279Br and C6H5CH281Br, respectively, whereas the small portion of propargyl and benzyl radicals are generated via dissociative photoionization. The inset in (b) highlights ion signal from m/z = 120 to 135 including naphthalene and potential isomers (C10H8; m/z = 128) at 298 K (open circles) and 1473 ± 10 K (solid line), respectively. | |
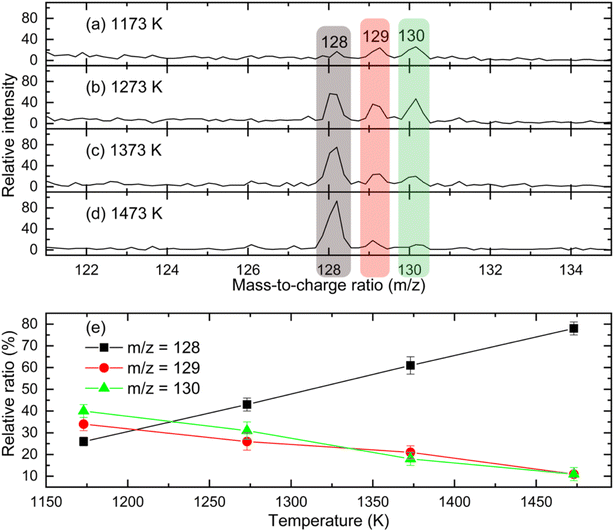 |
| Fig. 2 Photoionization mass spectra from m/z = 121 to 135 recorded at a temperature of 1173 K (a), 1273 (b), 1373 (c), and 1473 K (d) at a photon energy of 9.50 eV for the benzyl plus propargyl reaction along with the relative ratio of m/z = 128, 129, and 130 as a function of the micro reactor temperature (e). | |
Photoionization efficiency curves
With the detection of hydrocarbon molecules of the molecular formula C10H10 and C10H8, it is our objective to elucidate the nature of the structural isomer(s) formed in the reaction of the benzyl with the propargyl radicals. A detailed analysis of the corresponding photoionization efficiency (PIE) curves for ions from m/z = 128 (C10H8+) to 130 (C10H10+) allows an elucidation of the structural isomers formed in the reaction. This PIE curve reports the intensity of a well-defined m/z ratio as a function of the photon energy from 7.90 to 10.0 eV (Fig. 3). The experimentally derived PIE curve at m/z = 128 (red) can be reproduced effectively by the reference PIE curve of naphthalene (C10H8, blue) at the highest temperature of 1473 K.40 The PIE curves of m/z = 129 (cyan) and m/z = 128 (red) are superimposable after scaling suggesting that ions at m/z = 129 at 1473 K are associated with 13C-naphthalene (13CC9H8+), being congruent with our discussion about the composition of m/z = 129 above. Hence, we conclude that within our error limits, naphthalene (C10H8) denotes the only contribution to signal at m/z = 128. An elucidation of the structural isomers at m/z = 130 (Fig. S1†) is tricky since no experimental PIE curves exist for any C10H10 isomer. Therefore, these are provided via electronic structure calculations (ESI; Fig. S2 and S3†). However, the evaluated adiabatic IE of 1,2-dihydronaphthalene has been reported as 8.0 eV,41 which does not match the onset of the experimental PIE curve at m/z = 130 at 8.95 ± 0.05 eV. Hence, 1,2-dihydronaphthalene is not formed in the reaction. Altogether, the analysis of the PIE curves at m/z = 128 and 130 reveals the formation of naphthalene and possibly to a minor extent benzofulvene (C10H8; 128 amu) as well as 3-butynylbenzene (HCCCH2CH2C6H5; C10H10; 130 amu).
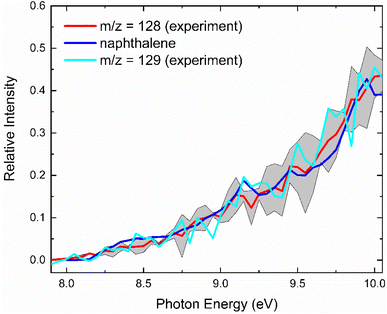 |
| Fig. 3 Experimental PIE curves of mass-to-charge ratios of m/z = 128 (red) and m/z = 129 (cyan) recorded in the reaction of the benzyl radical with propargyl radicals at a reactor temperature of 1473 ± 10 K. The reference PIE curve of naphthalene is shown in blue. The error bars consist of two parts: ±10% based on the accuracy of the photodiode and a 1σ error of the PIE curve averaged over the individual scans. | |
Reaction mechanisms – formation of methyleneindanyl radicals
The C10H10 potential energy surface (PES) accessed by the benzyl–propargyl radical reaction has been described in detail in previous publications;34,42 here we focus on the channels which are relevant to the experimental conditions of the present study and to conditions in high temperature circumstellar envelopes (Fig. 4a, b, 5, S2–S5 and Table S1†). The resonance stabilized propargyl radical
can recombine barrierlessly with its radical center located at the methylenic (CH2) or acetylenic (CH) moiety with the benzyl radical
(Fig. 4a) forming intermediates i1 (3-butynylbenzene, C10H10) and i2 (2,3-butadienylbenzene, C10H10), respectively; these are stabilized by 283 and 287 kJ mol−1 with respect to the separated reactants. The subsequent reaction mechanism features a closure of the five-membered ring involving the newly added C3H3 moiety producing various methylene-indane isomers (i3–i5; i7–i9), followed by hydrogen atom migrations conserving the same methylene-indane skeleton, and completed by an atomic hydrogen loss leading to isomers of the methylene-indanyl radical (p3–p5). In particular, i2 undergoes ring closure to 1-methylene-indane i3; the latter can eliminate atomic hydrogen forming the 1-methylene-2-indanyl p4 product via a low exit barrier of only 6 kJ mol−1 above the products with the overall reaction exoergicity of 70 kJ mol−1. Alternatively, hydrogen atom shifts in i3 can lead to i4 or i5via barriers which are higher than that for the hydrogen atom loss. In turn, i4 can decompose without exit barriers to p4 and also to 3-methylene-1-indanyl p5. The i5 intermediate can dissociate via hydrogen atom loss to p5 without an exit barrier and may also eliminate a molecular hydrogen producing benzofulvene (1-methylene-1H-indene) p1via a tight exit transition state. Although these products are thermodynamically favored compared to the hydrogen atom loss products p4/p5, the molecular hydrogen loss is not competitive with the atomic hydrogen loss pathways according to the kinetics calculations (Fig. S4†). The five-membered ring closure in the initial complex i1 has to be preceded by a shift of the C3H3 moiety to the ortho carbon atom in the ring, i1 → i6; in principle, i6 can be also accessed by the addition of propargyl by its CH end to the ortho carbon of benzyl. Next, the five-membered ring closure in i6 results in 2-methylene-indane i7. A facile 1,2-H migration in i7 from the edge connecting the six- and five-membered rings to the neighboring CH group in the five-membered ring requires a barrier of only 25 kJ mol−1 and produces a low-lying C2v symmetric isomer i8. The latter can eliminate one of four symmetric hydrogen atoms from CH2 groups in the five-membered ring forming 2-methylene-1-indanyl p3 without an exit barrier; alternatively, a molecular hydrogen loss leading to 2-benzofulvene (2-methylene-2H-indene) p2via a tight transition state and high transition state was computed; again, this channel is not competitive with the hydrogen atom loss. Two other hydrogen atom shifts in i7 can lead to i9 and i10, but they feature higher barriers as compared to the one for i7 → i8. The i9 and i10 isomers are also connected via a hydrogen atom migration; both of them can split a hydrogen atom forming p3 without exit barriers. In summary, the most favorable exit channels of the benzyl–propargyl reaction include i2 → p4 + H and i1 → i6 → i7 → i8 → p3 + H viai2 and i1, respectively. These lead to the formation of methylene-indanyl radicals, which are precursors of benzofulvene and naphthalene as discussed in the following paragraph.
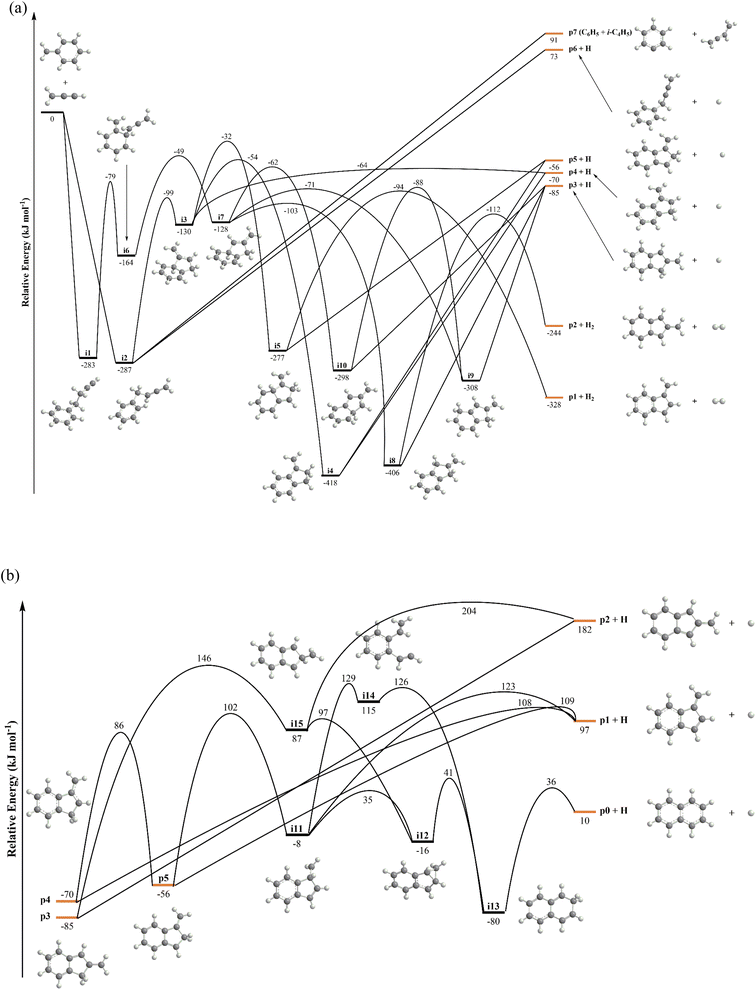 |
| Fig. 4 (a) Main reaction pathways for the benzyl plus propargyl reaction. All energies are given in kJ mol−1 with respect to the energy of the separated reactants. (b) Main reaction pathways for unimolecular decomposition methylene-indanyl radicals C10H9 (p3–p5). All energies are given in kJ mol−1 with respect to the energy of the initial reactants of the benzyl–propargyl radical–radical reaction. Atoms are colored in black (carbon) and gray (hydrogen). | |
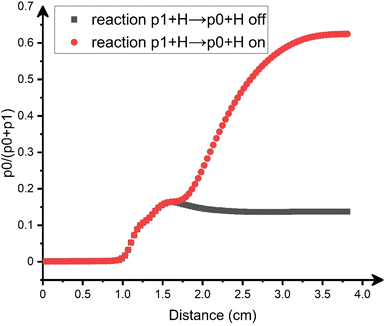 |
| Fig. 5 Relative yield of naphthalene (p0) with respect to the total yield of C10H8 calculated with computational fluid dynamics (CFD) and kinetic simulations with and without hydrogen atom assisted isomerization of benzofulvene (p1) to naphthalene (p0). The x-axis specifies the distance along the length of the micro reactor. | |
The total and individual product channel reaction rate constants computed at the pressure of 30 torr representative of the hot reactive zone in the micro reactor are illustrated in Fig. S4a.†36,43 At lower temperatures, the radical recombination is followed by collisional stabilization of i1 and i2, which is preferable up to 1500 K. According to the computed rate constants for the unimolecular decomposition of these two C10H10 isomers, their lifetime in the 1375–1500 K range is on the order of 2–19 μs, and the prevailing decomposition channels lead back to the reactants. Since the computed adiabatic ionization energy (IE) of 3-butynylbenzene i1, of 8.8 ± 0.1 eV, reveals a good match with the experimental onset of the PIE curve of m/z = 130 of close to 8.9 eV (Fig. S1†), a sufficient fraction of i1 survives long enough to exit the micro reactor. On the other hand, 2,3-butadienylbenzene i2 has a much lower computed IE of 8.4 ± 0.1 eV. The computational method employed here for the determination of the IEs typically underestimates the experiment by about 0.1 eV, e.g. the computed values for phenylacetylene and naphthalene are 8.68 and 8.01 eV versus the NIST evaluated values of 8.82 and 8.14 eV, respectively;44 therefore, it is unlikely that i2 contributes to the experimental PIE curve for m/z = 130 (Fig. S1†). The computationally predicted relative yield of i2 is significantly lower than that of i1 under the reactor conditions and hence, the amount of i2 molecules that survive to exit the reactor was insufficient for their detection. At higher temperatures, the endoergic products 1-phenyl-2-butyn-4-yl (C6H5CH2CCCH2) plus atomic hydrogen (p6) and phenyl (C6H5) plus 1,3-butadien-2-yl (i-C4H5) (p7) take over and become dominant; but their formation does not open any pathways toward naphthalene. On the other hand, the yield of 1-methylene-2-indanyl p4 maximizes in the 1250–1750 K temperature range, where the rate constant for its production reaches 6–7 × 10−14 cm3 molecule−1 s−1 (Fig. S4†). In the same temperature interval, the rate constants to form alternative methylene-indanyl radicals p3 and p5 also reach their maximal values of about 9 × 10−15 and 3 × 10−15 cm3 molecule−1 s−1, respectively, but they are appreciably lower than that for the formation of p4. It should be also noted that the rate constants to form benzofulvene isomers p1 and p2 along with molecular hydrogen are more than two orders of magnitude lower than that for the atomic hydrogen loss exit channel forming p4. While the pathway toward naphthalene plus molecular hydrogen also exists in the primary reaction, the calculated rate constant is on the order of 10−17 cm3 molecule−1 s−1 in the relevant temperature range. Below we seek to answer, how then can the experimentally detected naphthalene isomer be formed in the microreactor?
Reaction mechanism – reaction of methyleneindanyl radicals to benzofulvene and naphthalene
Previous calculations of the C10H9 PES firmly established the methylene-indanyl radicals as critical precursors of benzofulvenes (p1, p2) and naphthalene (p0) (Fig. 4b) at elevated temperatures.45 Here, we briefly highlight the results by discussing the most significant reaction pathways as compiled in Fig. 4b. 1-Methylene-2-indanyl p4 can decompose via atomic hydrogen loss to benzofulvene p1via a barrier of 178 kJ mol−1 or undergoes a 1,2-H shift forming 3-methylene-1-indanyl p5via a 156 kJ mol−1 barrier. The latter can also dissociate to p1via atomic hydrogen loss or isomerize to naphthalene (p0) via a multistep isomerization sequence involving one additional hydrogen atom migration to the ipso position, i6 → i11, insertion of the methylene group into the C–C bond in the five-membered ring leading to the ring expansion, i11 → i12 → i13, and hydrogen atom loss from i13 producing naphthalene p0. The highest barrier on the naphthalene formation pathway is 172 kJ mol−1 relative to p4, which is slightly lower than that for the formation of benzofulvene. However, p1 is produced both from p4 and p5via relatively loose hydrogen atom loss transition states; hence, this channel is preferable from the point of view of entropy. The preference for the formation of p1 over p0 in unimolecular decomposition of p4 is displayed in the rate constants for these product channels illustrated in Fig. S4c.† The RRKM–ME rate constant calculations take into account both barrier heights and entropies of activation and the multistep character of the p4 → p0 + H pathway as compared to the direct p4 → p1 + H dissociation. In particular, at 1000 and 1250 K, the computed p4 → p1 + H rate constant is respectively, factors of 5.9 and 4.6 higher than that for p4 → p0 + H. 2-Methylene-1-indanyl p3 can dissociate only to the high-lying 2-benzofulvene p2 plus atomic hydrogen without an exit barrier, but with a high energy demand of 267 kJ mol−1. The alternative pathway to p0 plus atomic hydrogen involves a hydrogen atom shift to the ipso position, p3 → i15, followed by a facile CH2 insertion into the five-membered ring, i15 → i12 → i13, and subsequent atomic hydrogen elimination.
The calculated rate constants for decomposition of the methylene-indanyl radicals (p3–p5) to benzofulvene and naphthalene are illustrated in Fig. S4c.† The rate constants are high at temperatures above 1000 K. For instance, for p4 – the main product of the primary benzyl–propargyl radical reaction – the values at 1250 K reach 1.7 × 105 and 7.7 × 105 s−1 for the p0 plus atomic hydrogen and p1 plus atomic hydrogen channels, respectively, corresponding to the lifetime of about 1 μs. Moreover, at higher temperatures, the calculations predict p4 not to exist as a chemical species but to merge/equilibrate with p0/p1 plus atomic hydrogen on the time scale which is faster than its collisional relaxation. Thus, at our higher experimental temperatures, p4 is expected to rapidly dissociate to p1 plus atomic hydrogen which is corroborated by the significant reduction in the m/z = 129 signal with the temperature increase (Fig. 2) accompanied with a growth of the m/z = 128 signal. The p5 species decomposes faster and is less thermally stable than p4, whereas p3 is more stable. The latter can survive up to 1650 K but the calculated p3 → p0 plus atomic hydrogen rate constant at 1500 K for its prevailing dissociation channel to naphthalene (p0), 3.5 × 105 s−1, corresponds to the lifetime of only about 3 μs.
Reaction mechanism – hydrogen assisted isomerization of benzofulvene to naphthalene
In addition to the secondary decomposition of methyleneindanyl (p3–p5; C10H9), benzofulvene (p1) can be converted to naphthalene (p0) via a hydrogen atom assisted isomerization. The isomerization rate constant is known to be high, 4 × 10−11 cm3 molecule−1 s−1, in the relevant temperature range (Fig. S4d†).45 While naphthalene (p0) has been clearly observed experimentally, the unequivocal identification of benzofulvene is impossible due to the unavailability of its measured PIE; this species is unstable under normal conditions. The fact that the calculated IE of benzofulvene (p1), 7.97 eV, is very close to that of naphthalene (p0), 8.01 eV, prohibits any distinction between the two isomers solely based on the onset of the experimental PIE curve. 2-Benzofulvene (p2) can be clearly ruled out since its calculated IE is much lower, 7.14 eV, and no ion signal is observed at this range for m/z = 128. To evaluate the naphthalene (p0)/benzofulvene (p1) branching ratio under the experimental conditions and to assess the role of the hydrogen assisted isomerization between them, we carried out computational fluid dynamics (CFD) and kinetic modeling of the processes in our microreactor (ESI†). According to the results (Fig. 5), at the exit of the reactor, the relative yield of naphthalene constitutes 62% of the total C10H8 yield. The contribution of the hydrogen-assisted isomerization is significant. When the hydrogen assisted isomerization (p1 + H → p0 + H) is intentionally excluded from the kinetic model, the final yield of naphthalene drops to only 14% of the total. Thus, the CFD and kinetic modeling confirms that naphthalene (p0) is the prevalent C10H8 isomer observed experimentally, but it forms mostly via benzofulvene (p1) and the contribution of the latter to the observed PIE curve at m/z = 128 cannot be discounted. Overall, we can conclude that the fast secondary decomposition of methylene-indanyl radicals (C10H9) is predicted to be responsible for the formation of benzofulvene (p1) and naphthalene (p0) via the following (simplified) reaction mechanism including the hydrogen assisted isomerization of benzofulvene (p1): | C7H7 + C3H3 → C10H9 (p3 + p4 + p5) + H | (1) |
| C10H9 (p3 + p4 + p5) → C10H8 (p0) + H | (2) |
| C10H9 (p3 + p4 + p5) → C10H8 (p1) + H | (3) |
| C10H8 (p1) + H → C10H8 (p0) + H | (4) |
Reaction mechanism – circumstellar envelopes and cold molecular clouds
Let us now consider the reaction kinetics under conditions of circumstellar envelopes, i.e., at high temperatures and low pressures. Fig. S4b† illustrates the reaction rate constants for the benzyl–propargyl process computed in the limit of low pressure, but considering infrared radiative stabilization of the C10H10 intermediates (ESI†). One can see that in this case, stabilization of i2 is no longer significant, whereas stabilization of i1 prevails only up to 600 K. Above 600 K and up to 1400 K, p4 is the main product with its rate constant being in the range of 2 × 10−12 to 1 × 10−13 cm3 molecule−1 s−1, more than an order of magnitude higher than its values at 30 torr. Clearly, the reduction of pressure strongly favors the production of 1-methylene-2-indanyl p4, which is a critical precursor of benzofulvene (p1)/naphthalene (p0) at high temperatures. Even though the yield of the endoergic p6 and p7 products exceeds that of p4 above 1400 K, the benzyl plus propargyl rate constant to p4 plus atomic hydrogen does not fall below 10−14 cm3 molecule−1 s−1 up to 2000 K. Thus, the reaction mechanism is applicable to the conditions of circumstellar envelopes of carbon rich stars with lower pressures stimulating the higher yield of the C10H8 isomers. In contrast, the benzyl–propargyl reaction is predicted not to be a viable source of naphthalene in low-temperature conditions such as in cold molecular clouds like the Taurus Molecular Cloud 1 (TMC-1). The rate constants computed in the 70–200 K range are illustrated in Fig. S5 (ESI).† While the total rate constant rises above 10−10 cm3 molecule−1 s−1 at low temperatures, the reaction nearly exclusively forms C10H10 (i1) via radiative stabilization. A small fraction of p4 is produced with the rate constant on the order of 10−14 cm3 molecule−1 s−1, however, at such low temperatures, the 1-methylene-2-indanyl radical (p4) does not possess sufficient energy to decompose via atomic hydrogen loss. The rate constants for the production of benzofulvene (p1) and naphthalene (p0) along with molecular hydrogen in the primary reaction are evaluated to be as low as 10−17 and ∼10−19 cm3 molecule−1 s−1, respectively, and hence can be neglected. Therefore, despite being classified as a radical–radical reaction, bimolecular reactions between the propargyl and the benzyl radicals are closed in cold molecular clouds, and radiative stabilization of the initial collision complex may control the outcome of the reaction, whereas in circumstellar envelopes, naphthalene (p0) along with minor fractions of benzofulvene (p1) can be formed via a complex sequence of chemical reactions (1) to (4).
Conclusion & outlook
The radical–radical reaction of propargyl with benzyl represents a fundamental benchmark of a benzannulation of an aromatic ring and could imply a versatile multistep mechanism of PAH growth in high temperature circumstellar envelopes of carbon rich stars and planetary nebulae as their descendants. Each PAH carrying a benzyl moiety in conjunction with a non-substituted carbon atom at the ortho position of the methylene group
may undergo ring annulation upon reaction with the propargyl radical (Fig. 6). In strong analogy of the benzyl–propargyl system leading via benzannulation through hydrogen assisted isomerization to the simplest PAH carrying two six-membered rings, i.e. naphthalene (C10H8), reactions of 1′- and 2′-methylnaphthyl
with propargyl may eventually access anthracene and phenanthrene (C14H10), which carry three six-membered rings. In deep space, 1′- and 2′-methylnaphthyl
can be generated easily via photodissociation of 1- and 2-methylnaphthalene (C10H7CH3), respectively, which in turn can be formed via the barrierless reactions of tolyl radicals (C6H4·CH3) with vinylacetylene (C4H4) as demonstrated recently in crossed molecular beams experiments.46,47 Further molecular mass growth processes commence with the photolysis and carbon–hydrogen bond cleavage within the methyl group of distinct isomers of methylated phenanthrenes and anthracenes; upon reaction with the propargyl radicals and eventual molecular hydrogen loss, five distinct C18H12 isomers including the simplest representatives of helicenes ([4]helicene), acenes (tetracene), and phenacenes ([4]phenacene) can be synthesized in deep space via entrance-barrierless, exoergic bimolecular reactions involving the propargyl radical (Fig. 5). Therefore, this novel reaction mechanism, which we designate Propargyl Addition–BenzAnnulation (PABA), may represent a critical sink of interstellar propargyl radicals as detected at substantial fractional abundance relative to molecular hydrogen of 8.7 × 10−9 toward TMC-1;35 this complex reaction sequence may represent a unusual molecular mass growth process potentially rivaling the Hydrogen Abstraction–Acetylene Addition (HACA)48–50 and Hydrogen Abstraction–Vinylacetylene Addition (HAVA) mechanisms.40,51–54 Therefore, the conceptual framework of the Propargyl Addition–BenzAnnulation (PABA) mechanism involving the reaction of astronomically abundant propargyl radicals with aromatic radicals carrying the radical center at the off-ring methylene moiety
provides a promising source of polyaromatics in carbon-rich, high temperature circumstellar environments thus bringing us closer to the unraveling of the aromatic nature of the universe we live in.
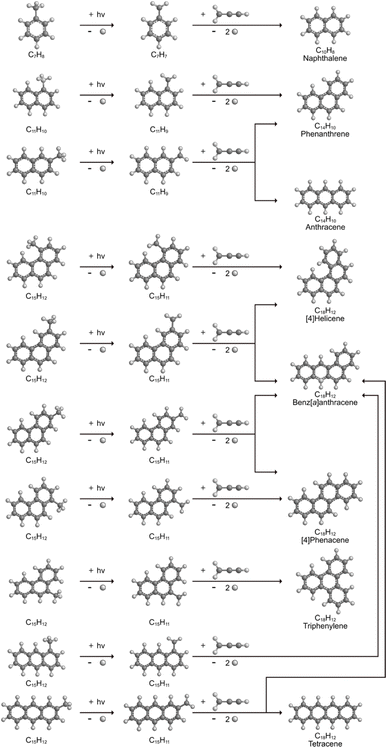 |
| Fig. 6 Selected PAHs carrying a benzyl moiety in conjunction with a non-substituted carbon atom at the ortho position of the methylene group can undergo overall ring annulation upon multi step reactions with the propargyl radical. | |
Data availability
Essential data are provided in the main text and the ESI.† Additional data can be available from the corresponding author upon reasonable request.
Author contributions
R. I. K. designed the experiment; C. H., and W. L. carried out the experimental measurements; M. A. supervised the experiment; C. H. performed the data analysis; V. S. K., P. S. P., M. V. Z., V. N. A., A. N. M., and A. M. M. carried out the theoretical analysis; R. I. K., A. M. M., and M. A. discussed the data; C. H., R. I. K., A. M. M., and M. A. wrote the paper.
Conflicts of interest
The authors declare no conflict of interest.
Acknowledgements
This work was supported by the US Department of Energy, Basic Energy Sciences DE-FG02-03ER15411 (experimental studies) and DE-FG02-04ER15570 (computational studies) to the University of Hawaii (UH) and Florida International University (FIU), respectively. W. L. and M. A. were supported by the Director, Office of Science, Office of Basic Energy Sciences, of the U.S. Department of Energy under Contract No. DE-AC02-05CH11231, through the Gas Phase Chemical Physics program of the Chemical Sciences Division. The Advanced Light Source at Berkeley is also supported under Contract No. DE-AC02-05CH11231. Ab initio calculations at Samara were supported by the Ministry of Higher Education and Science of the Russian Federation under Grant No. 075-15-2021-597.
References
- P. Swings and L. Rosenfeld, Considerations Regarding Interstellar Molecules, Astrophys. J., 1937, 86, 483–486 CrossRef CAS.
- A. Leger and J. L. Puget, Identification of the “Unidentified” IR Emission Features of Interstellar Dust?, Astron. Astrophys., 1984, 137, L5–L8 CAS.
- G. Nagendrappa, Benzene and Its Isomers, Resonance, 2001, 6, 74–78 CrossRef CAS.
- T. C. Dinadayalane, U. D. Priyakumar and G. N. Sastry, Exploration of C6H6 Potential Energy Surface: A Computational Effort to Unravel the Relative Stabilities and Synthetic Feasibility of New Benzene Isomers, J. Phys. Chem. A, 2004, 108, 11433–11448 CrossRef CAS.
- J. A. Miller and C. F. Melius, Kinetic and Thermodynamic Issues in the Formation of Aromatic Compounds in Flames of Aliphatic Fuels, Combust. Flame, 1992, 91, 21–39 CrossRef CAS.
- C. F. Melius, J. A. Miller and E. M. Evleth, Unimolecular Reaction Mechanisms Involving C3H4, C4H4, and C6H6 Hydrocarbon Species, Symp. (Int.) Combust., 1992, 24, 621–628 CrossRef.
- L. K. Madden, A. M. Mebel, M.-C. Lin and C. F. Melius, Theoretical Study of the Thermal Isomerization of Fulvene to Benzene, J. Phys. Org. Chem., 1996, 9, 801–810 CrossRef CAS.
- A. M. Mebel, S. H. Lin, X. M. Yang and Y. T. Lee, Theoretical Study on the Mechanism of the Dissociation of Benzene. The C5H3 + CH3 Product Channel, J. Phys. Chem. A, 1997, 101, 6781–6789 CrossRef CAS.
- A. M. Mebel, M.-C. Lin, D. Chakraborty, J. Park, S. H. Lin and Y. T. Lee, Ab Initio Molecular Orbital/Rice–Ramsperger–Kassel–Marcus Theory Study of Multichannel Rate Constants for the Unimolecular Decomposition of Benzene and the H + C6H5 Reaction Over the Ground Electronic State, J. Chem. Phys., 2001, 114, 8421–8435 CrossRef CAS.
- V. V. Kislov, T. L. Nguyen, A. M. Mebel, S. H. Lin and S. C. Smith, Photodissociation of Benzene under Collision-Free Conditions: An Ab Initio/Rice–Ramsperger–Kassel–Marcus Study, J. Chem. Phys., 2004, 120, 7008–7017 CrossRef CAS PubMed.
- J. A. Miller and S. J. Klippenstein, The Recombination of Propargyl Radicals: Solving the Master Equation, J. Phys. Chem. A, 2001, 105, 7254–7266 CrossRef CAS.
- J. A. Miller and S. J. Klippenstein, The Recombination of Propargyl Radicals and Other Reactions on a C6H6 Potential, J. Phys. Chem. A, 2003, 107, 7783–7799 CrossRef CAS.
- M. Frenklach and E. D. Feigelson, Formation of Polycyclic Aromatic Hydrocarbons in Circumstellar Envelopes, Astrophys. J., 1989, 341, 372–384 CrossRef CAS.
- R. Zenobi, J.-M. Philippoz, R. N. Zare, M. R. Wing, J. L. Bada and K. Marti, Organic Compounds in the Forest Vale, H4 Ordinary Chondrite, Geochim. Cosmochim. Acta, 1992, 56, 2899–2905 CrossRef CAS PubMed.
- Y. M. Rhee, T. J. Lee, M. S. Gudipati, L. J. Allamandola and M. Head-Gordon, Charged Polycyclic Aromatic Hydrocarbon Clusters and the Galactic Extended Red Emission, Proc. Natl. Acad. Sci. U. S. A., 2007, 104, 5274–5278 CrossRef CAS PubMed.
- A. G. G. M. Tielens, Interstellar Polycyclic Aromatic Hydrocarbon Molecules, Annu. Rev. Astron. Astrophys., 2008, 46, 289–337 CrossRef CAS.
- P. Ehrenfreund and M. A. Sephton, Carbon Molecules in Space: From Astrochemistry to Astrobiology, Faraday Discuss., 2006, 133, 277–288 RSC.
- E. Herbst and E. F. Van Dishoeck, Complex Organic Interstellar Molecules, Annu. Rev. Astron. Astrophys., 2009, 47, 427–480 CrossRef CAS.
- J. L. Puget and A. Léger, A New Component of the Interstellar Matter: Small Grains and Large Aromatic Molecules, Annu. Rev. Astron. Astrophys., 1989, 27, 161–198 CrossRef CAS.
- P. Schmitt-Kopplin, Z. Gabelica, R. D. Gougeon, A. Fekete, B. Kanawati, M. Harir, I. Gebefuegi, G. Eckel and N. Hertkorn, High Molecular Diversity of Extraterrestrial Organic Matter in Murchison Meteorite Revealed 40 Years after Its Fall, Proc. Natl. Acad. Sci. U. S. A., 2010, 107, 2763–2768 CrossRef CAS PubMed.
- B. A. McGuire, R. A. Loomis, A. M. Burkhardt, K. L. K. Lee, C. N. Shingledecker, S. B. Charnley, I. R. Cooke, M. A. Cordiner, E. Herbst, S. Kalenskii, M. A. Siebert, E. R. Willis, C. Xue, A. J. Remijan and M. C. McCarthy, Detection of Two Interstellar Polycyclic Aromatic Hydrocarbons via Spectral Matched Filtering, Science, 2021, 371, 1265–1269 CrossRef CAS PubMed.
- L. M. Ziurys, The Chemistry in Circumstellar Envelopes of Evolved Stars: Following the Origin of the Elements to the Origin of Life, Proc. Natl. Acad. Sci. U. S. A., 2006, 103, 12274–12279 CrossRef CAS PubMed.
- A. W. Jasper and N. Hansen, Hydrogen-assisted Isomerizations of Fulvene to Benzene and of Larger Cyclic Aromatic Hydrocarbons, Proc. Combust. Inst., 2013, 34, 279–287 CrossRef CAS.
- C. S. McEnally, L. D. Pfefferle, B. Atakan and K. Kohse-Höinghaus, Studies of Aromatic Hydrocarbon Formation Mechanisms in Flames: Progress towards Closing the Fuel Gap, Prog. Energy Combust. Sci., 2006, 32, 247–294 CrossRef CAS.
- H. Richter and J. B. Howard, Formation and Consumption of Single-ring Aromatic Hydrocarbons and their Precursors in Premixed Acetylene, Ethylene and Benzene Flames, Phys. Chem. Chem. Phys., 2002, 4, 2038–2055 RSC.
-
A. G. G. M. Tielens and L. J. Allamandola, in Physics and Chemistry at Low Temperatures, ed. L. Khriachtchev, Pan Stanford Publishing, Singapore, 2011 Search PubMed.
- K. Fujishima, S. Dziomba, H. Yano, S. I. Kebe, M. Guerrouache, B. Carbonnier and L. J. Rothschild, The Non-Destructive Separation of Diverse Astrobiologically Relevant Organic Molecules by Customizable Capillary Zone Electrophoresis and Monolithic Capillary Electrochromatography, Int. J. Astrobiol., 2019, 18, 562–574 CrossRef CAS.
- L. J. Allamandola, S. A. Sandford and B. Wopenka, Interstellar Polycyclic Aromatic Hydrocarbons and Carbon in Interplanetary Dust Particles and Meteorites, Science, 1987, 237, 56–59 CrossRef CAS PubMed.
-
A. G. G. M. Tielens, C. van Kerckhoven, E. Peeters and S. Hony, Astrochemistry: From Molecular Clouds to Planetary Systems, presented in part at the Symposium - International Astronomical Union, 2000, vol. 197 Search PubMed.
- I. Cherchneff, J. R. Barker and A. G. G. M. Tielens, Polycyclic Aromatic Hydrocarbon Formation in Carbon-Rich Stellar Envelopes, Astrophys. J., 1992, 401, 269–287 CrossRef CAS.
- M. Cohen, A. G. G. M. Tielens and J. D. Bregman, Mid-Infrared Spectra of WC 9 Stars: The Composition of Circumstellar and Interstellar Dust, Astrophys. J., 1989, 344, L13–L16 CrossRef CAS.
- E. R. Micelotta, A. P. Jones and A. G. G. M. Tielens, Polycyclic Aromatic Hydrocarbon Processing in Interstellar Shocks, Astron. Astrophys., 2010, 510, A36 CrossRef.
- N. Hansen, B. Yang, M. Braun-Unkhoff, A. Ramirez and G. Kukkadapu, Molecular-growth Pathways in Premixed Flames of Benzene and Toluene Doped with Propyne, Combust. Flame, 2022, 243, 112075 CrossRef CAS.
- A. Matsugi and A. Miyoshi, Computational Study on the Recombination Reaction between Benzyl and Propargyl Radicals, Int. J. Chem. Kinet., 2012, 44, 206–218 CrossRef CAS.
- M. Agúndez, C. Cabezas, B. Tercero, N. Marcelino, J. D. Gallego, P. de Vicente and J. Cernicharo, Discovery of the Propargyl Radical (CH2CCH) in TMC-1: One of the Most Abundant Radicals Ever Found and a Key Species for Cyclization to Benzene in Cold Dark Clouds, Astron. Astrophys., 2021, 647, L10 CrossRef PubMed.
- M. V. Zagidullin, R. I. Kaiser, D. P. Porfiriev, I. P. Zavershinskiy, M. Ahmed, V. N. Azyazov and A. M. Mebel, Functional Relationships between Kinetic, Flow, and Geometrical Parameters in a High-Temperature Chemical Microreactor, J. Phys. Chem. A, 2018, 122, 8819–8827 CrossRef CAS PubMed.
- R. I. Kaiser, L. Zhao, W. Lu, M. Ahmed, V. S. Krasnoukhov, V. N. Azyazov and A. M. Mebel, Unconventional Excited-State Dynamics in the Concerted Benzyl (C7H7) Radical Self-Reaction to Anthracene (C14H10), Nat. Commun., 2022, 13, 786 CrossRef CAS PubMed.
- L. Zhao, W. Lu, M. Ahmed, M. V. Zagidullin, V. N. Azyazov, A. N. Morozov, A. M. Mebel and R. I. Kaiser, Gas-Phase Synthesis of Benzene via the Propargyl Radical Self-Reaction, Sci. Adv., 2021, 7, eabf0360 CrossRef CAS PubMed.
- F. Qi, Combustion Chemistry Probed by Synchrotron VUV Photoionization Mass Spectrometry, Proc. Combust. Inst., 2013, 34, 33–63 CrossRef CAS.
- L. Zhao, R. I. Kaiser, B. Xu, U. Ablikim, M. Ahmed, M. V. Zagidullin, V. N. Azyazov, A. H. Howlader, S. F. Wnuk and A. M. Mebel, VUV Photoionization Study of the Formation of the Simplest Polycyclic Aromatic Hydrocarbon: Naphthalene (C10H8), J. Phys. Chem. Lett., 2018, 9, 2620–2626 CrossRef CAS PubMed.
-
Y. Li, J. Yang and Z. Cheng, Photoionization Cross Section Database (Version 2.0), National Synchrotron Radiation Laboratory, Hefei, China, 2017, http://flame.nsrl.ustc.edu.cn/database/data.php Search PubMed.
- V. S. Krasnoukhov, P. S. Pivovarov, M. V. Zagidullin, V. N. Azyazov, A. M. Mebel and A. N. Morozov, Formation of Two-ring Polycyclic Aromatic Hydrocarbons via the Recombination of Benzyl and Propargyl Radicals under the Circumstellar Envelopes Conditions of Asymptotic Giant Branch Stars, Astron. Rep., 2022, 66, 811–826 CrossRef CAS.
- D. W. Kohn, H. Clauberg and P. Chen, Flash Pyrolysis Nozzle for Generation of Radicals in a Supersonic Jet Expansion, Rev. Sci. Instrum., 1992, 63, 4003–4005 CrossRef CAS.
-
NIST Chemistry WebBook, SRD 69, https://webbook.nist.gov/chemistry/ Search PubMed.
- A. M. Mebel, Y. Georgievskii, A. W. Jasper and S. J. Klippenstein, Pressure-Dependent Rate Constants for PAH Growth: Formation of Indene and Its Conversion to Naphthalene, Faraday Discuss., 2016, 195, 637–670 RSC.
- D. S. N. Parker, B. B. Dangi, R. I. Kaiser, A. Jamal, M. N. Ryazantsev, K. Morokuma, A. Korte and W. Sander, An Experimental and Theoretical Study on the Formation of 2-Methylnaphthalene (C11H10/C11H3D7) in the Reactions of the Para-Tolyl (C7H7) and Para-Tolyl-d7 (C7D7) with Vinylacetylene (C4H4), J. Phys. Chem. A, 2014, 118, 2709–2718 CrossRef CAS PubMed.
- T. Yang, L. Muzangwa, R. I. Kaiser, A. Jamal and K. Morokuma, A Combined Crossed Molecular Beam and Theoretical Investigation of the Reaction of the Meta-Tolyl Radical with Vinylacetylene–toward the Formation of Methylnaphthalenes, Phys. Chem. Chem. Phys., 2015, 17, 21564–21575 RSC.
- M. Frenklach, D. W. Clary, W. C. Gardiner Jr and S. E. Stein, Detailed Kinetic Modeling of Soot Formation in Shock-Tube Pyrolysis of Acetylene, Symp. (Int.) Combust., 1985, 20, 887–901 CrossRef.
- M. Frenklach, Reaction Mechanism of Soot Formation in Flames, Phys. Chem. Chem. Phys., 2002, 4, 2028–2037 RSC.
- M. Frenklach, On the Driving Force of PAH Production, Symp. (Int.) Combust., 1989, 22, 1075–1082 CrossRef.
- R. I. Kaiser, L. Zhao, W. Lu, M. Ahmed, M. M. Evseev, V. N. Azyazov, A. M. Mebel, R. K. Mohamed, F. R. Fischer and X. Li, Gas-Phase Synthesis of Racemic Helicenes and Their Potential Role in the Enantiomeric Enrichment of Sugars and Amino Acids in Meteorites, Phys. Chem. Chem. Phys., 2022, 24, 25077–25087 RSC.
- R. I. Kaiser and N. Hansen, An Aromatic Universe–A Physical Chemistry Perspective, J. Phys. Chem. A, 2021, 125, 3826–3840 CrossRef CAS PubMed.
- R. I. Kaiser, D. S. N. Parker and A. M. Mebel, Reaction Dynamics in Astrochemistry: Low-Temperature Pathways to Polycyclic Aromatic Hydrocarbons in the Interstellar Medium, Annu. Rev. Phys. Chem., 2015, 66, 43–67 CrossRef CAS PubMed.
- A. M. Mebel and R. I. Kaiser, Formation of Resonantly Stabilised Free Radicals via the Reactions of Atomic Carbon, Dicarbon, and
Tricarbon with Unsaturated Hydrocarbons: Theory and Crossed Molecular Beams Experiments, Int. Rev. Phys. Chem., 2015, 34, 461–514 Search PubMed.
Footnote |
† Electronic supplementary information (ESI) available: Experimental and computational methods, PIE curve for the species (m/z = 130) in the benzyl + propargyl system (Fig. S1), computed ionization Franck–Condon factors and integrated PIE curves for three isomers of C10H8 (Fig. S2) and for two isomers of C10H10 (Fig. S3), calculated total and individual product channel rate constants for the benzyl–propargyl radical–radical reaction (Fig. S4 and S5), and optimized Cartesian coordinates (Å) and vibrational frequencies (cm−1) for all intermediates, transition states, reactants and products involved in the reaction of benzyl + propargyl system in the format of an input file for RRKM–ME calculations employing the MESS package (Table S1). See DOI: https://doi.org/10.1039/d3sc00911d |
|
This journal is © The Royal Society of Chemistry 2023 |