DOI:
10.1039/D3SC00563A
(Edge Article)
Chem. Sci., 2023,
14, 5503-5509
Orthometric multicolor encoded hybridization chain reaction amplifiers for multiplexed microRNA profiling in living cells†
Received
1st February 2023
, Accepted 19th April 2023
First published on 20th April 2023
Abstract
Multiplexed microRNA (miRNA) profiling of more than four types in living cells is challenging due to fluorescent spectral overlap, representing a significant limitation in studying the complex interactions related to the occurrence and development of diseases. Herein, we report a multiplexed fluorescent imaging strategy based on an orthometric multicolor encoded hybridization chain reaction amplifier named multi-HCR. The targeting miRNA can trigger this multi-HCR strategy due to the specific sequence recognition, and then its self-assembly to amplify the programmability signals. We take the four-colored chain amplifiers, showing that the multi-HCR can form 15 combinations simultaneously. In a living process of hypoxia-induced apoptosis and autophagy under complicated mitochondria and endoplasmic reticulum stress, the multi-HCR demonstrates excellent performance in detecting eight different miRNA changes. The multi-HCR provides a robust strategy for simultaneously profiling multiplexed miRNA biomarkers in studying complicated cellular processes.
Introduction
Living cells are the most precise molecular machines, integrating internal gene networks with external cellular behavior in response to multiple stimuli.1 High multiplexing fluorescence (FL) imaging capability is crucial for studying the interacting regulatory elements in a biological system's developmental and pathological processes.2 However, performing parallel mapping of multiple interactions is challenging owing to the emission spectral overlap.3 MicroRNAs (miRNAs) are novel biomarkers for multiple diseases, including cancers and neurodegenerative and cardiovascular diseases.4 They are 18–22 nucleotide endogenous non-coding RNAs that govern the expression of target genes by recruiting the RNA-induced silencing complex to the specific sequences at the 3′-UTR of target mRNAs and disrupting the stability of mRNA.5 The great clinical potential of miRNAs for recognizing typical disease signatures depends mainly upon the ability to parallelly map the expression profile and concentration changes of multiple miRNAs.2a,6
Over the past few decades, in situ fluorescent hybridization has been an indispensable tool for studying genetic regulation in a morphological context.7 Current multiplexed methods, such as the rolling circle amplification (RCA),3b Exchange-Points Accumulation in Nanoscale Topography technique (Exchange-PAINT),8 sequential hybridization9 and split-probe strategy with multiplexed fluorescence in situ hybridization (split-FISH),10 require serial amplification with enzyme-dependent performance, limiting their use to fixed cells. In contrast, enzyme-free amplification techniques such as hybridization chain reaction (HCR) and catalyzed hairpin amplification (CHA) are more suitable for living cell detection. Significantly, the limit of detection of HCR-based miRNA imaging in living cells can be down to attomole (aM) levels,11 satisfying the high sensitivity requirement of intracellular miRNA (picomolar (pM)–femtomolar (fM) range) analysis.2a,12 Our group also previously developed several HCR strategies for miRNA imaging;13 however, owing to the spectral overlap between fluorophores,3a simultaneously mapping more than four different miRNAs in living cells is challenging.
Herein, we developed an orthometric multicolor encoded hybridization chain HCR amplifier (multi-HCR) to implement 15 combinations for simultaneously mapping miRNAs in living cells. The multi-HCR consists of metastable nucleic acid hairpins (H) modified with four kinds of fluorophores (Alexa Fluor (AF) 405, AF488, AF594, and AF647). The fluorescent hairpins were separately modified on a biodegradable mesoporous silica nanoparticles (DMSN) vector, forming a spherical structure of DMSN@H. The DMSN vector endows the hairpins with high cell penetration ability.14 After cellular uptake, the endogenous glutathione (GSH) decomposes DMSN to release the hairpins.15 Target miRNAs complementary to the hairpin probes trigger HCR and self-assemble into encoded tethered fluorescent amplifiers. Owing to the sequence-specific and ordered-dye designation, these hairpins can be self-assembled in an orthometric way to simultaneously achieve 15 combinations for miRNA imaging in a colorectal cancer cell model (Scheme 1). Excellent performance was realized when imaging eight different miRNAs under hypoxia-induced mitochondria (Mito) stress and endoplasmic reticulum (ER) stress cross-talking in related reactive oxygen species (ROS), apoptosis, and autophagy pathways.
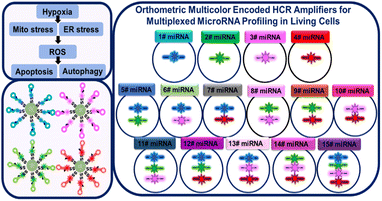 |
| Scheme 1 Schematic presentation of orthometric multi-HCR amplifiers for multiplexed miRNAs profile in living cells. | |
Results and discussion
HCR orthometric assembly feasibility analysis. Inspired by the logical combination of FL signals,6a,16 the HCR extension hairpin chains containing different FL dyes were designed to programmatically self-assemble and be recognized in an orthometric combination manner during the HCR reaction procedure (Fig. S1†). To test this hypothesis, a series of tests for miRNA-155-triggered FL hairpins with similar self-dimer structures were designed (Fig. S2 and Table S1†). According to the base complementarity reaction law and the principle of the classical HCR reaction, the introduction of an initiator strand (target miRNA) triggers a chain reaction of alternating kinetic escapes by the two hairpin species corresponding to “polymerization” into a nicked double helix, then amplification of the initiator recognition event continues until the supply of H1 or H2 is exhausted, forming DNA polymers,17 as depicted by transmission electron microscopy (TEM) (Fig. 1a and S3†). Native polyacrylamide gel electrophoresis (native-PAGE) demonstrated the excellent specificity of the HCR assembly (Fig. S4†). FL analysis using AF488-labeled miR-155 probes showed its excellent base-mismatch discrimination ability (Fig. 1b), and it could respond in a target concentration-dependent manner (Fig. S5†). In principle, using three fluorescent-dye (AF488, AF594, AF647)-labeled hairpin structures, it could be orthogonally self-assembled into seven different encoded amplifiers through the equation of N = C31 + C32 + C33; N = 7 (Fig. 1c and S6†). Accordingly, four different fluorescent-dye (AF405, AF488, AF594, AF647)-labeled hairpin structures could be self-assembled into 15 kinds of encoded amplifiers (N = C41 + C42 + C43 + C44; N = 15) with distinct FL spectra (Fig. 1d, S7, S8 and Table S2†), which holds great potential for multiplexed miRNA profiling. Although there was Förster Resonance Energy Transfer (FRET) in the adjacent dyes, its effect was weak; it could not entirely disappear a color;3b,18 the FRET had a limited effect on the classification ability of the multi-HCR system (Fig. 1d). This highlights the novelty of the multi-HCR system in that its multiplexed coding capability comes from the color combinations, not intensity, which makes it an easy-to-operate tool for multiple miRNA profiling.
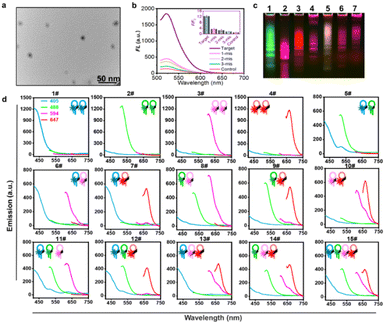 |
| Fig. 1 Feasibility analysis of the multi-HCR assembly. (a) Representative TEM images for HCR self-assembled amplifier. (b) Base-mismatched analysis toward the complementary target (miRNA-155, 100 nM). Single-base-mismatched strand (1 mis, 100 nM); two-base-mismatched strand (2 mis, 100 nM), three-base-mismatched strand (3 mis, 100 nM), and control (PBS). (c) Fluorescent native-PAGE electrophoresis (2 μM) of three-color multi-HCR encoded amplifiers. (d) FL emission spectra of self-assembled encoded amplifiers from four different dye-labeled hairpins in response to target miRNA-155. Each experiment was repeated three times. | |
GSH response and target miRNA-triggered multi-HCR in living cells
As the primary intracellular antioxidant inside cells, GSH plays a critical role in mammalian cell function. It was reported that the GSH concentration inside the cells is about 1 × 10−3–10 × 10−3 M,19 as compared with 2 × 10−6–40 × 10−6 M in blood and other body fluids.20 What is more, cancer cells have about four-fold higher GSH than normal cells,21 indicating that GSH could also be used as an effective signal molecule for enhancing the specificity of tumor detection. On the other hand, to improve the cellular transfection efficiency of the DNA hairpin probes,14a,22 we modified the HCR hairpins on biodegradable DMSN by a disulfide bond,23 forming spherical nucleic acid structures of DMSN@H, termed multi-HCR (Fig. 2a), which has been reported to possess high stability, excellent biocompatibility, and high cell entry ability.24 The loading efficiency for the hairpins was calculated to be 48%. The TEM results in Fig. 2b show that under the stimulation of 10 mM GSH, the DMSN vectors were degraded and the hairpins were released from the DMSN vectors, and the co-existing H1 and H2 were self-assembled into spherical DNA gel amplifiers in the presence of target miRNA, confirming that the DMSN@H was activated in a GSH-responsive manner. The Cell-Counting-Kit-8 (CCK-8) test verified good biosafety for living colon cancer cells (Hct116) (Fig. S9†), which is very important for nanoparticle probes to be used in living cell imaging. Confocal laser scanning microscopy (CLSM) analysis confirmed that the DMSN@H had no FL (purple box) unless it had entered the cells (Fig. 2c). Notably, when the Hct116 cells were pretreated with GSH inhibitor L-buthionine sulfoximine (BSO),23c the mean FL intensity decreased dramatically (∼5.4 fold), further verifying the GSH-mediated release of hairpins probes, which provided endogenous GSH-activatable and spatially controllable miRNA imaging. Moreover, the FL intensity was observed to increase with the reaction time, and it reached a plateau after 24 h incubation with the cells (Fig. 2d and e). The AF488-labeled DMSN@H barcodes toward miRNA-155 were put in pH 7.2, pH 6.0, and pH 5.0 cell culture mediums for 4 h. The size and FL intensity were measured, as shown in Fig. S10;† the results show that the sizes and FL were stable under different pH. These results strongly confirmed the GSH-responsive and target miRNA-triggered self-assembly-encoded process of DMSN@H barcodes in living tumor cells.
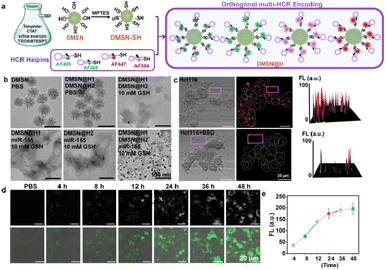 |
| Fig. 2 GSH-activated and miRNA target-triggered multi-HCR barcode. (a) Assembly process diagram for multi-HCR. (b) Representative TEM of DMSN@H after 24 h treatment in different conditions. (c) Representative CLSM images of the multi-HCR-treated Hct116 cells with or without GSH inhibitor, BSO. The 3D FL intensity surface profiling pictures were acquired by ImageJ software (version 1.52i, USA). (d) Representative CLSM images and (e) mean FL intensity of the multi-HCR-treated cells at different incubation times. Each experiment was repeated three times. | |
The encoding ability of multi-HCR amplifiers in living cells
We further tested the intracellular encoding ability of the multi-HCR system. As illustrated in Fig. 3a, the intracellular DMSN@H decomposed in response to endogenous GSH and released the hairpins loaded with different color dyes. The presence of the target miRNA initiated the cross-opening of two specific DNA hairpins, yielding double helices that are analogous to alternating copolymers, and different colored dyes were enclosed into the barcode for classification. As shown in Fig. 3b, DMSN@H (Table S3†) was mixed and treated with the living Hct116 cells for intracellular miRNA-155 imaging. The encoding composition could be acquired by CLSM sequencing scanning the four FL channels with an appropriate exciting laser and emission filter. In Fig. 3c, Gaussian curve analysis of the full width at half maximum (FWHM) of two adjacent dots in the green box of Fig. 3b showed that the multi-HCR barcodes could be well distinguished using the CLSM system. The 15 different kinds of amplifiers with distinct emission characteristics were detected synchronously. The dot analysis shown in Fig. 3d verified that the multi-HCR approach is a simple way to distinguish miRNA types based on the encoded color composition but not based on the intensity. These demonstrated the excellent encoding ability of multi-HCR for in situ multiplexed miRNA analysis.
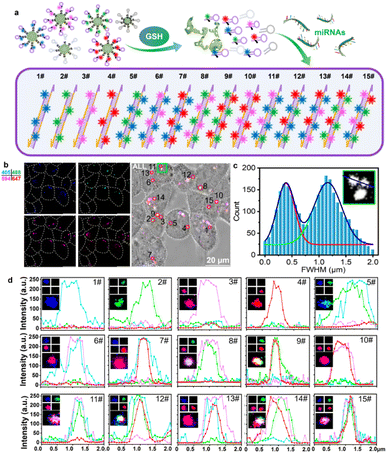 |
| Fig. 3 Multi-HCR encoded in living cells. (a) Illustration of the multi-HCR barcode encoded in living cells. (b) Representative CLSM images for the four-color DMSN@H simultaneously encoded in living cells. (c) Gaussian curve analysis of the adjacent FL dots in the green box of (b). (d) Representative images and FL curves of the 15 kinds of barcodes. Each experiment was repeated three times. | |
Multiplexed miRNA profiling under hypoxia-induced Mito/ER stress and apoptosis/autophagy processes
Finally, we attempted to simultaneously detect multiple miRNAs that vary during hypoxia-induced Mito/ER stress processes and apoptosis/autophagy responses. Hypoxia can induce Mito and ER stress, leading to apoptosis and autophagy in living cells. As critical regulators, miRNA expression alters in response to the hypoxia to influence downstream cellular functions involved in apoptosis and autophagy.25 Hypoxia adaptation is essential for cancer cell progression; however, little research has investigated the changes in multiplexed miRNA profiles in hypoxia-induced Mito/ER stress and apoptosis/autophagy. First, hypoxia-induced Mito–ER stress and apoptosis/autophagy experiments were performed to prove the elaborate cell behavior alterations in the hypoxic microenvironment (Fig. S11†). Then, we attempted to simultaneously monitor eight reported hypoxic-responsive miRNAs in living cancer cells using the orthogonal multi-HCR strategy (Fig. 4a and Table S4†). Different multi-HCR chains for eight types of miRNAs involved in these processes26 were designed to map multiplexed miRNAs (Fig. S12, S13 and Table S5†). The orthogonal FL assay (Fig. 4b) and native-PAGE electrophoresis (Fig. S14†) showed good specific recognition. Then, the DMSN@H mixture was incubated with Hct116 cells in normal and hypoxic conditions. As expected, CLSM images with different distinct emission dots were obtained, indicating a successful GSH response and target miRNA-triggered self-assembly of amplifiers in living Hct116 cells (Fig. 4c and S15†). The decoded dots presented in Fig. S16† strongly demonstrated the ability to image multiplexed miRNAs. Compared with the cells in normal conditions, changes in the FL intensities of most of the amplifiers were observed in hypoxic conditions. The FL intensity assigned to miRNA-211, miRNA-346, and miRNA-210 separately increased 2.1, 2.65, 3.52 times, respectively, while the intensity for miRNA-153, miRNA-455, miRNA-141, miRNA-181 separately decreased by 3.2, 1.43, 1.29, 1.40 times, respectively. Only the miRNA-30c-2 had no statistically significant change. The FL intensity change trends were consistent with the quantitative reverse transcriptase polymerase chain reaction (qPCR) results (Fig. 4d) and previous reports.26a,b,i,j,27 These results confirmed the robust performance of the designed multi-HCR for multiplexed miRNA profiling in developmental and pathological processes.
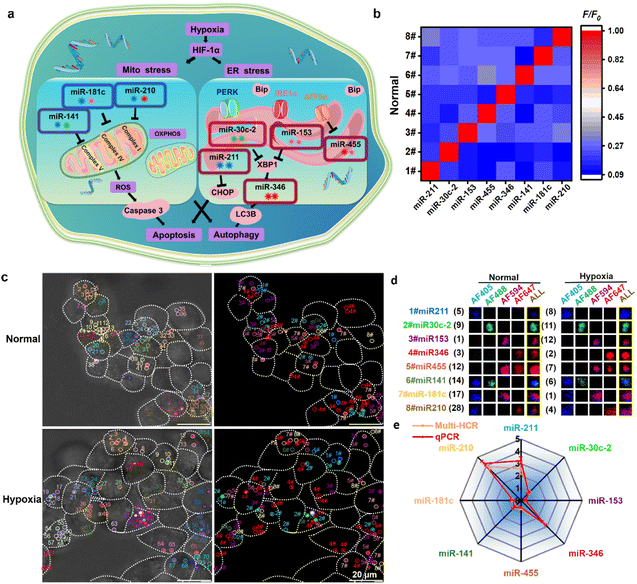 |
| Fig. 4 Multiplexed miRNA profiling under hypoxia-induced Mito/ER stress and apoptosis/autophagy processes. (a) Schematic presentation of the hypoxia-induced miRNAs alteration. (b) Normalized heatmap for the specific test of the eight hypoxia-induced miRNAs. (c) Representative CLSM images for simultaneously profiling eight miRNAs using multi-HCR amplifiers. (d) Representative decoding and calculation process for the eight miRNAs in living cells (e) comparison of miRNA profiles tested by multi-HCR and qPCR methods. Each experiment was repeated three times. | |
Experimental
Synthesis of 80 nm biodegradable mesoporous silicon nano-vector (DMSN)
The DMSN was synthesized using cetyltrimethylammoniumtosylate (CTAT) as a template and tetraethyl orthosilicate (TEOS) with bis[3-(triethoxysilyl)propyl]tetrasulfide (BTESPT) as biodegradable silica sources.23b,28 0.6 g of CTAT, 0.15 g of triethanolamine (TEA), and 40 mL of ddH2O were stirred at 80 °C for 30 min. Then, a solution of 1.0 g of BTESPT and 4.0 g of TEOS was added dropwise to the solution. The resulting mixture was stirred at 1000 rpm at 70 °C for 2 h. The products were collected by centrifugation at 10
000 rpm for 20 min, washed with anhydrous ethanol, and refluxed in the anhydrous ethanol solution for 24 h. Finally, the DMSN was washed, collected, and dried for further use.
Synthesis of multi-HCR barcodes (DMSN@H)
The DMSN was modified with thiol groups (–SH) as follows:29 10 mg of DMSN was ultrasonically dispersed into 2 mL of anhydrous ethanol containing 0.03 g of CTAT, 0.008 g of TEA, 0.1 g of TEOS, and 0.1 g of METES. The mixture was stirred at 1000 rpm for 30 min at room temperature, then centrifuged and the sediment was washed three times using anhydrous ethanol. 10 μL of different HCR hairpins (100 μM) were separately mixed with 90 μL of Tris–HCl buffer (pH 7.4, 10 mM MgCl2), and heated at 95 °C for 5 min, then kept at 37 °C for 2 h to stabilize the hairpin structure. 100 μL of DMSN-SH (10 mg mL−1) was mixed with the above HCR hairpin buffer under gently shaken for 24 h at room temperature. Finally, the obtained DMSN@H was washed and resuspended in 1 mL of PBS buffer and kept at 4 °C for further use.
Multiplexed fluorescence assay in liquid solution
To assess the feasibility of detecting orthorhombic HCR hairpins assembled for 15 kinds of color compositions, different colors of hairpins were equally mixed at a total concentration of 120 nM and incubated with the target miRNA-155 (120 nM) at 37 °C for 4 h. For the specificity assay of the eight HCR hairpin pairs targeting eight kinds of miRNAs, 100 μL of total hairpins (120 nM) was incubated with 100 μL of targeting miRNAs (120 nM) at 37 °C for 4 h. The fluorescence intensity was measured on a Hitachi F-4500 fluorescence spectrometer (Tokyo, Japan). The AF405, AF488, AF595, and AF647 were excited at 402 nm, 488 nm, 594 nm, and 633 nm, and emissions peaks were recorded at 419 nm, 610 nm, and 665 nm, respectively.
Cell culture
For 0.2% O2 incubation (hypoxia group), 1 × 104 Hct116 cells were cultured in an AnaerPack™-Anaero box (2.5 L). For 5% O2 treatment, the cells were settled in an AnaerPack™-MicroAero (2.5 L). Other operations were similar to those in a normoxic environment (normal group).30 All the cells were incubated in DMEM containing 10% FBS and 1% penicillin/streptomycin at 37 °C in a humidified atmosphere containing 5% CO2.
CLSM imaging and analysis
Briefly, before incubating with the multi-HCR nanoparticles, 1 × 104 Hct116 cells were cultured under normal (21% O2) and hypoxic (0.2% O2) environments for 48 h. Then they were treated with the multi-HCR barcodes at a total concentration of 100 μg mL−1 (or 240 nM total HCR probes) per confocal dish of cells for 24 h at 37 °C under normal (21% O2) and hypoxic (0.2% O2) environments. The resulting cells were washed twice using PBS, and fresh DMEM (1 mL) was added. The scanning was in sequencing mode: AF405 was excited with a 405 nm laser line and detected with a 430–450 nm band-pass filter. AF488 was excited at 488 nm, with emission collected at 510–530 nm. AF594 and AF647 were excited with 561 nm and 633 nm lasers, respectively, then 590–620 nm and 650–700 nm filters were used for FL detection. Finally, the acquired FL barcodes were analyzed using ImageJ software (version 1.52i, USA), and a positive color composition was confirmed by subtracting three times the background fluorescence intensity.23
Statistical analysis
All of the results have been acquired by performing at least three independent experiments. All data are expressed in this manuscript as mean ± SD. A two-tailed Student's t-test was used to analyze the differences between the two groups. Asterisks indicate significant differences (*p < 0.05, **p < 0.01, ***p < 0.001). The analysis was performed using Origin 2018 software (OriginLab Inc., USA).
Conclusions
Multiplexed in situ analysis of interacting molecules in living cells is of great importance for revealing cell physiopathology, clinical diagnostic applications, and biosensing. We report an encoded multi-HCR amplifier based on orthogonal amplification cascades with hybridization chain reactions. It comprises different colored metastable hairpins separately modified on mesoporous silica nanoparticles. After cellular uptake, the GSH-responsive hairpins are released and target miRNA-triggered orthogonal amplification, enabling self-assembly of 15 fluorescent HCR amplifiers for miRNA profiling. Excellent performance is achieved when imaging eight target miRNAs in hypoxia-induced Mito/ER stress and apoptosis and autophagy. The multi-HCR provides a robust tool for simultaneously profiling multiplexed biomarkers, facilitating a deeper understanding of interacting regulatory elements in developmental and pathological processes. It should be noted that connecting the barcoded nucleic acid chains with antibodies can easily extend the strategy to the high-throughput detection of protein biomarkers in living cells.
Author contributions
H. F. D. conceived the project and supervised all the experiments. Y. Y. Z. and Y. Y. W. did the electrophoresis experiments. W. W. and F. Y. performed FL analysis. S. S. Y. and J. Z. L. captured the TEM images. W. W and Y. F. Z did the qPCR test. W. W. and L. P. Z performed cell culture and confocal microscopy analysis. H. F. D. and W. W. wrote the first edition of this manuscript.
Conflicts of interest
There are no conflicts to declare.
Acknowledgements
The work was supported by the Excellent Young Scientists Fund (22022407), Special Foundation for State Major Research Program of China (Grant 2022YFB3207202), Guangdong Province Pearl River Team (2021ZT09C289), Shenzhen Key Laboratory for Nano-Biosensing Technology (ZDSYS20210112161400001). 2021 Stability support plan of Shenzhen University (8940206/0200). We thank Instrumental Analysis Center of Shenzhen University for the assistance with the Electron Microscope/Nuclear Magnetic Resonance spectroscope/Mass Spectrometer/Confocal Microscope/Small Animal Diagnostics Imaging/Material Characterization technical support.
References
-
(a) X. Liu and Y.-T. Chang, Chem. Soc. Rev., 2022, 51, 1573–1591 RSC;
(b) J. Shen, Y. Li, H. Gu, F. Xia and X. Zuo, Chem. Rev., 2014, 114, 7631–7677 CrossRef CAS PubMed.
-
(a) T. Jet, G. Gines, Y. Rondelez and V. Taly, Chem. Soc. Rev., 2021, 50, 4141–4161 RSC;
(b) Z. Xiang, J. Zhao, D. Yi, Z. Di and L. Li, Angew. Chem., 2021, 60, 22659–22663 CrossRef CAS PubMed.
-
(a) E. Lubeck and L. Cai, Nat. Methods, 2012, 9, 743–748 CrossRef CAS PubMed;
(b) R. Deng, K. Zhang, L. Wang, X. Ren, Y. Sun and J. Li, Chem, 2018, 4, 1373–1386 CrossRef CAS.
-
(a) M. Hill and N. Tran, Trends Cancer, 2018, 4, 465–468 CrossRef CAS PubMed;
(b) H. Galagali and J. K. Kim, Curr. Opin. Cell Biol., 2020, 67, 118–140 CrossRef CAS PubMed.
-
(a) T. Treiber, N. Treiber and G. Meister, Nat. Rev. Mol. Cell Biol., 2019, 20, 5–20 CrossRef CAS PubMed;
(b) S. Cai, T. Pataillot-Meakin, A. Shibakawa, R. Ren, C. L. Bevan, S. Ladame, A. P. Ivanov and J. B. Edel, Nat. Commun., 2021, 12, 3515 CrossRef CAS PubMed.
-
(a) D. Wang, S. Li, Z. Zhao, X. Zhang and W. Tan, Angew. Chem., 2021, 60, 1–6 CrossRef;
(b) T. Ueda, S. Volinia, H. Okumura, M. Shimizu, C. Taccioli, S. Rossi, H. Alder, C.-g. Liu, N. Oue, W. Yasui, K. Yoshida, H. Sasaki, S. Nomura, Y. Seto, M. Kaminishi, G. A. Calin and C. M. Croce, Lancet Oncol., 2010, 11, 136–146 CrossRef CAS PubMed;
(c) X.-H. Shi, X. Li, H. Zhang, R.-Z. He, Y. Zhao, M. Zhou, S.-T. Pan, C.-L. Zhao, Y.-C. Feng, M. Wang, X.-J. Guo and R.-Y. Qin, Sci. Rep., 2018, 8, 7638 CrossRef PubMed;
(d) F. Kern, L. Krammes, K. Danz, C. Diener, T. Kehl, O. Küchler, T. Fehlmann, M. Kahraman, S. Rheinheimer, E. Aparicio-Puerta, S. Wagner, N. Ludwig, C. Backes, H.-P. Lenhof, H. von Briesen, M. Hart, A. Keller and E. Meese, Nucleic Acids Res., 2020, 49, 127–144 CrossRef PubMed;
(e) B. Liu, B. Wang, X. Zhang, R. Lock, T. Nash and G. Vunjak-Novakovic, Sci. Transl. Med., 2021, 13, eabd0914 CrossRef CAS PubMed.
- J. B. Lawrence, R. H. Singer and L. M. Marselle, Cell, 1989, 57, 493–502 CrossRef CAS PubMed.
-
(a) S. S. Agasti, Y. Wang, F. Schueder, A. Sukumar, R. Jungmann and P. Yin, Chem. Sci., 2017, 8, 3080–3091 RSC;
(b) P. Y. Weidong Xu and M. Dai, Angew. Chem., 2018, 57, 14075–14079 CrossRef PubMed.
- E. Lubeck, A. F. Coskun, T. Zhiyentayev, M. Ahmad and L. Cai, Nat. Methods, 2014, 11, 360–361 CrossRef CAS PubMed.
- J. J. L. Goh, N. Chou, W. Y. Seow, N. Ha, C. P. P. Cheng, Y.-C. Chang, Z. W. Zhao and K. H. Chen, Nat. Methods, 2020, 17, 689–693 CrossRef CAS PubMed.
-
(a) H. M. T. Choi, J. Y. Chang, L. A. Trinh, J. E. Padilla, S. E. Fraser and N. A. Pierce, Nat. Biotechnol., 2010, 28, 1208–1212 CrossRef CAS PubMed;
(b) M. Schwarzkopf and N. A. Pierce, Nucleic Acids Res., 2016, 44, e129 Search PubMed;
(c) H. M. T. Choi, V. A. Beck and N. A. Pierce, ACS Nano, 2014, 8, 4284–4294 CrossRef CAS PubMed.
- J. Liu, T. Zheng and Y. Tian, Angew. Chem., 2019, 58, 7757–7761 CrossRef CAS PubMed.
-
(a) F. Yang, Y. Cheng, Y. Cao, H. Dong, H. Lu, K. Zhang, X. Meng, C. Liu and X. Zhang, Chem. Sci., 2019, 10, 1709–1715 RSC;
(b) Y. Cao, H. Dong, S. Pu and X. Zhang, Nano Res., 2018, 11, 4074–4081 CrossRef CAS.
-
(a) M.-H. Kim, H.-K. Na, Y.-K. Kim, S.-R. Ryoo, H. S. Cho, K. E. Lee, H. Jeon, R. Ryoo and D.-H. Min, ACS Nano, 2011, 5, 3568–3576 CrossRef CAS PubMed;
(b) P. Mora-Raimundo, D. Lozano, M. Benito, F. Mulero, M. Manzano and M. Vallet-Regí, Adv. Sci., 2021, 8, 2101107 CrossRef CAS PubMed;
(c) Y. Zhou, G. Quan, Q. Wu, X. Zhang, B. Niu, B. Wu, Y. Huang, X. Pan and C. Wu, Acta Pharm. Sin. B, 2018, 8, 165–177 CrossRef PubMed.
- C. Wang, H. Lin, X. Ge, J. Mu, L. Su, X. Zhang, M. Niu, H. Yang and J. Song, Adv. Funct. Mater., 2021, 31, 2009942 CrossRef CAS.
-
(a) M. J. Lajoie, S. E. Boyken, A. I. Salter, J. Bruffey, A. Rajan, R. A. Langan, A. Olshefsky, V. Muhunthan, M. J. Bick, M. Gewe, A. Quijano-Rubio, J. Johnson, G. Lenz, A. Nguyen, S. Pun, C. E. Correnti, S. R. Riddell and D. Baker, Science, 2020, 369, 1637–1643 CrossRef CAS PubMed;
(b) Q. Yang, J. Li, X. Wang, H. Xiong and L. Chen, Anal. Chem., 2019, 91, 6561–6568 CrossRef CAS PubMed;
(c) M. Xiao, K. Zou, L. Li, L. Wang, Y. Tian, C. Fan and H. Pei, Angew. Chem., Int. Ed., 2019, 58, 15448–15454 CrossRef CAS PubMed.
-
(a) R. M. Dirks and N. A. Pierce, Proc. Natl. Acad. Sci. U. S. A., 2004, 101, 15275–15278 CrossRef CAS PubMed;
(b) Z. Wu, G.-Q. Liu, X.-L. Yang and J.-H. Jiang, J. Am. Chem. Soc., 2015, 137, 6829–6836 CrossRef CAS PubMed.
- Y. Li, Y. T. H. Cu and D. Luo, Nat. Biotechnol., 2005, 23, 885–889 CrossRef CAS PubMed.
- C. Hwang, A. J. Sinskey and H. F. Lodish, Science, 1992, 257, 1496–1502 CrossRef CAS PubMed.
- R. Hong, G. Han, J. M. Fernández, B.-j. Kim, N. S. Forbes and V. M. Rotello, J. Am. Chem. Soc., 2006, 128, 1078–1079 CrossRef CAS PubMed.
- M. H. Lee, Z. Yang, C. W. Lim, Y. H. Lee, S. Dongbang, C. Kang and J. S. Kim, Chem. Rev., 2013, 113, 5071–5109 CrossRef CAS PubMed.
-
(a) H. Sork, J. Z. Nordin, J. J. Turunen, O. P. B. Wiklander, B. Bestas, E. M. Zaghloul, H. Margus, K. Padari, A. D. Duru, G. Corso, J. Bost, P. Vader, M. Pooga, C. I. E. Smith, M. J. A. Wood, R. M. Schiffelers, M. Hällbrink and S. E. L. Andaloussi, Mol. Ther.–Nucleic Acids, 2016, 5, e290 CrossRef CAS PubMed;
(b) H. Fang, Z. Guo, L. Lin, J. Chen, P. Sun, J. Wu, C. Xu, H. Tian and X. Chen, J. Am. Chem. Soc., 2018, 140, 11992–12000 CrossRef CAS PubMed;
(c) K. Ren, Y. Liu, J. Wu, Y. Zhang, J. Zhu, M. Yang and H. Ju, Nat. Commun., 2016, 7, 13580 CrossRef CAS PubMed;
(d) W. Wei, W. Dai, F. Yang, H. Lu, K. Zhang, Y. Xing, X. Meng, L. Zhou, Y. Zhang, Q. Yang, Y. Cheng and H. Dong, Angew. Chem., 2022, 61, e202116909 CAS.
-
(a) W. Wei, W. Cheng, W. Dai, F. Lu, Y. Cheng, T. Jiang, Z. Ren, Y. Xie, J. Xu, Q. Zhao, X. Yu, Y. Yin, J. Li and H. Dong, Nano Lett., 2022, 22, 211–219 CrossRef CAS PubMed;
(b) D. Shao, M. Li, Z. Wang, X. Zheng, Y. H. Lao, Z. Chang, F. Zhang, M. Lu, J. Yue, H. Hu, H. Yan, L. Chen, W. F. Dong and K. W. Leong, Adv. Mater., 2018, 30, e1801198 CrossRef PubMed;
(c) N. Yan, L. Lin, C. Xu, H. Tian and X. Chen, Small, 2019, 15, e1903016 CrossRef PubMed.
-
(a) K. Jiao, Q. Yan, L. Guo, Z. Qu, S. Cao, X. Chen, Q. Li, Y. Zhu, J. Li, L. Wang, C. Fan and F. Wang, Angew. Chem., 2021, 60, 14438–14445 CrossRef CAS PubMed;
(b) S. Zhu, H. Xing, P. Gordiichuk, J. Park and C. A. Mirkin, Adv. Mater., 2018, 30, 1707113 CrossRef PubMed;
(c) M. K. Vasher, G. Yamankurt and C. A. Mirkin, J. Am. Chem. Soc., 2022, 144, 3174–3181 CrossRef CAS PubMed;
(d) S. B. Ebrahimi, D. Samanta and C. A. Mirkin, J. Am. Chem. Soc., 2020, 142, 11343–11356 CrossRef CAS PubMed.
- M. E. Crosby, R. Kulshreshtha, M. Ivan and P. M. Glazer, Cancer Res., 2009, 69, 1221–1229 CrossRef CAS PubMed.
-
(a) F. Elena, R. Anassuya, M. C. Robert, G. Harriet, B. Christine, C. Meredith, D. Cecilia, B. Christopher, B. Francesca and J. L. Li, PLoS One, 2010, 5, e10345 CrossRef PubMed;
(b) D. Sabry, S. E. M. El-Deek, M. Maher, M. A. H. El-Baz, H. M. El-Bader, E. Amer, E. A. Hassan, W. Fathy and H. E. M. El-Deek, Mol. Cell. Biochem., 2019, 454, 177–189 CrossRef CAS PubMed;
(c) Z. Chen, Y. Li, H. Zhang, P. Huang and R. Luthra,
Oncogene, 2010, 29, 4362–4368 CrossRef CAS PubMed;
(d) J. Guo, Z. Yang, X. Yang, T. Li, M. Liu and H. Tang, Cancer Lett., 2018, 413, 69–81 CrossRef CAS PubMed;
(e) R. Bartoszewski, J. W. Brewer, A. Rab, D. K. Crossman, S. Bartoszewska, N. Kapoor, C. Fuller, J. F. Collawn and Z. Bebok, J. Biol. Chem., 2011, 286, 41862–41870 CrossRef CAS PubMed;
(f) S. Das, M. Kohr, B. Dunkerly-Eyring, D. I. Lee, D. Bedja, O. A. Kent, A. K. Leung, J. Henao-Mejia, R. A. Flavell and C. Steenbergen, J. Am. Heart Assoc., 2017, 6, e004694 CrossRef PubMed;
(g) W. A. Baseler, D. Thapa, R. Jagannathan, E. R. Dabkowski, T. L. Croston and J. M. Hollander, Am. J. Physiol.: Cell Physiol., 2012, 303, C1244–C1251 CrossRef CAS PubMed;
(h) S. Jiang, L.-F. Zhang, H.-W. Zhang, S. Hu, M.-H. Lu, S. Liang, B. Li, Y. Li, D. Li, E.-D. Wang and M.-F. Liu, EMBO J., 2012, 31, 1985–1998 CrossRef CAS PubMed;
(i) G. Wan, W. Xie, Z. Liu, W. Xu, Y. Lao, N. Huang, K. Cui, M. Liao, J. He and Y. Jiang, Autophagy, 2014, 10, 70–79 CrossRef CAS PubMed;
(j) N. S. Chitnis, D. Pytel, E. Bobrovnikova-Marjon, D. Pant, H. Zheng, N. L. Maas, B. Frederick, J. A. Kushner, L. A. Chodosh, C. Koumenis, S. Y. Fuchs and J. A. Diehl, Mol. Cell, 2012, 48, 353–364 CrossRef CAS PubMed;
(k) A. E. Byrd, I. V. Aragon and J. W. Brewer, J. Cell Biol., 2012, 196, 689–698 CrossRef CAS PubMed;
(l) H. Liang, J. Xiao, Z. Zhou, J. Wu, F. Ge, Z. Li, H. Zhang, J. Sun, F. Li, R. Liu and C. Chen, Oncogene, 2018, 37, 1961–1975 CrossRef CAS PubMed;
(m) P. J. Belmont, W. J. Chen, D. J. Thuerauf and C. C. Glembotski, J. Mol. Cell. Cardiol., 2012, 52, 1176–1182 CrossRef CAS PubMed.
-
(a) R. Kulshreshtha, M. Ferracin, S. E. Wojcik, R. Garzon, H. Alder, F. J. Agosto-Perez, R. Davuluri, C.-G. Liu, C. M. Croce, M. Negrini, G. A. Calin and M. Ivan, Cell. Mol. Biol., 2007, 27, 1859–1867 CrossRef CAS PubMed;
(b) Y.-M. Meng, X. Jiang, X. Zhao, Q. Meng, S. Wu, Y. Chen, X. Kong, X. Qiu, L. Su, C. Huang, M. Wang, C. Liu and P.-P. Wong, Nat. Commun., 2021, 12, 6011 CrossRef CAS PubMed;
(c) W. Li, J. Yang, L. Luo, M. Jiang, B. Qin, H. Yin, C. Zhu, X. Yuan, J. Zhang, Z. Luo, Y. Du, Q. Li, Y. Lou, Y. Qiu and J. You, Nat. Commun., 2019, 10, 3349 CrossRef PubMed.
- Y. Xing, Q. Pan, X. Du, T. Xu, Y. He and X. Zhang, ACS Appl. Mater. Interfaces, 2019, 11, 10426–10433 CrossRef CAS PubMed.
- K. Zhang, X. Meng, Z. Yang, Y. Cao, Y. Cheng, D. Wang, H. Lu, Z. Shi, H. Dong and X. Zhang, Adv. Mater., 2019, 31, e1807888 CrossRef PubMed.
- K. Zhang, Z. Yu, X. Meng, W. Zhao, Z. Shi, Z. Yang, H. Dong and X. Zhang, Adv. Sci., 2019, 6, 1900530 CrossRef PubMed.
Footnote |
† Electronic supplementary information (ESI) available: Binding specificity and stability analysis, electron microscopy observation, multi-HCR analysis details, fluorescent composition masses and probe sequences. See DOI: https://doi.org/10.1039/d3sc00563a |
|
This journal is © The Royal Society of Chemistry 2023 |