DOI:
10.1039/D3SC00401E
(Edge Article)
Chem. Sci., 2023,
14, 6095-6104
Role of supramolecular polymers in photo-actuation of spiropyran hydrogels†
Received
23rd January 2023
, Accepted 14th May 2023
First published on 16th May 2023
Abstract
Supramolecular-covalent hybrid polymers have been shown to be interesting systems to generate robotic functions in soft materials in response to external stimuli. In recent work supramolecular components were found to enhance the speed of reversible bending deformations and locomotion when exposed to light. The role of morphology in the supramolecular phases integrated into these hybrid materials remains unclear. We report here on supramolecular-covalent hybrid materials that incorporate either high-aspect-ratio peptide amphiphile (PA) ribbons and fibers, or low-aspect-ratio spherical peptide amphiphile micelles into photo-active spiropyran polymeric matrices. We found that the high-aspect-ratio morphologies not only play a significant role in providing mechanical reinforcement to the matrix but also enhance photo-actuation for both light driven volumetric contraction and expansion of spiropyran hydrogels. Molecular dynamics simulations indicate that water within the high-aspect-ratio supramolecular polymers exhibits a faster draining rate as compared to those in spherical micelles, which suggests that the high-aspect-ratio supramolecular polymers effectively facilitate the transport of trapped water molecules by functioning as channels and therefore enhancing actuation of the hybrid system. Our simulations provide a useful strategy for the design of new functional hybrid architectures and materials with the aim of accelerating response and enhancing actuation by facilitating water diffusion at the nanoscopic level.
Introduction
Many complex biological functions are enabled by highly ordered structures that are organized through both covalent and supramolecular interactions. Iconic examples include deoxyribonucleic acid (DNA), a covalent biopolymer composed of a series of nucleotides, that can form the double helical structure known as DNA duplex through non-covalent base-pairing and thus encode the genetic information of life.1,2 Another example is the sarcomere of skeletal muscle that integrates covalent titin with supramolecular polymers including actin and myosin to form the highly aligned contractile unit of a muscle fiber.3,4 Those examples in nature have recently inspired increasing interest in the development of hybrid bonding polymers in which the supramolecular structure allows dynamics and reconfiguration while the covalent polymer offers mechanical robustness.5–10 Multiple supramolecular interactions such as hydrogen bonding,11–14 host–guest interactions,15,16 electrostatic attraction,17,18 among others have been integrated with covalent bonds to create supramolecular-covalent hybrid materials19 for applications such as photocatalysis,20 tough hydrogels,21–23 tissue adhesives,24,25 recyclable polyureas,26 artificial muscles,9,27 and soft robotics.10 Importantly, supramolecular and covalent polymers can be integrated into a single material through either a simultaneous5 or sequential polymerization strategy,9,10 and interactions among both components makes it possible to precisely tune the dynamics and robustness of the hybrid material.28
An important knowledge gap in the development of supramolecular-covalent hybrid materials is the role of morphology in the supramolecular component. In living systems, there are many examples in which the shape of the supramolecular component plays a critical role in their highly dynamic and complex functions.29 For example, the fibrous network of the cytoskeleton provides mechanical support and helps cells maintain their shape and internal organization.30,31 The spherical shape of many organelles such as lysosomes helps degrade parts of cells and destroy invading viruses and bacteria.32 Synthetic supramolecular polymers33–36 alone have been demonstrated to self-assemble into structures that exhibit many different morphologies including micelles,37,38 fibers,39–42 ribbons,43–45 tubes,46–49 among others, but only very few examples have been reported in which the shapes of supramolecular components are considered when integrated with covalent systems.9,20 Peptide amphiphiles (PA) are versatile self-assembling building blocks to create designable supramolecular morphologies50 and we recently reported that fibrous PA supramolecular polymers act as a dynamic water-draining skeleton that enhances the photo-contraction performance of the hybrid materials.10 We also reported a covalent hydrogel containing photo-expanding spiropyran (SP) photo-switches that displayed a volumetric swelling (rather than contraction) upon light irradiation.51 However, hybrid materials based on this photo-expanding system have not been demonstrated. We report here on the molecular design of light-responsive hybrid hydrogels through the incorporation of supramolecular components with variable self-assembled shapes into covalent photo-expanding as well as photo-contracting systems. We systematically investigate the critical functional roles of the supramolecular assemblies through both experimental and computational studies.
Results and discussion
Design of PAs with variable supramolecular morphologies
To create a chemical connection with the covalent network, we synthesized supramolecular monomer PA 1 (Fig. 1A) that contains a polymerizable methacrylamide group at the amino terminus (N terminus; C16VVEEK-methacrylamide), which self-assembled into ribbon-shaped supramolecular polymers (Fig. S1†). The noncovalent polymerization of PA 1 and unfunctionalized PA 2 (C16VVEE-NH2) with a molar ratio of 1
:
1 in aqueous solution was found to form high-aspect-ratio twisted supramolecular ribbons. Cryogenic transmission electron microscopy (cryo-TEM, Fig. 1D) and atomic force microscopy (AFM, Fig. 1G) revealed that the co-assembled supramolecular filaments are several microns in length and tens of nanometers in diameter. Small-angle X-ray scattering (SAXS) curves have a slope of −1.3 at low q, which further indicated the formation of high-aspect-ratio filaments (Fig. 1J). We changed the order of hydrophobic valines and hydrophilic glutamic acids in PA 1 and PA 2, and synthesized PA 3 (C16VEVEK-methacrylamide) and PA 4 (C16VEVE-NH2) in order to connect to a covalent network. These supramolecular copolymers also formed high-aspect-ratio fibers (Fig. 1B, E, H and K). In order to obtain different supramolecular morphologies, we also designed PAs which replace the two hydrophobic valines with hydrophilic glutamic acids, resulting into polymerizable PA 5 (C16EEEEK-methacrylamide) and unfunctionalized PA 6 (C16EEEE-NH2). The co-assembly of PA 5 and PA 6 (Fig. 1C) with a molar ratio of 1
:
1 was found to form spheres with polydispersity in size by cryo-TEM (Fig. 1F) and atomic force microscopy (AFM) (Fig. 1I). As expected SAXS scattering scans shown in Fig. 1L reveal completely flat with a slope of 0 for q in the range 0.05–0.1 Å−1, confirming the formation of low-aspect-ratio spherical structures. Dynamic light scattering (DLS) indicated that the diameter of these micellar aggregates is approximately 50.7 nm (Fig. S2†). These results demonstrate that we could obtain different supramolecular morphologies in the co-assemblies investigated by simply changing peptide sequences.
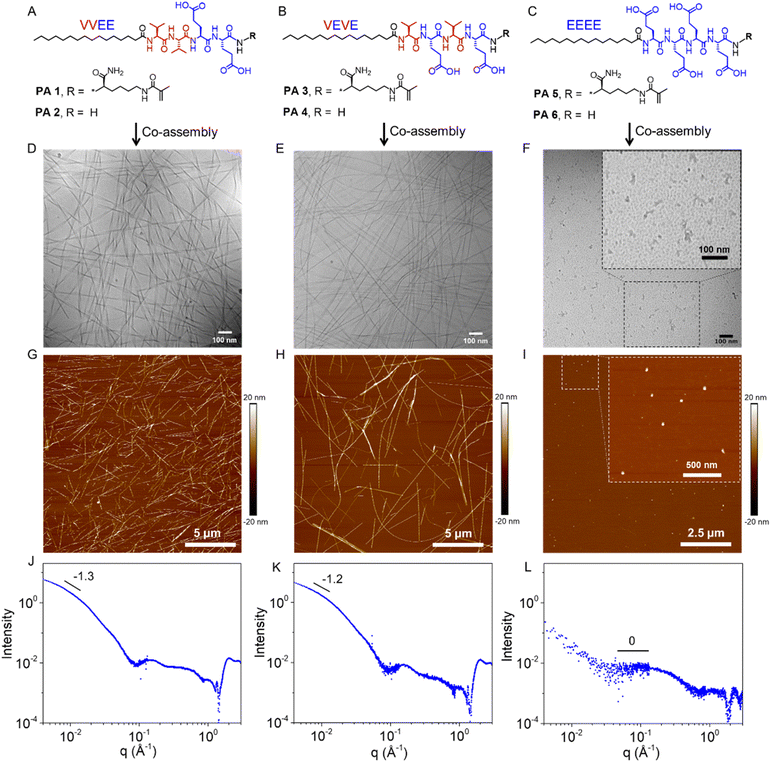 |
| Fig. 1 (A–C) Chemical structure of PAs. (D and E) A representative cryo-TEM image of high-aspect-ratio morphologies formed by co-assembly with a molar ratio of 1 : 1 of PA 1 and PA 2 (ribbons, D), PA 3 and PA 4 (fibers, E). Scale bar is 100 nm. (F) A representative cryo-TEM image of spherical micelles formed by co-assembly of PA 5 and PA 6 with a molar ratio of 1 : 1. (G and H) A representative AFM image of high-aspect-ratio morphologies formed by co-assembly with a molar ratio of 1 : 1 of PA 1 and PA 2 (G), PA 3 and PA 4 (H). (I) A representative AFM image of spherical micelles formed by co-assembly of PA 5 and PA 6 with a molar ratio of 1 : 1. (J and K) SAXS profiles of co-assembly in water with a molar ratio of 1 : 1 of PA 1 and PA 2 (J), PA 3 and PA 4 (K), PA 5 and PA 6 (L), showing the background-subtracted scattered intensity versus the scattering vector q (log–log plot). | |
Synthesis of supramolecular-covalent hybrid hydrogel
Co-assembled supramolecular nanostructures that contain polymerizable methacrylamide groups were used for free-radical copolymerization in the presence of N-isopropylacrylamide (NIPAM) and methacrylate-spiropyran monomers, N,N′-methylenebisacrylamide (MBAAm) crosslinkers, and ammonium persulfate and tetramethylethylenediamine initiators (Table S1†). The connection between supramolecular polymers and the covalent network creates a “hybrid bonding polymer” which our previous work showed is critical for mechanical reinforcement of the resulting hydrogels.6,10 Using rheological measurements, we investigated how the shape of the supramolecular nanostructure affects the mechanical properties (Fig. 2A). We found that the presence of supramolecular nanostructures with high-aspect-ratio morphologies (VVEE or VEVE) resulted in a significant mechanical reinforcement of the hybrid hydrogel and the reinforcement is tunable by adjusting the weight percentage of the high-aspect-ratio supramolecular components (Fig. 2B and C). We suggest the observed reinforcement can be explained by the covalent connection as well as physical entanglement among supramolecular high-aspect-ratio fibers or ribbons and covalent polymers. In contrast, the micellar supramolecular structures were found to provide a minimal contribution to mechanical reinforcement of the hybrid materials even though the weight percentage was as high as 2 wt% (Fig. 2D). This could be attributed to the fact that the supramolecular micelles have nearly no entanglement interactions with the covalent polymers given their spherical shape. These results demonstrate that the shape of the supramolecular nanostructures is critical for the mechanical reinforcement of these hybrid materials.
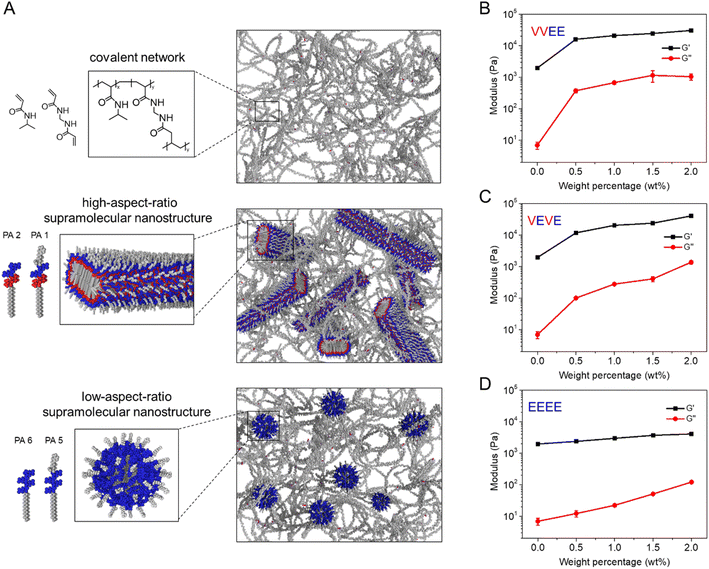 |
| Fig. 2 (A) Molecular graphics representation of supramolecular-covalent hybrid polymers formed through a free-radical polymerization process in the presence of the high-aspect-ratio (VVEE or VEVE, middle) or low-aspect-ratio spherical (EEEE, bottom) supramolecular nanostructures. The covalent network without supramolecular nanostructures is shown at the top. (B–D) Storage and loss moduli of hybrid hydrogels with variable content of total PA from 0 wt% to 2.0 wt%, with a fixed co-assembly ratio at 1 : 1 (molar ratio of methacrylate PA to unfunctionalized PA) of PA 1 and PA 2 (B), PA 3 and PA 4 (C), PA 5 and PA 6 (D). | |
Photoisomerization of supramolecular-covalent hybrid hydrogels
In addition to mechanical reinforcement, the supramolecular nanostructures were also found to play an important role in the photoisomerization of the hybrid hydrogels containing spiropyran compounds C1 and C2 (Fig. 3A and S3†). Our previous study showed that the pure covalent spiropyran hydrogels displayed a volume contraction (for C1) and expansion (for C2), respectively, upon light irradiation due to the isomerization of the protonated merocyanine (MC) to SP.51 We found that the hybrid hydrogels (VVEE, VEVE and EEEE) exhibited the same trend in terms of in volume change as do the pure covalent spiropyran hydrogels upon irradiation (Fig. 3B), indicating the presence of supramolecular components does not inhibit the observed chemically driven photo-contraction or photo-expansion. The light-induced volume change of the EEEE hybrid was found to be slightly larger than that of the VVEE or VEVE hybrids, which we attribute to the fact that the EEEE hybrid is mechanically softer (Fig. S4†) and has a relatively loose crosslinked network. This trend was also verified in the pure covalent hydrogels, where samples with a lower crosslinking density displayed a larger volume change (Fig. S5†).
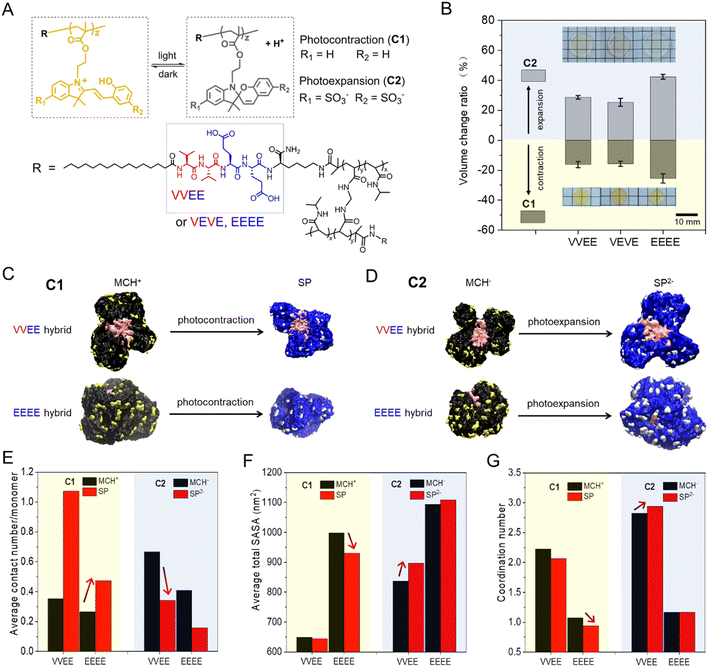 |
| Fig. 3 (A) Chemical structures of VVEE, VEVE and EEEE hybrid PNIPAM polymers containing photo-switches C1 and C2 in their spiropyran (right) and merocyanine (left) forms. (B) Plot of the net volume change of different hydrogels upon irradiation (insets show photographs of C2 expanding (top) and C1 contracting (bottom) hydrogels after irradiation). (C and D) Snapshots of final simulated structures of VVEE (top) and EEEE (bottom) hybrid polymers containing C1 (C) and C2; MCH form-PNIPAM is shown in black, SP form-PNIPAM in blue; PA assemblies in pink; MCH molecules in yellow; SP moieties in gray. (E) Average MCH or SP contacts involved within each polymer chain containing C1 (left) and C2 (right) two MCH (or SP) neighbors form a contact if their interatomic separation is shorter than 0.7 nm. (F) Average value of the solvent accessible surface area (SASA) for the total polymer systems containing C1 (left) and C2 (right). (G) Coordination number of water beads found in the first solvation shell of radial distribution functions (data in (E–G) were averaged over the last 200 ns of each simulation and arrows in (E–G) indicate value changes in the y axis). | |
In order to gain more insight into the mechanisms underlying the photo-actuation behavior, we performed a series of molecular dynamics (MD) simulations on the hybrid systems using the coarse-grained (CG) martini force field (see Fig. S6–S8† for details on the martini beads and initial conformations). Hybrid systems with supramolecular high-aspect-ratio nanostructures (VVEE hybrid; VEVE was not investigated due to its similarity to VVEE hybrid) or supramolecular spherical micelle nanostructures (EEEE hybrid) containing C1 and C2 were simulated over a time scale of approximately 2 μs (Table S2†). Control systems (pure covalent polymers without supramolecular components) with different crosslinking densities were also investigated using the same simulations. Snapshots of the final structures simulated shown in Fig. 3C, D and S9† indicated that VVEE and EEEE hybrids maintained their supramolecular high-aspect-ratio filaments and spherical micelle nanostructures, respectively, regardless of whether the spiropyran is in its SP or protonated merocyanine (MC) form. Interestingly, while maintaining their original high-aspect-ratio or spherical morphology, the internal PA nanostructures all undergo some degree of deformation, especially the PAs in the VVEE hybrid systems, which all transformed from the original cylindrical fibers to twisted fibers (see Fig. S10 and S11†). Due to the presence of these supramolecular nanostructures and their chemical connection to the covalent polymers, the pre-stretched covalent polymers collapsed around the internal PA nanostructures. We calculated the average number of spiropyran moieties interacting with each other within each polymer chain (contact number) to understand how interactions affect the photoisomerization process. As shown in Fig. 3E and S12,† the contact number increased in systems containing C1 but decreased in systems containing C2 upon irradiation. This suggests that irradiation leads to the formation of small spiropyran clusters in systems containing C1, while spiropyran units do not form clusters upon irradiation in systems containing C2, which should be a result of repulsive electrostatic interactions. We note that the average contact numbers are never much larger than 1, so clustering leading to a phase transition is probably not an important factor in the isomerization. The opposite trend between C1 and C2 systems was also observed in calculations of the solvent accessible surface area (SASA, Fig. 3F) and in values of the coordination number between the polymers and solvent particles (Fig. 3G) (see second set of simulations in Fig. S13†). These observed trends above could be explained as arising from a decrease in the net charge density of C1 upon irradiation, which results in less repulsive electrostatic interactions among SPs and consequently a higher contact number, less interaction between polymers and water, and a net photo-contraction of the hydrogel. However, the net charge density of C2 increased upon irradiation and therefore exhibited an opposite trend versusC1. These results suggest that the photoinduced decrease (or increase) of charge density accelerates the drainage of water out of (or into) the gel (Fig. S14 and S15†), which agrees with the volume changes we observed experimentally. Collectively, these results indicate that the addition of sulfonate groups in C2 can trigger an opposite photo-actuation behavior relative to C1, and most importantly, the presence of supramolecular nanostructures in the hybrid systems does not inhibit the photoisomerization of spiropyrans or the photo-actuation of SP hydrogels as we have observed in the covalent systems.51
Effect of supramolecular morphology on photo-contraction of the C1 hydrogels
To test photo-contraction performance, C1 hydrogel thin films (0.5 mm thick) were cut into a cross shape (each arm 7.0 mm long and 3.0 mm wide) and irradiated with light from the top (Fig. 4A). Because of the creation of a light-induced photoisomerization gradient through the films, the films gradually bent up towards the direction of the light source. Eventually the films flattened as prolonged irradiation eliminated the isomerization gradient (Fig. 4B). Bending angles of the different thin films were measured during this bending-flattening process and plotted in Fig. 4C. It was found that the hybrid systems containing the high-aspect-ratio supramolecular morphology (VVEE and VEVE) exhibit the fastest response to light and give a maximum bending angle of ∼70°. In contrast, hybrid materials containing the spherical micelle supramolecular morphology (EEEE) displayed a smaller bending angle (∼44°), close to that of the soft covalent polymer control material (∼33°), probably due to their low moduli. However, the stiff covalent polymer control sample with a higher crosslinking density exhibited a modulus and a maximum bending angle both close to that of the VVEE or VEVE hybrids, but bending of this highly crosslinked material was about 1.7 times slower (Fig. S16A and B†). These results indicated that mechanical enhancement by simply increasing covalent crosslinking density indeed contributes to the greater maximum bending angle of these hydrogels, but in turn limits the photoactuation rate because the denser network hinders water diffusion. The presence of high-aspect-ratio supramolecular polymer reinforces the hybrid hydrogels by physical entanglement and simultaneously maintains a relatively loose crosslinked network that is highly permeable to water, resulting in both a larger bending angle and a faster actuation rate. The results also demonstrate that the high-aspect-ratio supramolecular polymer is more effective than spherical aggregates in enhancing the mechanical properties as well as the photo-actuation performance of the hybrid system.
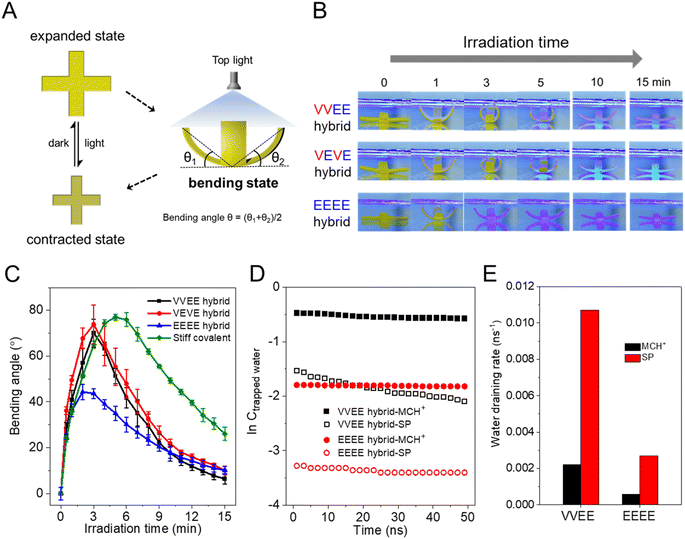 |
| Fig. 4 (A) Schematic representation of the bending and flattening process of a cross-shaped hydrogel film containing C1 when exposed by irradiation from the top; the bending angle was calculated as indicated on the right from photographs using ImageJ software. (B) Photographs of bending and flattening of different cross-shaped hydrogel films (0.5 mm thick) containing C1 irradiated continuously with blue light from the top (450 nm, 120 mW cm−2). (C) Plot of bending angles of PA hybrid films containing C1 and different supramolecular nanostructures as well as stiff covalent hydrogel with a crosslinker ratio of 10%. (D) Plot of the trapped water concentration calculated from a computer simulation as a function of time for VVEE, EEEE hybrid polymers containing either the MCH+ or SP moieties of C1 (plotted on a logarithmic scale). (E) Plot of the fitted water draining rate for VVEE and EEEE hybrid polymers containing either the MCH+ or SP moieties of C1 obtained from linear fitting of the data in (D). | |
To gain further insight into the role of different supramolecular morphologies in photo-actuation, we carried out MD simulations and monitored changes in the concentration of trapped water for different hybrid systems before and after light irradiation. These are plotted on a logarithmic scale in Fig. 4D (see Methods for details). After fitting the slopes of all the curves plotted in Fig. 4D, we determined the draining rate of the trapped water in different systems (see ESI† for details). As shown in Fig. 4E, the water draining rate for both the VVEE and EEEE hybrid systems increased upon irradiation, which can be explained by the hydrophobic nature of the ring-closed form of the C1 molecule. In comparison, the water draining rates of the SP and MCH control systems are both very small (Fig. S16C and D†), which suggests a more dynamic environment for water diffusion in the hybrid materials containing supramolecular polymers relative to samples composed only of covalent polymers. Furthermore, the presence of a high-aspect-ratio supramolecular morphology (VVEE) resulted in a much faster draining rate for trapped water in the hybrid systems for both the MCH+ and SP forms relative to the EEEE hybrid system containing spherical micelles. To show more intuitively how the trapped water can escape from the hybrid systems, we also plotted the pathways of those draining water molecules in different systems, as shown in Fig. S17 and S18.† These indicate that the high-aspect-ratio supramolecular morphology plays a critical role in enabling the trapped water to escape from the polymer network. The results suggest that the high-aspect-ratio filaments form a more continuous, percolating structure that facilitates the channeling of water molecules out the material as a more hydrophobic environment forms upon light exposure. In contrast, spherical micelles are isolated structures with poor continuity to be effective at expelling water through long range diffusion and this way impeding mechanical actuation. We also plotted the final concentration of the trapped water for different systems and found it to be on the order of ∼10−1 M (Fig. S19†), which is comparable to that reported previously for other covalent polymer systems.52 The final concentration of trapped water in the SP form is smaller than that of the MCH+ form for all the systems investigated here. This suggests that much of the water is expelled from the hydrogel upon irradiation, which is consistent with the light-induced contraction behavior observed experimentally.
Effect of supramolecular morphology on photo-expansion of the C2 hydrogels
We found that cross-shaped C2 hydrogels tend to display an asymmetric and unstable bending geometry upon irradiation from the bottom, probably due to non-uniform expansion of the gel or non-specific interactions between the gel and the glass substrate. To improve the stability and reproducibility of the expanding the C2 gels, we utilized rod-shaped hydrogel samples that hanging vertically to test the photo-expansion performance with irradiation from the left (Fig. 5A). These rod hydrogels initially bent away from the light source because of an expansion gradient across their diameter and becoming straight again with prolonged irradiation as the expansion gradient becomes smaller (Fig. 5B). The bending angles plotted in Fig. 5C revealed that the hybrid systems with a high-aspect-ratio supramolecular morphology (VVEE and VEVE) showed a larger bending angle and faster bending relative to the hybrid system with the spherical micelle supramolecular morphology (EEEE) or the fully covalent control system with low modulus. On the other hand, the stiff covalent control with a higher crosslinking density displayed a similar maximum bending angle to the VVEE and VEVE hybrids but the bending is much slower (Fig. S20†). This trend agrees with what we observed in the photo-contraction of C1 hydrogels, indicating that the supramolecular high-aspect-ratio structures play a critical role in facilitating water diffusion, regardless of whether the water is diffusing out of the material (C1 system) or being absorbed by the material (C2 system) as a result of irradiation. As previously explained, we hypothesize this is due to the water permeability of supramolecular high-aspect-ratio filaments combined with their ability to create a continuous path for water diffusion as a result of their high-aspect-ratio.
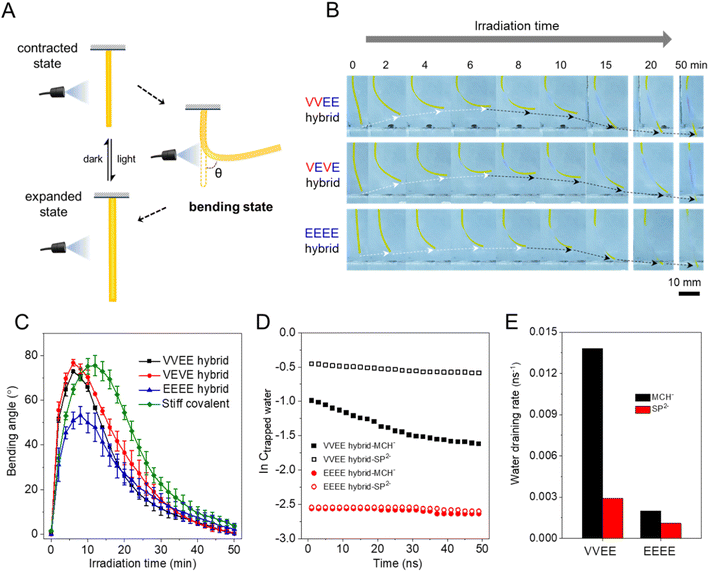 |
| Fig. 5 (A) Schematic representation of the bending-unbending process of a rod hydrogel containing C2 by irradiation from left. (B) Photographs of bending rod hydrogels containing C2 upon irradiation with continuous blue light from left (450 nm, 15 mW cm−2). (C) Plot of bending angles of PA hybrid hydrogels containing C2 with different supramolecular nanostructures as well as stiff covalent hydrogel with a crosslinker ratio of 10%. (D) Time evolution of concentration of trapped water for VVEE, EEEE hybrid polymers containing C2 obtained from simulation. (E) Fitted water draining rate for VVEE and EEEE hybrid polymers containing C2 obtained from linear fitting of data in (D). | |
To better understand the mechanisms associated with the photo-expansion and bending behaviors we observed above, we performed CG simulations and tracked the escape of water trapped in the polymer network containing C2 (Fig. 5D). The final concentration of trapped water is larger in the SP2− form than in the MCH− form for all the four systems (Fig. S21†), suggesting the occurrence of a light-induced expansion that matches well with our experimental observations. To further quantify the water diffusion difference before and after irradiation, we calculated the draining rate of trapped water in Fig. 5D, E and S22,† which indicates that all four systems display a clear decrease in the draining rate of trapped water upon irradiation (MCH− → SP2−). We interpret this decreased draining rate to be the result of the more hydrophilic nature of polymers containing the SP2− moiety relative to the MCH− moiety since the higher charge density of SP2− makes it more difficult for water to escape. Meanwhile, we observed that the presence of the high-aspect-ratio supramolecular morphology in the VVEE hybrid resulted in a larger draining rate of trapped water in both MCH− and SP2− forms relative to the micelle-containing EEEE hybrid and covalent controls (Fig. 5E and S22†). We again plotted the pathways of draining water in different systems, as shown in Fig. S23 and S24.† These observations suggest that the draining rates of water trapped in VVEE hybrid polymer are the fastest in the C2 system, highlighting the important role of the high-aspect-ratio supramolecular morphology in facilitating photo-expansion of the supramolecular-covalent hybrid polymers. Together with the previous photo-contracting C1 system, one can conclude that the supramolecular high-aspect-ratio morphology we described here facilitates water diffusion bidirectionally, that is, both into and out of the polymer network.
Conclusions
Integration of porosity and channels into hydrogels to facilitate water diffusion has been reported to improve the actuation magnitude and speed. Previous methods to create channeled hydrogels have focused on sacrificial templates and micromolding techniques, as well as 3D printing, among others.53 In this work, we have been able to incorporate supramolecular components of varying morphologies into photo-responsive spiropyran covalent networks to investigate their effect on mechanical reinforcement and photo-actuation of the hybrid materials. High-aspect-ratio supramolecular peptide amphiphile assemblies reinforced the hybrids and improved their light driven actuation relative to those containing spherical micelles. This trend was similar in both photo-contracting and photo-expanding systems. Based on our simulations, we conclude that the hydrophilic supramolecular high-aspect-ratio filaments are able to entangle with the covalent polymer network and increase the transport of water in and out of the hybrid materials, thus enhancing the mechanical properties and photoactuation rate. Further improvements on mechanical actuation might be possible with more precise variation of supramolecular morphology which may involve alignment or more complex structures of the water-transporting vasculature.
Data availability
The data that support the finding of this study are available in the main text and the ESI.†
Author contributions
C. L. and Q. X. contributed equally to this work. C. L. carried out the synthesis and experimental characterization. Q. X. performed the simulations. T. D. C. carried out the Cryo-TEM. H. S. carried out X-ray scattering. Y. Y. and M. H. S. performed AFM. A. I. helped with the simulations. C. L., L. C. P., G. C. S., and S. I. S. supervised the research.
Conflicts of interest
The authors declare no competing financial interest.
Acknowledgements
This work was supported by the Center for Bio-Inspired Energy Science (CBES), an Energy Frontier Research Center funded by the U.S. Department of Energy (DOE) Office of Basic Energy Sciences (DE-SC0000989). C. L. acknowledged the support from University of Science and Technology of China (KY2060000188, KY2060000212) and Natural Science Foundation of Anhui Province (2208085MB27). This work made use of the MatCI Facility which receives support from the MRSEC Program (NSF DMR-1720139) of the Materials Research Center at Northwestern University, the IMSERC at Northwestern University, which has received support from the NIH (1S10OD012016-01/1S10RR019071-01A1), Soft and Hybrid Nanotechnology Experimental (SHyNE) Resource (NSF ECCS-1542205), the State of Illinois, and the International Institute for Nanotechnology (IIN). This work also made use of the BioCryo facility of Northwestern University's NUANCE Center, which has received support from the Soft and Hybrid Nanotechnology Experimental (SHyNE) Resource (NSF ECCS-1542205); the MRSEC program (NSF DMR-1720139) at the Materials Research Center; the International Institute for Nanotechnology (IIN); and the State of Illinois, through the IIN. The X-ray scattering experiment was performed at the DuPont-Northwestern-Dow Collaborative Access Team (DND-CAT) located at Sector 5 of the Advanced Photon Source (APS). DND-CAT is supported by Northwestern University, The Dow Chemical Company, and DuPont de Nemours, Inc., and APS is a U.S. Department of Energy (DOE) Office of Science User Facility operated for the DOE Office of Science by Argonne National Laboratory under Contract No. DE-AC02-06CH11357. The authors acknowledge Mark Seniw for creating schematic illustrations.
References
-
R. R. Sinden, C. E. Pearson, V. N. Potaman and D. W. Ussery, DNA: structure and function, in Advances in Genome Biology, ed. R. S. Verma, JAI, 1998, vol. 5, pp. 1–141 Search PubMed.
- J. D. Watson and F. H. C. Crick, Molecular structure of nucleic acids – a structure for deoxyribose nucleic acid, Nature, 1953, 171(4356), 737–738 CrossRef CAS PubMed.
-
M. A. Geeves and K. C. Holmes, The molecular mechanism of muscle contraction, in Advances in Protein Chemistry, Academic Press, 2005, vol. 71, pp. 161–193 Search PubMed.
- E. Behrmann, M. Muller, P. A. Penczek, H. G. Mannherz, D. J. Manstein and S. Raunser, Structure of the rigor actin-tropomyosin-myosin complex, Cell, 2012, 150(2), 327–338 CrossRef CAS PubMed.
- Z. Yu, F. Tantakitti, T. Yu, L. C. Palmer, G. C. Schatz and S. I. Stupp, Simultaneous covalent and noncovalent hybrid polymerizations, Science, 2016, 351(6272), 497–502 CrossRef CAS PubMed.
- S. I. Stupp, T. D. Clemons, J. K. Carrow, H. Sai and L. C. Palmer, Supramolecular and hybrid bonding polymers, ISR J. Chem., 2020, 60, 124–131 CrossRef CAS.
- X. S. Hou, C. F. Ke, Y. Zhou, Z. Xie, A. Alngadh, D. T. Keane, M. S. Nassar, Y. Y. Botros, C. A. Mirkin and J. F. Stoddart, Concurrent covalent and supramolecular polymerization, Chem.–Eur. J., 2016, 22(35), 12301–12306 CrossRef CAS PubMed.
- E. P. Bruckner, T. Curk, L. Dordevic, Z. W. Wang, Y. Yang, R. M. Qiu, A. J. Dannehoffer, H. Sai, J. Kupferberg, L. C. Palmer, E. Lujiten and S. I. Stupp, Hybrid nanocrystals of small molecules and chemically disordered polymers, ACS Nano, 2022, 16(6), 8993–9003 CrossRef CAS PubMed.
- S. M. Chin, C. V. Synatschke, S. P. Liu, R. J. Nap, N. A. Sather, Q. F. Wang, Z. Alvarez, A. N. Edelbrock, T. Fyrner, L. C. Palmer, I. Szleifer, M. O. Olvera de la Cruz and S. I. Stupp, Covalent-supramolecular hybrid polymers as muscle-inspired anisotropic actuators, Nat. Commun., 2018, 9(1), 2395 CrossRef PubMed.
- C. Li, A. Iscen, H. Sai, K. Sato, N. A. Sather, S. M. Chin, Z. Alvarez, L. C. Palmer, G. C. Schatz and S. I. Stupp, Supramolecular-covalent hybrid polymers for light-activated mechanical actuation, Nat. Mater., 2020, 19(8), 900–909 CrossRef CAS PubMed.
- M. Y. Guo, L. M. Pitet, H. M. Wyss, M. Vos, P. Y. W. Dankers and E. W. Meijer, Tough stimuli-responsive supramolecular hydrogels with hydrogen-bonding network junctions, J. Am. Chem. Soc., 2014, 136(19), 6969–6977 CrossRef CAS PubMed.
- K. M. Hutchins, Functional materials based on molecules with hydrogen-bonding ability: applications to drug co-crystals and polymer complexes, R. Soc. Open Sci., 2018, 5(6), 180564 CrossRef PubMed.
- J. A. Neal, D. Mozhdehi and Z. B. Guan, Enhancing mechanical performance of a covalent self-healing material by sacrificial noncovalent bonds, J. Am. Chem. Soc., 2015, 137(14), 4846–4850 CrossRef CAS PubMed.
- Y. Yanagisawa, Y. L. Nan, K. Okuro and T. Aida, Mechanically robust, readily repairable polymers via tailored noncovalent cross-linking, Science, 2018, 359(6371), 72–76 CrossRef CAS PubMed.
- H. Yang, B. Yuan, X. Zhang and O. A. Scherman, Supramolecular chemistry at interfaces: host-guest interactions for fabricating multifunctional biointerfaces, Acc. Chem. Res., 2014, 47(7), 2106–2115 CrossRef CAS PubMed.
- J. Teyssandier, S. De Feyter and K. S. Mali, Host-guest chemistry in two-dimensional supramolecular networks, Chem. Commun., 2016, 52(77), 11465–11487 RSC.
- S. C. Huang, X. X. Xia, R. X. Fan and Z. G. Qan, Programmable electrostatic interactions expand the landscape of dynamic functional hydrogels, Chem. Mater., 2020, 32(5), 1937–1945 CrossRef CAS.
- R. M. Capito, H. S. Azevedo, Y. S. Velichko, A. Mata and S. I. Stupp, Self-assembly of large and small molecules into hierarchically ordered sacs and membranes, Science, 2008, 319(5871), 1812–1816 CrossRef CAS PubMed.
- Z. M. Zhang, L. Cheng, J. Zhao, L. Wang, K. Liu, W. Yu and X. Z. Yan, Synergistic covalent and supramolecular polymers for mechanically robust but dynamic materials, Angew. Chem., Int. Ed., 2020, 59(29), 12139–12146 CrossRef CAS PubMed.
- H. Sai, A. Erbas, A. Dannenhoffer, D. X. Huang, A. Weingarten, E. Siismets, K. Jang, K. R. Qu, L. C. Palmer, M. O. Olvera de la Cruz and S. I. Stupp, Chromophore amphiphile-polyelectrolyte hybrid hydrogels for photocatalytic hydrogen production, J. Mater. Chem. A, 2020, 8(1), 158–168 RSC.
- J. Y. Sun, X. H. Zhao, W. R. K. Illeperuma, O. Chaudhuri, K. H. Oh, D. J. Mooney, J. J. Vlassak and Z. G. Suo, Highly stretchable and tough hydrogels, Nature, 2012, 489(7414), 133–136 CrossRef CAS PubMed.
- C. B. Rodell, N. N. Dusaj, C. B. Highley and J. A. Burdick, Injectable and cytocompatible tough double-network hydrogels through tandem supramolecular and covalent crosslinking, Adv. Mater., 2016, 28(38), 8419–8424 CrossRef CAS PubMed.
- T. Y. Cao, H. Y. Jia, Y. C. Dong, S. B. Gui and D. S. Liu, In situ formation of covalent second network in a DNA supramolecular hydrogel and its application for 3D cell imaging, ACS Appl. Mater. Interfaces, 2020, 12(4), 4185–4192 CrossRef CAS PubMed.
- Y. C. Wang, D. Liang, Z. G. Suo and K. Jia, Synergy of noncovalent interlink and covalent toughener for tough hydrogel adhesion, Extreme Mech. Lett., 2020, 39, 100797 CrossRef.
- H. Yuk, C. E. Varela, C. S. Nabzdyk, X. Y. Mao, R. F. Padera, E. T. Roche and X. H. Zhao, Dry double-sided tape for adhesion of wet tissues and devices, Nature, 2019, 575(7781), 169–174 CrossRef CAS PubMed.
- B. Qin, S. Zhang, P. Sun, B. H. Tang, Z. H. Yin, X. Cao, Q. Chen, J. F. Xu and X. Zhang, Tough and multi-recyclable cross-linked supramolecular polyureas via incorporating noncovalent bonds into main-chains, Adv. Mater., 2020, 32(36), 2000096 CrossRef CAS PubMed.
- Z. M. Zhang, L. Cheng, J. Zhao, H. Zhang, X. Y. Zhao, Y. H. Liu, R. X. Bai, H. Pan, W. Yu and X. Z. Yan, Muscle-mimetic synergistic covalent and supramolecular polymers: phototriggered formation leads to mechanical performance boost, J. Am. Chem. Soc., 2021, 143(2), 902–911 CrossRef CAS PubMed.
- T. D. Clemons and S. I. Stupp, Design of materials with supramolecular polymers, Prog. Polym. Sci., 2020, 111, 101310 CrossRef CAS PubMed.
- G. K. Voeltz and W. A. Prinz, Sheets, ribbons and tubules – how organelles get their shape, Nat. Rev. Mol. Cell Biol., 2007, 8(3), 258–264 CrossRef CAS PubMed.
- B. Wickstead and K. Gull, The evolution of the cytoskeleton, J. Cell Biol., 2011, 194(4), 513–525 CrossRef CAS PubMed.
- R. H. Pritchard, Y. Y. S. Huang and E. M. Terentjev, Mechanics of biological networks: from the cell cytoskeleton to connective tissue, Soft Matter, 2014, 10(12), 1864–1884 RSC.
- S. O. Lie, B. H. Schofield, H. A. Taylor Jr and S. B. Doty, Structure and function of the lysosomes of human fibroblasts in culture: dependence on medium pH, Pediatr. Res., 1973, 7(1), 13–19 CrossRef CAS PubMed.
- L. Brunsveld, B. J. Folmer, E. W. Meijer and R. P. Sijbesma, Supramolecular polymers, Chem. Rev., 2001, 101(12), 4071–4098 CrossRef CAS PubMed.
- T. Aida, E. W. Meijer and S. I. Stupp, Functional supramolecular polymers, Science, 2012, 335(6070), 813–817 CrossRef CAS PubMed.
- B. Qin, Z. H. Yin, X. Y. Tang, S. Zhang, Y. H. Wu, J. F. Xu and X. Zhang, Supramolecular polymer chemistry: from structural control to functional assembly, Prog. Polym. Sci., 2020, 100, 101167 CrossRef CAS.
- T. Aida and E. W. Meijer, Supramolecular polymers – we've come full circle, ISR J. Chem., 2020, 60, 33–47 CrossRef CAS.
- X. F. Ji, J. Y. Li, J. Z. Chen, X. D. Chi, K. L. Zhu, X. Z. Yan, M. M. Zhang and F. H. Huang, Supramolecular micelles constructed by crown ether-based molecular recognition, Macromolecules, 2012, 45(16), 6457–6463 CrossRef CAS.
- W. Z. Yuan, H. Zou, W. Guo, T. X. Shen and J. Ren, Supramolecular micelles with dual temperature and redox responses for multi-controlled drug release, Polym. Chem., 2013, 4(9), 2658–2661 RSC.
- J. D. Hartgerink, E. Beniash and S. I. Stupp, Self-assembly and mineralization of peptide-amphiphile nanofibers, Science, 2001, 294(5547), 1684–1688 CrossRef CAS PubMed.
- M. L. Ma, Y. Kuang, Y. Gao, Y. Zhang, P. Gao and B. Xu, Aromatic-romatic interactions induce the self-assembly of pentapeptidic derivatives in water to form nanofibers and supramolecular hydrogels, J. Am. Chem. Soc., 2010, 132(8), 2719–2728 CrossRef CAS PubMed.
- C. B. Cooper, J. H. O. Kang, Y. K. Yin, Z. A. Yu, H. C. Wu, S. Nikzad, Y. Ochiai, H. P. Yan, W. Cai and Z. N. Bao, Multivalent assembly of flexible polymer chains into supramolecular nanofibers, J. Am. Chem. Soc., 2020, 142(39), 16814–16824 CrossRef CAS PubMed.
- S. Debnath, S. Roy and R. V. Ulijn, Peptide nanofibers with dynamic instability through nonequilibrium biocatalytic assembly, J. Am. Chem. Soc., 2013, 135(45), 16789–16792 CrossRef CAS PubMed.
- Y. Vyborna, M. Vybornyi and R. Haner, From Ribbons to networks: hierarchical organization of DNA-grafted supramolecular polymers, J. Am. Chem. Soc., 2015, 137(44), 14051–14054 CrossRef CAS PubMed.
- F. Garcia, J. Buendia and L. Sanchez, Supramolecular ribbons from amphiphilic trisamides self-assembly, J. Org. Chem., 2011, 76(15), 6271–6276 CrossRef CAS PubMed.
- E. T. Pashuck and S. I. Stupp, Direct observation of morphological transformation from twisted ribbons into helical ribbons, J. Am. Chem. Soc., 2010, 132(26), 8819–8821 CrossRef CAS PubMed.
- X. Y. Gao and H. Matsui, Peptide-based nanotubes and their applications in bionanotechnology, Adv. Mater., 2005, 17(17), 2037–2050 CrossRef CAS PubMed.
- T. Shimizu, M. Masuda and H. Minamikawa, Supramolecular nanotube architectures based on amphiphilic molecules, Chem. Rev., 2005, 105(4), 1401–1443 CrossRef CAS PubMed.
- T. Sendai, S. Biswas and T. Aida, Photoreconfigurable supramolecular nanotube, J. Am. Chem. Soc., 2013, 135(31), 11509–11512 CrossRef CAS PubMed.
- J. Y. Rho, H. Cox, E. D. H. Mansfield, S. H. Ellacott, R. Peltier, J. C. Brendel, M. Hartlieb, T. A. Waigh and S. Perrier, Dual self-assembly of supramolecular peptide nanotubes to provide stabilisation in water, Nat. Commun., 2019, 10, 4708 CrossRef PubMed.
- M. P. Hendricks, K. Sato, L. C. Palmer and S. I. Stupp, Supramolecular assembly of peptide amphiphiles, Acc. Chem. Res., 2017, 50(10), 2440–2448 CrossRef CAS PubMed.
- C. Li, A. Iscen, L. C. Palmer, G. C. Schatz and S. I. Stupp, Light-driven expansion of spiropyran hydrogels, J. Am. Chem. Soc., 2020, 142(18), 8447–8453 CrossRef CAS PubMed.
- M. K. T. de Brito, W. R. dos Santos, B. R. D. Correia, R. A. de Queiroz, F. V. D. Tavares, G. L. D. Neto and A. G. B. de Lima, Moisture absorption in polymer composites reinforced with vegetable fiber: a three-dimensional investigation via langmuir model, Polymers, 2019, 11(11), 1847 CrossRef PubMed.
- R. Xie, W. Zheng, L. Guan, Y. Ai and Q. Liang, Engineering of hydrogel materials with perfusable microchannels for building vascularized tissues, Small, 2020, 16(15), 1902838 CrossRef CAS PubMed.
Footnotes |
† Electronic supplementary information (ESI) available: Experimental procedures, compound characterization and coarse-grained simulations including Supporting Fig. S1–S24, Supporting Tables S1 and S2. See DOI: https://doi.org/10.1039/d3sc00401e |
‡ These authors contributed equally to this work. |
§ Present address: Max Planck Institute for Polymer Research, Ackermannweg 10, Mainz 55128, Germany. |
|
This journal is © The Royal Society of Chemistry 2023 |
Click here to see how this site uses Cookies. View our privacy policy here.