DOI:
10.1039/D3SC00127J
(Edge Article)
Chem. Sci., 2023,
14, 4351-4356
Copper-catalyzed asymmetric allylic alkylation of racemic inert cyclic allylic ethers under batch and flow conditions†
Received
9th January 2023
, Accepted 22nd March 2023
First published on 22nd March 2023
Abstract
Highly enantioselective Cu-catalyzed asymmetric allylic alkylation of racemic inert cyclic allylic ethers is accomplished in this work. The use of Grignard reagents in combination with BF3·OEt2 and CuBr·SMe2/L2 is the key to enable employment of long-challenging low reactive allylic substrates in this AAA reaction. In addition, the approach exhibited a remarkable superiority in the construction of a stereogenic center involving challenging sterically hindered nucleophiles for both primary and secondary alkyl groups. Extension of this method under continuous flow conditions was also performed with excellent enantioselectivity in a short residence time. Mechanistic experiments indicated that the reaction proceeded through an in situ generated allylic bromide intermediate. All these advantages demonstrated that this chiral catalytic system is a valuable complement for existing asymmetric catalytic methods.
Introduction
Asymmetric allylic alkylation (AAA) reactions are powerful synthetic tools to strategically install C–C bonds.1–4 Generally, there are two main strategies for generating asymmetric C–C bonds in AAA reactions: (1) introduction of a chiral center from a prochiral substrate through the SN2′ pathway5–15 (Scheme 1a); (2) resolution,16,17 dynamic kinetic processes18–22 (DyKAT), or direct enantioconvergent transformation23–27 (DECT) from racemic chiral starting materials. Compared to prochiral substrates, AAA reactions from racemic substrates were far less studied. There are two equal amounts of enantiomers in the starting materials which complicated the catalytic processes and made the reaction more challenging.21 Even though the use of stabilized nucleophiles (pKa < 25) in Pd-catalyzed AAA of racemic starting materials has been particularly successful since 1994,22,28 the use of non-stabilized nucleophiles (pKa > 50) in Cu-catalyzed AAA of racemic starting materials with high yields and enantioselectivities was not reported until 2009.24 Many pioneers have made significant contributions to the development of this chemistry since then. For example, different copper–ligand complexes were synthesized,20,23 and various non-stabilized nucleophiles such as Grignard reagents,24,29 organolithium reagents,26 and organozirconium reagents17,30 were developed; versatile allylic substrates, such as bromides,20,23,24 chlorides,17,18,29 acetates25 and phosphates19 were employed (Scheme 1b). However, there still remain long-challenging issues in this field. First, Cu-catalyzed AAA reactions normally use allylic substrates with good leaving groups (such as Br, Cl, OCOR etc.) to enhance the reactivity and enantioselectivity.24 However, these substrates are mostly sensitive, prone to deterioration, and hard to purify and store, which limits their applications in multistep reactions and synthesis.8,31 Meanwhile, racemic inert substrates such as allylic ethers31–34 have shown unsatisfactory performance in AAA reactions. The pioneering research reported by Consiglio and co-workers revealed moderate enantioselectivities in Ni-catalyzed AAA using phenyl-ethers.35 Later on, Okamoto and co-workers achieved moderate results by employing pyridyl-ethers in Cu-catalyzed AAA with Grignard reagents.36 In 2012, Feringa and co-workers developed a highly enantioselective Cu-catalyzed AAA reaction with prochiral linear allylic ethers using organolithium reagents.8 However, the nucleophile scope was limited to nBuLi and nHexLi in this beautiful chemistry. To the best of our knowledge, despite extensive endeavours, highly efficient Cu-catalyzed AAA reactions with racemic inert substrates have not yet emerged.
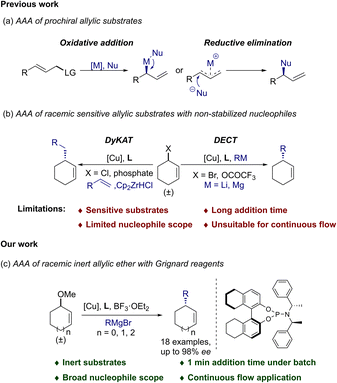 |
| Scheme 1 Asymmetric allylic alkylation (AAA) procedures. | |
Another key challenge in Cu-catalyzed AAA reactions is the generality of the non-stabilized nucleophile scope used in the catalytic systems. Employment of functionalized and sterically hindered nucleophiles was found to be extremely difficult during the process.24 Crucial pioneering work was first conducted by Alexakis and co-workers where sterically hindered secondary alkyl groups were accomplished in moderate enantioselectivities with racemic allylic bromides.23 Later on, the improvement of the performance of secondary alkyl metal reagents was achieved with the same group by a well-designed catalytic system using racemic allylic trifluoroacetates, though it was not compatible with low reactive simple primary alkyl groups (such as MeMgBr).25 At the same time, Fletcher and co-workers described the breakthrough of a Cu-catalyzed AAA of racemic allylic chlorides with organozirconium reagents. The reaction was able to tolerate a variety of functionalized primary alkyl groups under convenient mild conditions.18,19 To the best of our knowledge, despite all these excellent advances, a general universal catalytic system which could enable efficient Cu-catalyzed AAA with challenging sterically hindered secondary and simple primary alkyl nucleophiles has not emerged. Herein, we reported a highly efficient Cu-catalyzed AAA reaction using racemic inert cyclic allylic ethers in combination with BF3·OEt2 and CuBr·SMe2/L2. Noteworthily, this catalytic system delivered general efficiency for challenging sterically hindered Grignard reagents with both primary and secondary alkyl groups.
Results and discussion
We started our investigation by examining the AAA reactions of 3-benzyloxycyclohexene (1a) with 1.5 equivalents of MeLi as a nucleophile in the presence of BF3·OEt2 (2 eq.), catalyzed by CuBr·SMe2 (5 mol%) and phosphoramidites (S,S,S)-L2 (11 mol%) in DCM. The reaction gave 3-methylcyclohexene (3a) in 70% yield with 48% ee (Table 1, entry 1). To enhance the enantioselectivity of 3a, we screened different copper sources, ligands and Lewis acids. However, employment of copper sources (such as CuCl, CuTc and Cu(OTf)2), ligands (such as (S,S,S)-L1 and (S,S,R)-L3), and Lewis acids (BF2OTf generated in situ from BF3·OEt2 and TMSOTf) gave poor results (Table 1, entries 1–7). Encouragingly, the enantioselectivity of 3a improved to 87% ee by using less reactive MeMgBr (Table 1, entry 8). Meanwhile, the copper/ligand ratio was found to have little effect on this catalytic system (Table 1, entries 8 vs. 9). When diluted MeMgBr (0.5 M in Et2O) was used, the enantioselectivity increased further to 96% ee in 46% yield (Table 1, entry 10). Delightfully, the yield of 3a was also optimized to 85% maintaining the high enantioselectivity by adjusting –OBn to the less hindered –OMe group (Table 1, entry 11). Benefiting from this low activity of the ether substrate, MeMgBr could be added dropwise for only 1 min instead of the 0.5 h or 2 h reported in previous work8,25 (Table 1, entries 11 vs. 12). Finally, single isomer 3a was produced in excellent yield and enantioselectivity with 10 mol% catalyst loading (99% yield, 98% ee, Table 1, entry 13). It was worth noting that the enantioselectivity of the product (Table S2,† entry 3, 94% ee) remained excellent even at a low catalyst loading (1 mol%), but the conversion rate of 3-methoxycyclohex-1-ene (1b) showed a significant decline at 1 mol% catalyst loading (Table S2,† entry 3, 78% conversion rate), despite extending the reaction time to 4 hours.
Table 1 Selected optimization experimentsa
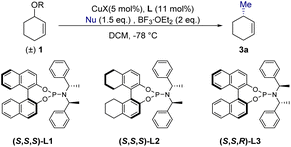
|
Entry |
Nu |
R |
CuX |
L |
Yieldd |
eei |
Conditions: racemic 1 (0.4 mmol), CuX (5% mol), L (11% mol), BF3·OEt2 (2 eq.), CH2Cl2 (4 mL), −78 °C, MeLi (1.5 eq.), 2 h addition time.
Conditions: racemic 1 (0.4 mmol), CuX (5% mol), L (6% mol), BF3·OEt2 (1.5 eq.), CH2Cl2 (4 mL), −78 °C, MeMgBr (3 M in Et2O, 1.5 eq.), 1 min addition time.
2 eq. BF3·OEt2 and 6 eq. TMSOTf were used.
Yield was determined by GC.
2 h addition time.
CuX (5% mol) and L (11% mol) were used.
CuX (10% mol) and L (11% mol) were used.
MeMgBr (0.5 M in Et2O) was used.
ee was determined by chiral GC.
|
1 |
MeLi |
Bn |
CuBr·SMe2 |
L2
|
70% |
48% |
2 |
MeLi |
Bn |
CuCl |
L2
|
57% |
24% |
3 |
MeLi |
Bn |
CuTc |
L2
|
61% |
37% |
4 |
MeLi |
Bn |
CuBr·SMe2 |
L1
|
74% |
<10% |
5 |
MeLi |
Bn |
CuBr·SMe2 |
L3
|
54% |
15% |
6 |
MeLi |
Bn |
Cu(OTf)2 |
L3
|
N.D. |
— |
7c |
MeLi |
Bn |
CuBr·SMe2 |
L2
|
N.D. |
— |
8b,f |
MeMgBr |
Bn |
CuBr·SMe2 |
L2
|
48% |
87% |
9b |
MeMgBr |
Bn |
CuBr·SMe2 |
L2
|
45% |
87% |
10b |
MeMgBre,h |
Bn |
CuBr·SMe2 |
L2
|
46% |
96% |
11b |
MeMgBre,h |
Me |
CuBr·SMe2 |
L2
|
85% |
96% |
12b |
MeMgBrh |
Me |
CuBr·SMe2 |
L2
|
88% |
96% |
13b,g |
MeMgBrh |
Me |
CuBr·SMe2 |
L2
|
99% |
98% |
With the optimal conditions in hand, the nucleophile scope of this novel protocol was then evaluated. Several enantiomerically enriched cycloalkenes were obtained with excellent enantioselectivities by using simple unfunctionalized primary alkyl (3a–3f, 4a), alkenyl (3g) and aromatic Grignard reagents (3i–3k, 5a). Surprisingly, bulky alkyl groups such as secondary alkyl Grignard reagents also reacted smoothly to give the corresponding products 3l–3p in 83–93% yields with excellent enantioselectivities. In particular, decent improvements were achieved for previously challenging sterically hindered nucleophiles in AAA of the reported allylic bromide approaches,23 such as 3h (65% ee vs. 28% ee), 3l (82% ee vs. 40% ee), 3m (90% ee vs. 74% ee), and 3n (88% ee vs. 70% ee) and for different ring sizes 4a (91% ee vs. 60% ee) and 5a (83% ee vs. 44% ee). To further extend the generality of this catalytic system, the reaction conditions were applied to challenging acyclic ether. However, only 15% ee was obtained in the reaction (Scheme S1†), which was similar to the previous result with acyclic bromide (8% ee).23 Preliminary experiments of AAA reactions using challenging 3-methoxy-1-methylcyclohex-1-ene to form a more complex quaternary cycloalkene were also performed. A mixture of 1,1- and 1,3-disubstituted alkylation products was obtained with moderate enantioselectivities (Scheme S2,† 35% yield, 83% ee for 1,1-disubstituted quaternary cycloalkene and 12% yield, 53% ee for 1,3-disubstituted cycloalkene). Further research focused on the construction of a stereogenic quaternary center is currently under study and will be reported in due course.
Although satisfactory results were achieved under batch conditions, an intractable question worth thinking about is whether Grignard reagents are too reactive to scale up.37–39 The recent emerging continuous flow technology demonstrated many advantages, including higher safety and efficiency, accurate control, better heat and mass transfer, easier amplification and better sustainability.40–43 Thus, we also explored the application of this protocol under continuous flow conditions. However, the enantioselectivities of the products dropped significantly under continuous flow conditions when using allylic bromide as the substrate in previous reports (up to 33% ee).26 Generally, the organometallic reagents were added dropwise over 2 h (ref. 26) or 0.5 h (ref. 25) to a solution of substrates to avoid side-reactions under batch conditions. In contrast, substrates and organometallic reagents are mixed simultaneously under flow conditions; the highly active allylic bromides may undergo uncatalyzed reactions6 or react with “ate” and “aggregated” copper species25 generated by a chiral catalyst exposed to an excess of organometallic reagents during the process. We suspected that the difference in contact mode between batch and flow mainly resulted in a decrease in enantioselectivities. Thus, we started our investigation by employing inert allylic ethers instead of high reactive allylic substrates here. The flow system was set up as shown in Scheme 3. Solutions of CuBr·SMe2, ligand L2 and cyclic racemic substrate 1b were first mixed with a BF3·OEt2 stream, and then, another stream of phenylethyl Grignard reagent was pumped into a microchip reactor. A sonication device was employed to increase reaction mixing and prevent solid aggregation. Encouragingly, the desired products were obtained in high yield with excellent enantioselectivity within 3.6 s under optimized flow conditions (Table S1 and Scheme S3†). And the system could continue operating for over 700 min without any loss of activity or enantioselectivity to provide 1.09 g product (84% yield, 91% ee).
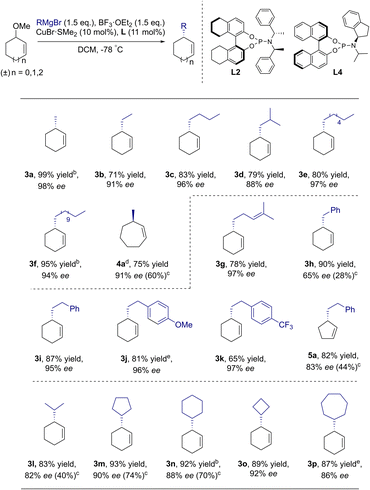 |
| Scheme 2 Substrate scope. aConditions: racemic 1 (0.4 mmol), CuBr·SMe2 (10% mol), L2 (11% mol), BF3·OEt2 (1.5 eq.), CH2Cl2 (4 mL), −78 °C, MeMgBr (1.5 eq.). ee was determined by chiral GC. bYield was determined by GC or NMR. cThe highest ee reported for the alkylation of analogous allylic bromides is given in parenthesis. dL4 was used. eIsolated yield of the corresponding diastereoisomeric epoxides. | |
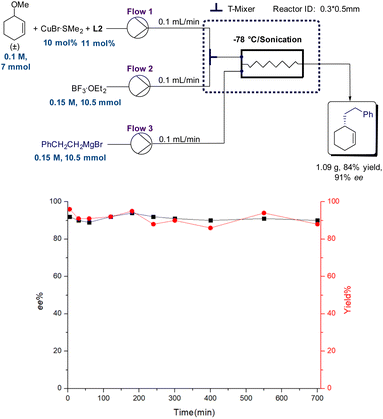 |
| Scheme 3 Cu-catalyzed AAA reaction of 1b under continuous flow conditions. | |
We then paid our attention to the mechanism of this new Cu-catalyzed AAA protocol. A Lewis acid was confirmed to be the key for the formation of desired products from racemic inert cyclic allylic ethers here.44 We carried out the AAA reaction of 1b without BF3·OEt2, and the starting material 1b could be almost completely recovered (Scheme 4 – (1)). It suggested that ether groups could act as a robust protecting group for former stages of synthetic schemes in the absence of a Lewis acid. Conversely, the ether groups could become leaving groups to perform enantioselective C–C bond formation in the presence of a Lewis acid and copper catalyst. This provided new opportunities for the application of the Cu-catalyzed AAA protocol using racemic inert cyclic allylic ethers in multistep reactions and synthesis.31 In addition, we examined the AAA reaction of 1b using cyclohexylmagnesium chloride as a nucleophile instead of cyclohexylmagnesium bromide (88% ee, Scheme 2, 3n). Surprisingly, the enantioselectivity of product 3n using cyclohexylmagnesium chloride was very low (Scheme 4 – (2), 33% ee). This phenomenon indicated that halogen anions from Grignard reagents might be involved in the reaction and influence the enantioselectivity of the product. A similar phenomenon was also observed in Fletcher and co-workers's work.18,19 They considered that allyl halide formed in situ from a halogen anion and phosphate in the reaction and reacted with nucleophiles to prove enantio-enriched alkylation products. We suspected that the allylic ethers might undergo analogical transformation here. To gain more insight into the reaction mechanism, MgBr2, instead of Grignard reagents was added to the mixture of 1b and BF3·OEt2. As we expected, 45% cyclic allylic bromide (1c) was observed in 1 h (Fig. S3†), indicating that 1c could be generated in situ from 1b in this catalytic system (Scheme 4 – (2) and (3)). Furthermore, since we were not able to observe the intermediate 1c in the reaction of racemic allylic ethers, an even lower reactive 1a substrate was employed in our experiments. Inspiringly, trace 1c was observed during our standard AAA reaction (Scheme 4 – (4), Fig. S1 and S2†). In combination with the inspiration from Alexakis's and co-workers pioneering work for DECT progress,25 we proposed a plausible mechanistic route for this new Cu-catalyzed AAA protocol using racemic inert cyclic allylic ethers. First, racemic 1c is generated from 1b in the presence BF3·OEt2 and Br−. Then, (R)-1c and (S)-1c underwent a DECT process to form the same intermediate Int-1. Finally, Int-1c underwent reductive elimination to provide the desired single product 3 in high yield and enantioselectivity (Scheme 4 – (5)).
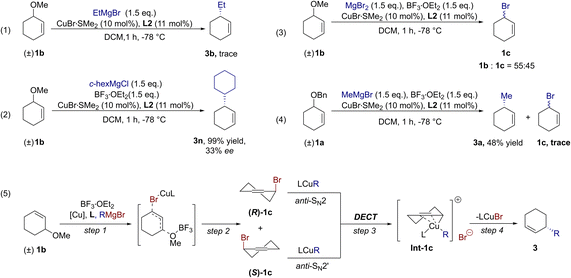 |
| Scheme 4 Mechanistic analysis and a plausible reaction mechanism. | |
Conclusions
In summary, a highly efficient Cu-catalyzed AAA reaction of racemic inert cyclic allylic ethers was developed here. The use of BF3·OEt2 with CuBr·SMe2/L2 was confirmed to be crucial in this catalytic system through the proposed allylic bromide intermediate. Meanwhile, the new method showed significant advantages in nucleophile scope over existing protocols with racemic sensitive allylic substrates. In addition, application of the method under continuous flow conditions was also conducted successfully with excellent enantioselectivity and yield in a short residence time. We anticipate that this research will aid the development of a new AAA reaction with inert substrates and provide a valuable tool for existing asymmetric catalytic methods. Intensive efforts are now directed toward the extension of this process to other inert allylic substrates in our lab.
Data availability
The ESI† is available and contains experimental and analytical data.
Author contributions
The manuscript was written through contributions of all authors. All authors have given approval to the final version of the manuscript.
Conflicts of interest
There are no conflicts to declare.
Acknowledgements
This work was supported by the Shenzhen Science and Technology Research Fund No. JCYJ20190806142203709; JSGG20191129114029286; JSGG20201103153807021; GXWD20220811173736002. We are also grateful to the Guangdong Basic and Applied Basic Research Foundation (No. 2021A1515110366) and Shenzhen Key Laboratory of Advanced Functional Carbon Materials Research and Comprehensive Application. We appreciate the guidance and opinions of Jingyuan Liao and Junwen Wang.
Notes and references
- C. A. Falciola and A. Alexakis, Copper-Catalyzed Asymmetric Allylic Alkylation, Eur. J. Org. Chem., 2008, 2008, 3765–3780 CrossRef.
- L. Susse and B. M. Stoltz, Enantioselective Formation of Quaternary Centers by Allylic Alkylation with First-Row Transition-Metal Catalysts, Chem. Rev., 2021, 121, 4084–4099 CrossRef CAS PubMed.
- T. Fan, J. Song and L. Z. Gong, Asymmetric Redox Allylic Alkylation to Access 3,3'-Disubstituted Oxindoles Enabled by Ni/NHC Cooperative Catalysis, Angew. Chem., Int. Ed., 2022, 61, e202201678 CAS.
- X. J. Liu, W. Y. Zhang, C. Zheng and S. L. You, Iridium-Catalyzed Asymmetric Allylic Substitution of Methyl Azaarenes, Angew. Chem., Int. Ed., 2022, 61, e202200164 CAS.
- K. Tissot-Croset, D. Polet and A. Alexakis, A highly effective phosphoramidite ligand for asymmetric allylic substitution, Angew. Chem., Int. Ed., 2004, 43, 2426–2428 CrossRef CAS PubMed.
- M. Perez, M. Fananas-Mastral, P. H. Bos, A. Rudolph, S. R. Harutyunyan and B. L. Feringa, Catalytic asymmetric carbon-carbon bond formation via allylic alkylations with organolithium compounds, Nat. Chem., 2011, 3, 377–381 CrossRef CAS PubMed.
- M. Fananas-Mastral, M. Perez, P. H. Bos, A. Rudolph, S. R. Harutyunyan and B. L. Feringa, Enantioselective synthesis of tertiary and quaternary stereogenic centers: copper/phosphoramidite-catalyzed allylic alkylation with organolithium reagents, Angew. Chem., Int. Ed., 2012, 51, 1922–1925 CrossRef CAS PubMed.
- M. Perez, M. Fananas-Mastral, V. Hornillos, A. Rudolph, P. H. Bos, S. R. Harutyunyan and B. L. Feringa, Asymmetric allylic alkylation of acyclic allylic ethers with organolithium reagents, Chem.–Eur. J., 2012, 18, 11880–11883 CrossRef CAS PubMed.
- V. Hornillos, M. Perez, M. Fananas-Mastral and B. L. Feringa, Copper-catalyzed enantioselective allyl-allyl cross-coupling, J. Am. Chem. Soc., 2013, 135, 2140–2143 CrossRef CAS PubMed.
- W.-H. Deng, F. Ye, X.-F. Bai, L. Li, T. Song, Y.-L. Wei and L.-W. Xu, Chlorotrimethylsilane (TMSCl): an efficient silicon-based Lewis acid mediator in allylic alkylation using a diethylzinc reagent, RSC Adv., 2014, 4, 479–483 RSC.
- M. Fananas-Mastral, R. Vitale, M. Perez and B. L. Feringa, Enantioselective synthesis of all-carbon quaternary stereogenic centers via copper-catalyzed asymmetric allylic alkylation of (Z)-allyl bromides with organolithium reagents, Chem.–Eur. J., 2015, 21, 4209–4212 CrossRef CAS PubMed.
- S. Guduguntla, V. Hornillos, R. Tessier, M. Fananas-Mastral and B. L. Feringa, Chiral Diarylmethanes via Copper-Catalyzed Asymmetric Allylic Arylation with Organolithium Compounds, Org. Lett., 2016, 18, 252–255 CrossRef CAS PubMed.
- W. Xiong, G. Xu, X. Yu and W. Tang, P-Chiral Monophosphorus Ligands for Asymmetric Copper-Catalyzed Allylic Alkylation, Organometallics, 2019, 38, 4003–4013 CrossRef CAS.
- R. Tarrieu, A. Dumas, J. Thongpaen, T. Vives, T. Roisnel, V. Dorcet, C. Crévisy, O. Baslé and M. Mauduit, Readily Accessible Unsymmetrical Unsaturated 2,6-Diisopropylphenyl N-Heterocyclic Carbene Ligands. Applications in Enantioselective Catalysis, J. Org. Chem., 2017, 82, 1880–1887 CrossRef CAS PubMed.
- S. Guduguntla, J.-B. Gualtierotti, S. S. Goh and B. L. Feringa, Enantioselective Synthesis of Di- and Tri-Arylated All-Carbon Quaternary Stereocenters via Copper-Catalyzed Allylic Arylations with Organolithium Compounds, ACS Catal., 2016, 6, 6591–6595 CrossRef CAS.
- A. Alexakis and R. Millet, SimplePhos as Efficient Ligand for the Copper-Catalyzed Kinetic Resolution of Cyclic Vinyloxiranes with Grignard Reagents, Synlett, 2008, 2008, 1797–1800 CrossRef.
- S. Karabiyikoglu, A. V. Brethomé, T. Palacin, R. S. Paton and S. P. Fletcher, Enantiomerically enriched tetrahydropyridine allyl chlorides, Chem. Sci., 2020, 11, 4125–4130 RSC.
- H. You, E. Rideau, M. Sidera and S. P. Fletcher, Non-stabilized nucleophiles in Cu-catalysed dynamic kinetic asymmetric allylic alkylation, Nature, 2015, 517, 351–355 CrossRef CAS PubMed.
- E. Rideau, H. You, M. Sidera, T. D. W. Claridge and S. P. Fletcher, Mechanistic Studies on a Cu-Catalyzed Asymmetric Allylic Alkylation with Cyclic Racemic Starting Materials, J. Am. Chem. Soc., 2017, 139, 5614–5624 CrossRef CAS PubMed.
- X. Y. Cui, Y. Ge, S. M. Tan, H. Jiang, D. Tan, Y. Lu, R. Lee and C. H. Tan, (Guanidine)copper Complex-Catalyzed Enantioselective Dynamic Kinetic Allylic Alkynylation under Biphasic Condition, J. Am. Chem. Soc., 2018, 140, 8448–8455 CrossRef CAS PubMed.
- R. He, X. Huo, L. Zhao, F. Wang, L. Jiang, J. Liao and W. Zhang, Stereodivergent Pd/Cu Catalysis for the Dynamic Kinetic Asymmetric Transformation of Racemic Unsymmetrical 1,3-Disubstituted Allyl Acetates, J. Am. Chem. Soc., 2020, 142, 8097–8103 CrossRef CAS PubMed.
- V. Bhat, E. R. Welin, X. Guo and B. M. Stoltz, Advances in Stereoconvergent Catalysis from 2005 to 2015: Transition-Metal-Mediated Stereoablative Reactions, Dynamic Kinetic Resolutions, and Dynamic Kinetic Asymmetric Transformations, Chem. Rev., 2017, 117, 4528–4561 CrossRef CAS PubMed.
- J.-B. Langlois and A. Alexakis, Copper-Catalyzed Asymmetric Allylic Alkylation of Racemic Cyclic Substrates: Application of Dynamic Kinetic Asymmetric Transformation (DYKAT), Adv. Synth. Catal., 2010, 352, 447–457 CrossRef CAS.
- J. B. Langlois and A. Alexakis, Dynamic kinetic asymmetric transformation in copper catalyzed allylic alkylation, Chem. Commun., 2009, 3868–3870, 10.1039/b907722g.
- J.-B. Langlois, D. Emery, J. Mareda and A. Alexakis, Mechanistic identification and improvement of a direct enantioconvergent transformation in copper-catalyzed asymmetric allylic alkylation, Chem. Sci., 2012, 3, 1062–1069 RSC.
- J. Li, X. Song, F. Wu, H. You and F.-E. Chen, Cu-Catalyzed Asymmetric Allylic Alkylation of Racemic Cyclic Allyl Bromides with Organolithium Compounds, Eur. J. Org. Chem., 2022, 2022, e202200860 CAS.
- Y. Ge, X. Y. Cui, S. M. Tan, H. Jiang, J. Ren, N. Lee, R. Lee and C. H. Tan, Guanidine-Copper Complex Catalyzed Allylic Borylation for the Enantioconvergent Synthesis of Tertiary Cyclic Allylboronates, Angew. Chem., Int. Ed., 2019, 58, 2382–2386 CrossRef CAS PubMed.
- B. M. Trost and R. C. Bunt, Asymmetric induction in allylic alkylations of 3-(acyloxy)cycloalkenes, J. Am. Chem. Soc., 1994, 116, 4089–4090 CrossRef CAS.
- J. B. Langlois and A. Alexakis, Identification of a valuable kinetic process in copper-catalyzed asymmetric allylic alkylation, Angew. Chem., Int. Ed., 2011, 50, 1877–1881 CrossRef CAS PubMed.
- M. Sidera and S. P. Fletcher, Cu-catalyzed asymmetric addition of sp(2)-hybridized zirconium nucleophiles to racemic allyl bromides, Chem. Commun., 2015, 51, 5044–5047 RSC.
- N. A. Butt and W. Zhang, Transition metal-catalyzed allylic substitution reactions with unactivated allylic substrates, Chem. Soc. Rev., 2015, 44, 7929–7967 RSC.
- N. Suzuki, D. Y. Kondakov and T. Takahashi, Zirconium-catalyzed highly regioselective carbon-carbon bond formation reactions, J. Am. Chem. Soc., 1993, 115, 8485–8486 CrossRef CAS.
- H. Yasui, K. Mizutani, H. Yorimitsu and K. Oshima, Cobalt-and rhodium-catalyzed cross-coupling reaction of allylic ethers and halides with organometallic reagents, Tetrahedron, 2006, 62, 1410–1415 CrossRef CAS.
- H. F. Tu, C. Zheng, R. Q. Xu, X. J. Liu and S. L. You, Iridium-Catalyzed Intermolecular Asymmetric Dearomatization of beta-Naphthols with Allyl Alcohols or Allyl Ethers, Angew. Chem., Int. Ed., 2017, 56, 3237–3241 CrossRef CAS PubMed.
- G. Consiglio, O. Piccolo, L. Roncetti and F. Morandini, Asymmetric nickel-catalyzed cross-coupling reaction of allylic substrates with grignard reagents, Tetrahedron, 1986, 42, 2043–2053 CrossRef CAS.
- S. Tominaga, Y. Oi, T. Kato, D. K. An and S. Okamoto, γ-Selective allylic substitution reaction with Grignard reagents catalyzed by copper N-heterocyclic carbene complexes and its application to enantioselective synthesis, Tetrahedron Lett., 2004, 45, 5585–5588 CrossRef CAS.
- G. Laudadio, Y. Deng, K. van der Wal, D. Ravelli, M. Nuño, M. Fagnoni, D. Guthrie, Y. Sun and T. Noël, C (sp3)–H functionalizations of light hydrocarbons using decatungstate photocatalysis in flow, Science, 2020, 369, 92–96 CrossRef CAS PubMed.
- Y. Saito and S. Kobayashi, Chiral Heterogeneous Scandium Lewis Acid Catalysts for Continuous-Flow Enantioselective Friedel–Crafts Carbon–Carbon Bond-Forming Reactions, Angew. Chem., Int. Ed., 2021, 60, 26566–26570 CrossRef CAS PubMed.
- J. Wang, J. Li, Y. Wang, S. He, H. You and F.-E. Chen, Polymer-Supported Chiral Heterogeneous Copper Catalyst for Asymmetric Conjugate Addition of Ketones and Imines under Batch and Flow, ACS Catal., 2022, 12, 9629–9637 CrossRef CAS.
- J. Liao, S. Zhang, Z. Wang, X. Song, D. Zhang, R. Kumar, J. Jin, P. Ren, H. You and F.-E. Chen, Transition-metal catalyzed asymmetric reactions under continuous flow from 2015 to early 2020, Green Synth. Catal., 2020, 1, 121–133 CrossRef.
- Y. Wu, W. Q. Chen, Y. Q. Zhao and H. R. Piao, Efficient synthesis of panaxadiol derivatives using continuous-flow microreactor and evaluation of anti-tumor activity, Chin. Chem. Lett., 2015, 26, 334–338 CrossRef CAS.
- Y. Xin, S. Peng, J. X. Chen, Z. J. Yang and J. Y. Zhang, Continuous flow synthesis of porous materials, Chin. Chem. Lett., 2020, 31, 1448–1461 CrossRef CAS.
- L. Wan, G. Kong, M. Liu, M. Jiang, D. Cheng and F. Chen, Flow chemistry in the multi-step synthesis of natural products, Green Synth. Catal., 2022, 3, 243–258 CrossRef.
- Y. Yamamoto, S. Yamamoto, H. Yatagai and K. Maruyama, Lewis acid mediated reactions of organocopper reagents. A remarkably enhanced regioselective gamma-attack of allylic halides and direct alkylation of allylic alcohols via RCu·BF3 system, J. Am. Chem. Soc., 1980, 102, 2318–2325 CrossRef CAS.
|
This journal is © The Royal Society of Chemistry 2023 |